Open Access
ARTICLE
Investigating the Effects of Eichhornia Crassipes Biodiesel and Liquefied Petroleum Gas on the Performance and Emissions of a Dual-Fuel Engine
Mechanical Engineering Department, University of Technology-Iraq, Bagdad, Iraq
* Corresponding Author: Hawraa S. Mohammed. Email:
(This article belongs to the Special Issue: Recent advancements in thermal fluid flow applications)
Fluid Dynamics & Materials Processing 2023, 19(9), 2329-2343. https://doi.org/10.32604/fdmp.2023.026890
Received 01 October 2022; Accepted 14 December 2022; Issue published 16 May 2023
Abstract
This study considers the effect of Eichhornia Crassipes Biodiesel (ECB) blends on the performances, combustion, and emission characteristics of a direct injection compression ignition engine operated in a dual-fuel mode (DFM) and equipped with an Exhaust gas recirculation technique (EGR). In particular, a single-cylinder, four-stroke, water-cooled diesel engine was utilized and four modes of fuel operation were considered: mode I, the engine operated with an ordinary diesel fuel; mode II, the engine operated with the addition of 2.4 L/min of liquefied petroleum gas (LPG) and 20% EGR; mode III, 20% ECB with 2.4 L/min LPG and 20% EGR; mode IV, 40% ECB with 2.4 L/min LPG and 20% EGR. The operation conditions were constant engine speed (1500 rpm), variation of load (25%, 50%, 75%, and 100%), full load, with a compression ratio of 18, and a time injection of 23° BTDC (Before top died center). With regard to engine emissions, carbon dioxide (CO2), carbon monoxide (CO), hydrocarbons (UHC), and nitrogen oxide (NOX) were measured using a gas analyzer. The smoke opacity was measured using an OPABOX smoke meter. By comparing the results related to the different modes with mode I at full load, the BTE (Brake thermal efficiency) increased by 20.17%, 11.45%, and 12.66% with modes II, III, and IV, respectively. In comparison to the results for mode II, the BTE decreased due to the combustion of ECB blends by 7.26% and 6.24% for mode III and mode IV, respectively, at full load. In comparison to mode II, the Brake specific energy consumption (BSEC) increased with the ECB substitution. With ECB blends, there is a noticeable decrease in the CO, CO2, and UHC emissions at a partial load. Furthermore, the 20% ECB has no effect on CO emissions at full load. For modes II and IV, the CO2 increased by 33.33% and 19%, respectively, while the UHC emissions were reduced by 14.49% for mode III and 26.08% for mode IV. The smoke of mode III was lower by 7.21%, but for mode IV, it was higher by 12.37%. In addition, with mode III and mode IV, the NOx emissions increased by 30.50% and 18.80%, respectively.Keywords
Nomenclature
a TDC | After top dead center |
ASTM | American Society for Testing and Materials |
BSEC | Brake specific energy consumption |
BTE | Brake thermal efficiency |
BTDC | Before top died center |
DI | Direct Injection |
DFM | Dual fuel mode |
EC | Eichhornia Crassipes plant |
ECB | Eichhornia Crassipes Biodiesel |
20% ECB | 20% Eichhornia Crassipes Biodiesel + 80% IDF |
40% ECB | 40% Eichhornia Crassipes Biodiesel + 60% IDF |
EGR | Exhaust gas recirculation |
LPG | Liquefied petroleum gas |
ppm | Part of million |
IDF | Iraqi diesel fuel |
In the 20th century, the utilization of energy in the whole world has inevitably increased due to rapid demand in sectors of industrialization and transportation [1]. This expansion has resulted in the depletion of fossil fuel reserves [2]. Such concerns encourage scientists to search for new and renewable sources of energy that are ecofriendly [3]. Oil shortages and awareness of fossil-based engines’ environmental impact have made sustainable energy sources more attractive, such as biodiesel and LPG, which can be blended with other hydrocarbon fuels or used directly [4]. Many studies have been carried out with different rates of EGR technique and how they affect the performance, combustion and emission characteristics in a CI diesel engine with biodiesel blends.
The benefits of utilizing biodiesel instead of diesel fuel are numerous; biodiesel is nontoxic and biodegradable as well as its capability to reduce smoke emissions [1,5–7]. One of attractive biomass source for the biofuel industry is the Eichhornia Crassipes (water hyacinth) plant [8]. It is considered one of the fastest and most widespread colonial plants [9]. As a result of this plant’s excessive consumption of oxygen and water, it negatively impacts fresh water, aquatic life, irrigation of agricultural crops, and waterways. Recently, these plants have been growing bountifully in the Tigris and Euphrates rivers, which hinders the flow of water in those rivers and their tributaries in Wasit, Dhi Qar and Maysan.
