Open Access
REVIEW
Lateral Performance for Wood-Frame Shear Walls–A Critical Review
1
School of Civil Engineering, Nanjing Forestry University, Nanjing, 210037, China
2
Joint International Research Laboratory of Bio-Composite Building Materials and Structures, Nanjing Forestry University, Nanjing,
210037, China
3
University of Naples Federico II, Via Claudio 21, Naples, 80133, Italy
4
Department of Furniture, Design and Habitat Brno, Mendel University in Brno, Brno, 61300, Czech Republic
5
Department of Wood Processing, Faculty of Forestry and Wood Sciences, Czech University of Life Sciences Prague, Suchdol, 16521,
Czech Republic
* Corresponding Authors: Ottavia Corbi. Email: ; Haitao Li. Email:
Journal of Renewable Materials 2023, 11(5), 2143-2169. https://doi.org/10.32604/jrm.2023.026773
Received 25 September 2022; Accepted 04 November 2022; Issue published 13 February 2023
Abstract
Wood is a green material in line with the sustainable development strategy. From the excellent performance of engineering wood products, modern wood structures represented by light wood structures have gained more development opportunities. As an indispensable part of light wood structure systems, the wood-frame shear wall plays a vital role in the bearing capacity and earthquake resistance of light wood structure systems. This paper is focused on a review of the lateral performance of wood-frame shear walls and classifies the influencing factors in relevant experimental research into three categories, including internal factors such as shear wall structure, external factors such as test scheme, and other factors of material production and test process. Finally, the research prospects in this field were introduced based on the summary of the research status. This work can be a reference for further research on the lateral performance of wood-frame shear walls.Keywords
Since the concept of sustainable development is increasingly being advocated, and environmental protection awareness is highly enhanced [1–4], green building materials represented by wood have attracted extensive attention. Wood is a naturally growing, renewable and organic material whose mechanical properties, such as strength and elastic modulus, can meet the needs of structural use, so wood has been widely used in various structural systems. Nowadays, the world vigorously promotes prefabricated buildings and advocates the concepts of energy conservation, emission reduction and environmental protection [5], making modern wood structure buildings a major innovation in the building materials industry. Engineering wood products not only overcomes the natural wood shortage but also greatly improves the strength and utilization of wood. It is a modern wood structure that has realized factory processing and assembly construction and developed towards large span, high-rise and super high-rise [6].
At present, modern wood structures, as one of the important forms of sustainable resource utilization and low-carbon building structures, are encouraged in many countries around the world [7,8], and the common structural forms are mainly light wood structures, glued wood structures and wood hybrid structures. Among them, the structural system of the light wood structure is mainly composed of wood-frame walls, wood floors, and wood roof systems. The system is nailed by specification materials and wood-based structural plates to bear and transfer various loads acting on the structure. In general, light wood structure buildings have the advantages of excellent seismic performance, good thermal insulation performance, high degree of prefabrication, fast and convenient construction, high material utilization rate and beautiful design [9].
As one of the three important components of the light wood structure system, the wood-frame shear wall is the most important lateral resistance member, which plays a vital role in resisting the horizontal effects of wind load and earthquake load for light wood structures [10,11]. The study of structural properties of wood-frame shear walls is the basis for the overall analysis of houses, and many scholars have researched the performance of wood-frame shear walls. Therefore, this paper presents a review of domestic and international experiments and research results on the lateral performance of wood-frame shear walls in the hope that it can provide a reference for future research and engineering applications.
As shown in Fig. 1, the wood-frame shear wall is composed of a wall column, wall panel, top beam plate, bottom beam plate and other units, and the connection between components is nailed. As a high-order statically indeterminate structural system, the bearing capacity of a wood-frame shear wall is obtained through the joint action of main structural members (wood frame) and secondary structural members (wall panel, floor panel, roof panel). The lateral force of the shear wall is mainly borne by the panel and then transmitted to the wood frame through the panel-wood frame nail joint. The wood-frame shear wall in the panel plane has high lateral stiffness, so it can withstand horizontal shear and reduce lateral deflection.
Figure 1: Wood-frame shear wall [12]
The lateral performance of the wall is an important factor in determining whether the building can be safe under the effect of earthquake hazards and is also a basic guarantee to strengthen and maintain the safety of the building. In modern wood buildings, the wall accounts for a large proportion, which is the key to building performance, structural safety, and architectural design and construction. Scholars around the world have found that the type and specification of wood-based structural panels, joint connection mode, wall column layout, and wall structure form in wood building walls are all important factors affecting seismic performance [13–16].