A group of researchers [9,10] studied biodiesel blended from Eichhornia Crassipes and mixed with pure diesel at various ratios (10%, 20%, 30%, 40%, and 100% vol.) using Kirloskar, a single-cylinder, four-stroke, direct injection, and air-cooled diesel engine. They found that the engine’s thermal efficiency increased while UHC and CO emissions decreased from the combustion of EC blends. Besides, the additional biodiesel results in slightly higher NOX and CO2 emissions. Abdul Wahhab et al. [11] examined the effects of blending 10%, 20%, and 40% Eichhornia Crassipes with pure diesel. Under varied operating conditions, the performance of the IC engine powered by pure diesel and biodiesel was improved. The engine test revealed that biodiesel blends increased BTE when compared to pure diesel and a significant reduction in the exhaust emissions of CO and UHC with an increase in NOx.
The potential benefits of utilizing LPG in diesel engines are both economical and environmentally benign [12]. It significantly reduces CO and CO2 pollution, particulate matter, and smoke opacity when compared to diesel engines [13–15]. Ergenç et al. [16] conducted experimental research on the use of LPG in diesel engines. They tested different LPG ratios (10%, 20%, and 25%) in the diesel. The use of 25% LPG ratio results in the maximum improvements in engine power, torque, and BSFC. In terms of exhaust emissions, all LPG ratios reduce NOx and UHC emissions while increasing CO and CO2. In a direct-injection, single-cylinder, water-cooled diesel engine, Yuvaraj et al. [17] investigated diesel and LPG fuels. The experiment’s findings revealed a reduction in CO, CO2, UHC, and NOx emissions when comparing with LPG and diesel modes; the BTE improved by more than 3% with increased load.
Mahla et al. [18] examined the use of the EGR technique in a diesel engine powered by compressed natural gas (CNG), using a Jatropha biodiesel (B20) blend as a pilot fuel. Experiments were carried out at Kirloskar; with single-cylinder, direct injection, compression ignition diesel engine modified to operate in dual fuel mode with EGR. According to the results, dual fuel mode with EGR reduced BTE, NOx, and smoke emissions at all load conditions. while causing an increase in CO and UHC emissions. Elkelawy et al. [19] tested a direct injection, single-cylinder, four-stroke modified diesel engine with a peak power of 5 kW, constant speed of 1500 rpm, and various loads. Operating in a dual fuel mode with biodiesel as a pilot fuel and LPG as the primary fuel. EGR at varying ratios (10%–30%) was supplied into the engine via the intake mixing chamber. Under medium and high engine loads, raising EGR results in a modest increase in BTE with reduced CO, UHC, and NOx emissions. Increased biodiesel use reduces BTE and NOx emissions while increasing BSEC, CO, and UHC emissions. Kathirvel et al. [20] investigated 10% (N10) and 20% (N20) Neem oil methyl ester (NME) blends with 5% and 10% EGR. At full load, BTE for N20 was found to be 7.2% higher than for diesel, while BSEC was 11.4% lower. Meanwhile, the addition of EGR degrades the N20 blend’s performance parameters. The emission analysis revealed that the NOx value increased with biodiesel, whereas the addition of EGR decreased the NOx value for both biodiesel blends at all loading conditions.
Accordingly, it is evident from the studies in the previous literature that the dual fuel mode for diesel engines with EGR technology is successful and improves the engine’s performance and emissions. Therefore, the aim of the present investigation is to study the effects of using LPG (2.4 L/min), ECB (20%–40%) as a pilot fuel, and 20% EGR on the performance and exhaust emission of a single-cylinder, Kirloskar-VCR diesel engine at various load conditions (25%–50%–75%–100%) and constant speed (1500 rpm).
Experimental map is a number of tests were that being carried out for experimental work. All fuels experimental tests maps are listed in Table 1
2.2 Production of Eichhornia Crassipes (Water Hyacinth) Biodiesel
In the beginning, the Eichhornia Crassipes plant is collected from rivers and bodies of water by a special harvesting machine, then the parts of the plant were separated from each other (leaves, petioles, and roots). The roots are removed because they absorb heavy metals, then the leaves and petioles are manually washed with tap water and distilled water. After that, it is dried in the oven so it can be ground. After the sample was powdered, it was pre-treated with 1% NaOH for 2 h. A total of 10 kg of Eichhornia Crassipes was treated with a 6% sulfuric acid catalyst and a methanol-to-oil ratio of 5:1 in a hydrodynamic cavitation reactor at 65°C with circulating liquid glycerol for 45 min. After some time, the mixture is divided into two layers: the upper layer is the liquid fatty acid (biodiesel) [21], and the lower layer is the glycerin collected at the bottom of the container. There are residues of methanol, sodium hydroxide, soap, and glycerin in biodiesel. By adding distilled water to the biodiesel and shaking it well for about a minute, this residue can be removed. After that, the mixture is re-separated into two layers of biodiesel and water. The washing process was repeated several times, using fresh water each time to ensure the highest purity of the fuel, as previously recommended. A 50-liter hydrodynamic cavitation reactor was used to produce Eichhornia Crassipes oil biodiesel. The reactor consists of a double-lever glass and diaphragm pump and an air compressor to drive the double diaphragm pump, which acts as a power dissipation device in the hydrodynamic cavitation reactor. All processing sequences are mentioned in Fig. 1.
Figure 1: The schematic of preparing the biodiesel fuel
The fuels that were used in this investigation are as follows:
• Iraqi diesel fuel with the molecular formula (C12.3H22.2) [22].
• The biodiesel was extracted from Eichhornia Crassipes oil (ECB). As pilot fuel, 20% ECB (20% ECB-80% IDF) and 40% ECB (40% ECB-60% IDF) by mass was used.
• Liquified petroleum gas (LPG) contains a mixture of gases including propane (0.3C3H8), butane (0.6C4H10), and ethane (0.06C2H6). LPG is used at a constant flow rate of 2.4 L/min. Table 2 lists the physical properties of the utilized fuels.
Experiments were conducted on a VCR diesel engine, as shown in Fig. 2. The main specifications of the engine are shown in Table 3. A water-cooled eddy current dynamometer was used in this study to measure the torque generated by the engine. A box with an orifice system was used to measure the air flow rate. The intake air manifold was equipped with a gas carburetor for controlling the flow of LPG and mixing it with the air entering the engine. The amount of LPG was determined by using an LPG flowmeter. The EGMA-CG-450, a multi-gas emissions analyzer was used to measure the concentrations of CO, CO2, NOx, and UHC. The smoke was measured and displayed using a TEXA-OPABOX (AUTOPOWER) smoke meter model. All experiments were carried out in the laboratories of the AL-Musayyib Engineering Technology College-University of Babylon-Babylon-Iraq.
Figure 2: The experimental rig
2.4.2 Exhaust Gas Recirculation System
There are two EGR system supplementation methods employed in diesel engines, which are classified into hot and cold. In this investigation, a cold EGR system was used with a different rate is continuously inducted into the air intake manifold. The setup used in this work is depicted in Fig. 3. It includes EGR copper and steel pipes, a surge tank, a water inlet port, a water flow meter, a rotameter, thermocouple arrangements, control valves, and an EGR flow line. Water pumped inside the surge tank and extended to a copper hollow cylindrical pipe cooled the exhaust gases, resulting in a higher rate of heat transfer due to the temperature difference between the inside and outside (i.e., the water in the surge tank and the gas in the secondary manifold). The rotameter was used to regulate the amount of return gas. The exhaust gases collected at the top of the surge tank seem to be 28°C–30°C, indicating effective reduction. An additional stomach containing silica gel was added to ensure the system’s effectiveness and water removal from gases, it is well known that this substance has powerful water absorption properties. The EGR ratio is the proportion of EGR to the amount of charge aspirated into a cylinder in an engine. In this study, the EGR ratio was determined at 20% by weight.
Figure 3: Exhaust gas recirculated system
In this study, four modes of fuel (IDF, 2.4 L/min LPG, 20% ECB, and 40% ECB) were tested. They were started on mode I (IDF) and warmed up to 1500 rpm under various loads. After that, parameters such as operation speed and load were measured. In mode II, the engine was started with a pilot fuel in dual fuel mode of operation, (2.4 L/min of LPG were added to the engine by the carburetor in the intake air manifold, The LPG flow rate was adjusted at 2.4 L/min to ensure smooth engine operation and the absence of knock, and after a steady state, 20% EGR was added to the engine intake air manifold. Fuel consumption and temperatures were measured using an instrument of measurement. Smoke opacity and exhaust emissions were measured with an exhaust gas analyzer and a smoke meter. The same testing procedure was followed for the ECB blends in mode III and mode IV. For more accuracy, each reading was repeated three times to obtain the mean value.
1. Mass flow rate of fuel (kg/h)
2. Brake specific energy consumption (kg/kW.h)
3. Brake power (kW)
4. Brake thermal efficiency (%)
5. Mass flow rate of air (kg/h)
6. EGR percentage
Errors and uncertainty in the investigations are caused by the apparatus choice, conditions, calibration, configuration, observations, measurement, and standardized testing. Errors can occur during any study, irrespective of how precisely it is conducted. Estimation is required to confirm the accuracy of the tests. From the main measures, the outcome is obtained in an experiment. The following general equations are used for calculating uncertainty:
Diesel Engine Efficiency Measurements Uncertainties:
A- Brake power (BP):
Uncertainty in the brake power is given via:
Relative error = Error/Exact value
Uncertainty for the B.P is ±0.09.
B- Brake specific energy consumption (BSEC):
The error in Brake specific energy consumption is given by:
Relative error = Error/Exact value
Uncertainty for the BSEC is ±0.09.
C- Brake thermal efficiency (ηBTE):
Relative error = error/exact value
Uncertainty for the ηB.T.E is ±0.09.
Measurements with a Gas Analyzer Uncertainties:
Table 4 lists the percentage of uncertanity for the gaseous emissions that measured by exhust gas analyzer according to the manufacturing company (EGMA Garage Equipment, Korea).
2.5.1 Brake Thermal Efficiency (BTE)
Under various loads, Fig. 4 shows the influence of ECB blends 20% ECB, and 40% ECB as pilot fuel to the DFM with 20% EGR on BTE. In modes II, III, and IV, the BTE improved by 20.17%, 11.45%, and 12.66%, respectively, compared to mode I. By creating a homogeneous mixture inside the combustion chamber, LPG reduces the fuel ignition delay and promotes a more thorough combustion process. In addition, high EGR percentages result in a greater admission of active radicals and unburned hydrocarbons into the cylinder. As a result of the partially-cooled EGR, the intake charge was preheated, enhancing combustion conditions. For mode III and mode IV, the BTE decreases by 7.26% and 6.24% respectively with ECB blends at full load compared to mode II. Due to the ECB’s higher viscosity, greater volatility, and lower calorific value, BTE has decreased. As a result of 40% ECB’s higher oxygen content, BTE was significantly higher than 20% ECB. This result is agrees with previous studies [23,24].
Figure 4: The variation of brake thermal efficiency with variation of load
2.5.2 Brake Specific Energy Consumption
The BSEC is an important parameter of an engine because it takes care of both the mass flow rate and heating value of the fuel [25]. The effect of DFM with 20% EGR with different blends of ECB on BSEC for various loads is presented in Fig. 5. There is a clear reduction in BSEC at mode II by 19.48% in comparison with mode I, owing to the high temperature, homogeneous LPG and air mixture, a high calorific value of LPG, and unburned fuel participates in the combustion process. In comparison to mode II, the BSEC increased with the ECB substitution; and adding EGR contributing in reducing the oxygen concentration of fresh air into the combustion chamber. The mixing time between the direct-injected fuel and the fresh oxygen increased. The, low heating value of ECB reduced the burning rate upon the start of the diffusion combustion, thus making the stable combustion more difficult to achieve and indicating a BSEC increase. In comparison to mode II, the ECB blends showed the fowling, and at the partial loads, the increase in the BSEC was 17.94% and 14.10% for mode III and mode IV, respectively the BSEC of mode IV was lower than mode III by 3.26%. At full load, the increase was 8.82% and 5.88% for mode III and mode IV, respectively. The BSEC of mode IV is lower than mode III by 0.8%. The current results are in agreement with [26–28].
Figure 5: The variation of brake specific energy consumption with a variation of load
2.5.3 Carbon Monoxide Emission (CO)
Fig. 6 depicts the CO emissions of a DFM with 20% EGR and variable ECB substitution. Due to the lower combustion temperature and lack of oxygen at partial (low) load, CO emissions increased at mode II compared to mode I. With additional loading, at full load, the LPG and EGR contribute to a 77.7% reduction in CO emissions. This could be due to the enhancement effect of LPG in the combustion strategy. EGR allows for the re-combustion of a portion of the unburned hydrocarbons, increasing the possibility of complete combustion. Also, the active radicals improved the combustion conditions which are present in EGR. At a partial (low) load, ECB blends reduce CO emissions by 12.5% when compared to IDF as a pilot fuel (mode II). At full load, there is no change in CO emissions for mode III, but the change at mode IV is lower than mode III and mode II by 16.66%. These results are in agreement with [20,27,29].
Figure 6: The variation of CO emission with variation of load
2.5.4 Carbon Dioxide Emissions (CO2)
CO2 emissions increased with load in general due to improvements in the combustion process. Fig. 7 depicts the CO2 emissions of a DFM with 20% EGR at various ECB substitution and loads. At full load, CO2 emissions were reduced by 44.4% with mode II compared with mode I. This is due to the fact that LPG has a lower carbon-to-hydrogen ratio than diesel. In comparison to mode II, at partial (low) load, ECB reduced CO2 by 20%, 27% for mode III and mode IV, respectively. However, at partial (high) load, ECB increases CO2 by 33.33% and 19% for mode III and IV, respectively. This result agrees with [27,30,31].
Figure 7: The variation of CO2 emission with variation of load
2.5.5 Unburned Hydrocarbons (UHC)
The UHC emissions are composed of unburned or partially burned fuel [32]. Fig. 8 depicts the UHC emissions of a DFM with 20% EGR at various ECB substitutions and loads. The LPG and EGR at mode II increased the UHC emissions. Because the quantity of pilot fuel is small at partial (low) loads, the flame cannot propagate quickly and far enough to ignite the entire mixture, and some fuel escapes into the exhaust without being burned. Furthermore, the reduction in fresh air caused by LPG and EGR substitution results in incomplete combustion with a rich mixture. At high load, the quantity of a pilot fuel increased, which effectively burnt the surrounding fuel-air mixture and reduced the UHC emission. Because of the oxygen content in the biodiesel blend, which helps to promote better combustion, there is a clear reduction in UHC emissions with ECB blends at all loads. At partial (low) load, the UHC emission of mode IV is 12% higher than that of mode III. At full load, UHC emissions decreased as ECB percentage in the fuel increased, with reductions of 14.49% and 26.08% for mode III and mode IV, respectively, in comparison to mode II. This result is agree [18,27,30,33].
Figure 8: The variation of UHC emission with variation of load
2.5.6 Oxidizes of Nitrogen (NOx)
Fig. 9 shows the NOx emission of DFM with 20% EGR for various ECB substitutions and loads. It can be observed that there is a reduction in NOx emissions with EGR substitution at all loads, because the use of EGR is an efficient strategy in the dual fuel engine for decreasing NOx emissions for all loading conditions. Diluting the mixture and increasing its heat capacity, EGR replaces some of the O2 with CO2 and a small amount of H2O. As a result of the decreased oxygen concentration, the combustion temperature drops. When compared to mode I, the reduction in NOx emissions with mode II is 23.07% at full load. Additionally, with the ECB, it is evident that NOx emissions are rising, due to high oxygen content of ECB. At full load, NOx emissions increase by 30.50% and 18.80% for mode III and mode IV, respectively in comparison to mode II. This result is in agreement with [27,31,34,35].
Figure 9: The variation of NOx emission with variation of load
The amount of smoke opacity emitted from a CI engine’s exhaust was measured for all tests. Fig. 10 illustrates the smoke opacity of DFM with 20% EGR at various ECB substitutions and loads. The opacity of the smoke increases with load due to an increase in the amount of fuel. In addition, when compared to mode I, smoke for mode II was reduced. This could be because LPG’s C/H ratio was lower than IDF’s [36]. At partial load, it can be seen that as ECB increased, smoke opacity increased as compared to mode II. However, at full load, the smoke of mode III was 7.21% lower than mode II’s, while mode IV’s smoke was 12.37 and 15.55% higher than mode II and mode III, respectively. This result agrees with [37,28,34,38].
Figure 10: The variation of smoke opacity with variation of load
Experimental work was carried out in the current study to investigate the effects of ECB blends on dual-fuel engines using the LPG and EGR techniques. The following can be deduced from the examination of the experimental work:
1. At maximum load, it can be obtained that the BTE falls with the ECB by 7.26% for mode III and 6.24% for mode IV, respectively. Additionally, the BTE of mode IV is a little bit greater than mode III.
2. With the ECB substitution, BSEC grew. At partial loads, the BSEC increased by 17.94% for mode III and 14.10% for mode IV, respectively.
3. Engine emission parameters showed that using ECB blends results in a 12.5% reduction in CO emissions when compared to mode II, at a partial (low) load. At full load, the CO emissions of mode III do not change. However, mode IV has a change that is 16.66% less than mode III and mode II.
4. For ECB, CO2 emissions are reduced by 20% and 27% for mode III and mode IV, respectively, at partial (low) load. However, under partial (high) load, ECB causes an increase in CO2 of 33.33% for mode III and 19% for mode IV, respectively.
5. Additionally, it is evident that ECB blends at all loads significantly decrease UHC emissions. The UHC emission of mode IV is 12% greater than that of mode III at a partial (low) load. As the engine was running at full capacity, the UHC emissions reduced as the ECB increased; the reduction of UHC was 14.49% for mode III and 26.08% for mode IV, respectively, when compared to mode II.
6. With an increase in ECB, smoke opacity increased. However, at full load, the smoke of mode III was less than mode II by 7.21%, while the smoke of mode IV was greater than both mode III and mode II by 12.37% and 15.55%, respectively.
Funding Statement: The authors received no specific funding for this study.
Conflicts of Interest: The authors declare that they have no conflicts of interest to report regarding the present study.
References
1. Elumalai, P. V., Krishna Moorthy, R., Parthasarathy, M., Samuel, O. D., Owamah, H. I. et al. (2022). Artificial neural networks model for predicting the behavior of different injection pressure characteristics powered by blend of biofuel-nano emulsion. Energy Science & Engineering, 10(7), 2367–2396. https://doi.org/10.1002/ese3.1144 [Google Scholar] [CrossRef]
2. Ramalingam, K., Perumal Venkatesan, E., Aabid, A., Baig, M. (2022). Assessment of CI engine performance and exhaust air quality outfitted with real-time emulsion fuel injection system. Sustainability, 14(9), 5313. https://doi.org/10.3390/su14095313 [Google Scholar] [CrossRef]
3. Dhahad, H. A., Hamadi, A. S., Ali, S. A. (2017). Effect of aluminum oxide nanoparticles fuel additives on the performance and emissions of diesel engine. Engineering and Technology Journal, 35(9), 956–960. https://doi.org/10.30684/etj.2017.139931 [Google Scholar] [CrossRef]
4. Mahmood, A. S., Qatta, H. I., Al-Nuzal, S., Abed, T. K. (2020). Characteristics of exhaust emissions for a diesel engine fuelled by corn oil biodiesel and blended with diesel fuel. Engineering and Technology Journal, 38(3A), 457–464. https://doi.org/10.30684/etj.v38i3A.446 [Google Scholar] [CrossRef]
5. Fayad, M. A., Chaichan, M. T., Dhahad, H. A. (2021). Engine performance and PM concentrations from the combustion of Iraqi sunflower oil biodiesel under variable diesel engine operating conditions. Journal of Physics: Conference Series, 1973(1), 012051. IOP Publishing. https://doi.org/10.1088/1742-6596/1973/1/012051 [Google Scholar] [CrossRef]
6. Paparao, J., Pandey, K. K., Murugan, S. (2021). Experimental studies on the effect of TBC piston in a dual-fueled diesel engine. Fuel, 306(15), 121700. https://doi.org/10.1016/j.fuel.2021.121700 [Google Scholar] [CrossRef]
7. Özener, O., Yüksek, L., Ergenç, A. T., Özkan, M. (2014). Effects of soybean biodiesel on a DI diesel engine performance, emission and combustion characteristics. Fuel, 115, 875–883. https://doi.org/10.1016/j.fuel.2012.10.081 [Google Scholar] [CrossRef]
8. Sharma, A. K., Sharma, V., Sharma, V., Sharma, J. K., Singh, R. (2020). Multifaceted potential of Eichhornia Crassipes (Water Hyacinth) ladened with numerous value aided and therapeutic properties. Plant Archives, 20(2), 2059–2065. [Google Scholar]
9. Venu, H., Venkataraman, D., Purushothaman, P., Vallapudi, D. R. (2019). Eichhornia Crassipes Biodiesel as a renewable green fuel for diesel engine applications: Performance, combustion, and emission characteristics. Environmental Science and Pollution Research, 26(18), 18084–18097. https://doi.org/10.1007/s11356-019-04939-z [Google Scholar] [PubMed] [CrossRef]
10. Alagu, K., Venu, H., Jayaraman, J., Raju, V. D., Subramani, L. et al. (2019). Novel water hyacinth biodiesel as a potential alternative fuel for existing unmodified diesel engine: Performance, combustion and emission characteristics. Energy, 179(1), 295–305. https://doi.org/10.1016/j.energy.2019.04.207 [Google Scholar] [CrossRef]
11. Abdul Wahhab, H. A., Al-Kayiem, H. H. (2021). Environmental risk mitigation by biodiesel blending from Eichhornia Crassipes: Performance and emission assessment. Sustainability, 13(15), 8274. https://doi.org/10.3390/su13158274 [Google Scholar] [CrossRef]
12. Sudhir, C. V., Desai, V., Suresh Kumar, Y., Mohanan, P. (2003). Studies on influence of injection timing and diesel replacement on lpg- diesel dual-fuel engine. Fall Technical Conference of the ASME Internal Combustion Engine Division, vol. 41618, pp. 161–166. Erie, Pennsylvania, USA. [Google Scholar]
13. Ganesan, N., Sahoo, B. B., Ekambaram, P., Elumalai, P. V., Samuel, O. D. et al. (2022). Experimental based comparative exergy analysis of a spark-ignition Honda GX270 Genset engine fueled with LPG and syngas. Energy Science & Engineering, 10(7), 2191–2204. https://doi.org/10.1002/ese3.1125 [Google Scholar] [CrossRef]
14. Paparao, J., Murugan, S. (2021). Oxy-hydrogen gas as an alternative fuel for heat and power generation applications–A review. International Journal of Hydrogen Energy, 46(76), 37705–37735. https://doi.org/10.1016/j.ijhydene.2021.09.069 [Google Scholar] [CrossRef]
15. Poonia, M. P., Bhardwaj, A., Jethoo, A. S., Pandel, U. (2011). Experimental investigations on engine performance and exhaust emissions in an LPG diesel dual fuel engine. International Journal of Environmental Science and Development, 2(6), 418–422. https://doi.org/10.7763/IJESD.2011.V2.162 [Google Scholar] [CrossRef]
16. Ergenç, A. T., Koca, D. Ö. (2014). PLC controlled single cylinder diesel-LPG engine. Fuel, 130, 273–278. https://doi.org/10.1016/j.fuel.2014.04.016 [Google Scholar] [CrossRef]
17. Yuvaraj, M., SenthamilSelvan, M., Louis, S. W., Kayalvizhi, V. (2018). Performance and emission characteristics of a diesel-LPG duel fuel in greeves engine. Journal of Automation and Automobile Engineering, 3(3), 18–24. https://doi.org/10.5281/zenodo.1475512 [Google Scholar] [CrossRef]
18. Mahla, S. K., Dhir, A., Gill, K. J., Cho, H. M., Lim, H. C. et al. (2018). Influence of EGR on the simultaneous reduction of NOx-smoke emissions trade-off under CNG-biodiesel dual fuel engine. Energy, 152(43), 303–312. https://doi.org/10.1016/j.energy.2018.03.072 [Google Scholar] [CrossRef]
19. Elkelawy, M., El Shenawy, E. A., Mohamed, S. A., Elarabi, M. M., Bastawissi, H. A. E. (2022). Impacts of using EGR and different DI-fuels on RCCI engine emissions, performance, and combustion characteristics. Energy Conversion and Management: X, 15(3), 100236. https://doi.org/10.1016/j.ecmx.2022.100236 [Google Scholar] [CrossRef]
20. Kathirvel, R., Palanimuthu, V. (2022). Assessment of diesel engine performance, combustion and emission characteristics with supplementation of neem oil methyl ester along with EGR. Nature Environment and Pollution Technology, 21(2), 851–866. https://doi.org/10.46488/NEPT.2022.v21i02.049 [Google Scholar] [CrossRef]
21. Alismaeel, Z. T., Al-Jadir, T. M., Albayati, T. M., Abbas, A. S., Doyle, A. M. (2022). Modification of FAU zeolite as an active heterogeneous catalyst for biodiesel production and theoretical considerations for kinetic modeling. Advanced Powder Technology, 33(7), 103646. https://doi.org/10.1016/j.apt.2022.103646 [Google Scholar] [CrossRef]
22. Jamal, L., Saleh, A. M. (2022). Study effect of exhaust gas recirculation upon emissions and performance by using european diesel and iraqi diesel. Engineering and Technology Journal, 40(7), 970–978. https://doi.org/10.30684/etj.2021.131273.1021 [Google Scholar] [CrossRef]
23. Tiwari, D. R., Sinha, G. P. (2014). Performance and emission study of LPG diesel dual fuel engine. International Journal of Engineering and Advanced Technology, 3(3), 198–203. [Google Scholar]
24. Pandhare, A. P., Zende, K. C., Joglekar, A. S., Bhave, S. C., Padalkar, A. S. (2011). Effect of EGR on the exhaust gas temperature and exhaust opacity in compression ignition engines using jatropha oil as fuel. In: Applied mechanics and materials, vol. 110–116, pp. 431–436. Switzerland: Trans Tech Publications, Ltd. https://doi.org/10.4028/www.scientific.net/amm.110-116.431 [Google Scholar] [CrossRef]
25. Gumus, M., Kasifoglu, S. (2010). Performance and emission evaluation of a compression ignition engine using a biodiesel (apricot seed kernel oil methyl ester) and its blends with diesel fuel. Biomass and Bioenergy, 34(1), 134–139. https://doi.org/10.1016/j.biombioe.2009.10.010 [Google Scholar] [CrossRef]
26. Choi, S. H., Oh, Y. T. (2014). Decrement of toxic emission from direct injection engine by using animal fat biodiesel and cooled-exhaust gas recirculation system. Journal of Renewable and Sustainable Energy, 6(4), 042011. https://doi.org/10.1063/1.4890827 [Google Scholar] [CrossRef]
27. Rosha, P., Bharj, R. S., Gill, K. J. (2014). Performance and emission characteristics of Diesel + LPG dual fuel engine with exhaust gas recirculation. International Journal of Science, Engineering and Technology Research (IJSETR), 3(10), 2570–2574. [Google Scholar]
28. Kumaraswamy, A., Prasad, B. D. (2012). Performance analysis of a dual fuel engine using LPG and diesel with EGR system. Procedia Engineering, 38, 2784–2792. https://doi.org/10.1016/j.proeng.2012.06.326 [Google Scholar] [CrossRef]
29. Srikanth, V. S., Ganesan, S., Reddy, K. V. K. (2020). Investigations on the impact of EGR with a modified combustion chamber for CI diesel engine using biodiesel. AIP Conference Proceedings, 2311, 020004. https://doi.org/10.1063/5.0034514 [Google Scholar] [CrossRef]
30. Kumaraswamy, A., Prasad, B. D. (2012). Performance analysis of a dual fuel engine using LPG and diesel with EGR system. Procedia Engineering, 38, 2784–2792. https://doi.org/10.1016/j.proeng.2012.06.326 [Google Scholar] [CrossRef]
31. Gaikwad, M., Jadhav, K., Kolekar, A. (2016). Experimental analysis of dual fuel compression ignition engine with exhaust gas recirculation. International Journal of Current Engineering and Technology, Special Issue-5, 480–486. http://inpressco.com/category/ijcet [Google Scholar]
32. Vedaraman, N., Puhan, S., Nagarajan, G., Velappan, K. C. (2011). Preparation of palm oil biodiesel and effect of various additives on NOx emission reduction in B20: An experimental study. International Journal of Green Energy, 8(3), 383–397. https://doi.org/10.1080/15435075.2011.557847 [Google Scholar] [CrossRef]
33. Fayad, M. A., Omran, S. H., Ali, M. H., Al Salihi, H. A. (2022). Emission characteristics and engine performance from castor oil methyl ester blends in diesel engine under various injection pressures. Journal of Advanced Research in Fluid Mechanics and Thermal Sciences, 94(1), 45–59. https://doi.org/10.37934/arfmts.94.1.4559 [Google Scholar] [CrossRef]
34. Pathak, S. K., Nayyar, A., Goel, V. (2021). Optimization of EGR effects on performance and emission parameters of a dual fuel (Diesel+CNG) CI engine: An experimental investigation. Fuel, 291(24), 120183. https://doi.org/10.1016/j.fuel.2021.120183 [Google Scholar] [CrossRef]
35. Pirouzpanah, V., Sarai, R. K. (2003). Reduction of emissions in an automotive direct injection diesel engine dual-fuelled with natural gas by using variable exhaust gas recirculation. Proceedings of the Institution of Mechanical Engineers, Part D: Journal of Automobile Engineering, 217(8), 719–725. https://doi.org/10.1243/09544070360692104 [Google Scholar] [CrossRef]
36. Al-Dawody, M. F., Idan Al-Chlaihawi, K. K., Al-Farhany, K. A. (2022). Numerical simulation of the effect of LPG blending on the characteristics of a diesel engine. Heat Transfer, 51(2), 1918–1938. https://doi.org/10.1002/htj.22381 [Google Scholar] [CrossRef]
37. Valery, C., Abbe, N. (2022). Experimental study on the effect of load and Air + Gas/fuel ratio on the performances, emissions and combustion characteristics of diesel-LPG fuelled single stationary CI engine. Research Square, 159(2), 1–24. https://doi.org/10.21203/rs.3.rs-1871551/v1 [Google Scholar] [CrossRef]
38. Rajan, K., Senthilkumar, K. R. (2009). Effect of exhaust gas recirculation (EGR) on the performance and emission characteristics of diesel engine with sunflower oil methyl ester. Jordan Journal of Mechanical and Industrial Engineering, 3(4), 306–311. [Google Scholar]
Cite This Article
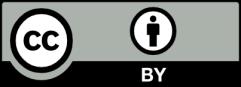