The study of the lateral performance of wood-frame shear walls is a top priority in the study of light wood structures. Therefore, many scholars have studied the lateral force resistance of wood-frame shear walls under different factors by conducting different tests and comparative analyses [17–20]. After reading the relevant literature, this paper classified them into the following three main categories of causes: intrinsic factors of shear wall structure, extrinsic factors of test scheme, and some other factors such as differences in the shear wall fabrication process and test procedure.
3 Intrinsic Factors of Shear Wall Structure
3.1 Effect of Panel Material on Wall Performance
It was the material, thickness, and size of the panel that had a significant impact on the mechanical properties of the shear wall, which would directly affect the nail joints and the damage degree of the wall [21,22]. Several scholars from China carried out comparative tests to verify the mechanical properties of the domestic board. He et al. [23] conducted tests on wood-frame shear walls of 2.44 m × 2.44 m covered with imported oriented strand board (OSB) panels, domestic OSB panels, domestic plywood panels, magnesium board, and gypsum board. As shown in Table 1, the shear walls with domestic plywood and OSB panels were better than that with imported OSB boards in terms of bearing capacity, ductility, and energy consumption. Magnesium board was not only of excellent fire and waterproof performance but also higher strength and toughness than gypsum board. In general, the OSB panel was still the most common and reliable choice for the construction of shear walls, while plywood could also meet the requirements of part buildings.
As shown in Table 2, Du et al. [24] tested three groups of the wood-frame wall, which used different panel materials with the same wall column material. When using the same wall column material, the shear strength of the wall with imported OSB board and domestic plywood board was relatively close, showing good shear performance, while the shear performance of the wall with douglas fir board was poor. Compared with the wall with OSB panel, the ultimate displacement of the wall with plywood was increased, while that of the wall with douglas fir board was greatly reduced, and the main reason for this was that the plywood had high strength, high toughness, and was easy to deform, on the contrary, the texture of the douglas fir board was relatively hard and brittle, which was prone to rupture. Overall, plywood could replace OSB for the fabrication of shear walls compared to traditional shear walls using OSB as panel material, while douglas fir was not suitable for that as a structural plate.
Gypsum wall boards (GWB) were widely used in light wood structures, which had a significant influence on the strength, stiffness, and ductility of those structures. Chen et al. [25] investigated the racking performance of shear walls with OSB and GWB under different panel orientations. Part of the parameters is listed in Table 3. It could be seen from Fig. 2 that the stiffness and strength of the shear wall with OSB or GWB in vertical panel orientation were higher than that in horizontal panel orientation. A full-scale X-type GWB sheathed shear wall test was conducted to further study the effect of the fastener type, spacing at panel edges, and panel edge distance [26].
Figure 2: Comparison of test results: (a) Stiffness; (b) Strength [25]
Zhu et al. [27] found that the stiffness of shear walls with GWB single-clad was significantly greater than that of OSB. Compared with the shear wall with OSB single-clad, the bearing capacity and stiffness of the shear wall with double-clad increased by 30.8% and 371.3%, respectively. Zhou [28] further studied the influence of GWB on wall performance under the premise that GWB could improve the lateral resistance of the light wood shear walls. It was found that when only one side was covered with GWB, the damage to the shear wall was more serious, and the panel was prone to large rotational deformation until failure. The results proved that the GWB could effectively improve the ultimate bearing capacity of the wall and can significantly increase the stiffness of the wall in the elastic stage. Therefore, the contribution of GWB should be taken into consideration during the design of wood-frame shear walls.
On this basis, many scholars proposed new panel materials based on the shortcomings of existing materials and also innovatively combined with reinforcement measures to effectively enhance the overall stability and lateral resistance of shear walls, achieving the expected results [29,30]. Li et al. [31] and Wang et al. [32] introduced a new light shear wall with ply-bamboo sheathing panels, which indicated that the mechanical properties of the new light shear wall were similar to those of the traditional light shear wall, except that the initial stiffness and peak load were higher, and the ductility was slightly worse.
Di et al. [33] tested the performance of light wood-frame shear walls with SPF, parallel bamboo strand lumber (PBSL), and OSB under two loading directions and compared the failure modes of the walls. As shown in Fig. 3, it was found that the stronger the mechanical properties of the panel, the greater the deformation of the panel nail at failure. Fig. 4 indicated that the use of PBSL and OSB for the panel not only enhanced the deformability but also improved the bearing capacity compared to the use of SPF. It was worth mentioning that the ultimate load of panel nails with PBSL for the panel under two directions was nearly doubled compared to SPF for the panel.
Figure 3: Failure modes of different panel-frame connections: (a) OSB (i) NAIL pulled out; (ii) Nail embedded; (b) SPF; (i) Nail bending; (ii) Panel tearing; (c) PBSL; (i) Nail pulled out; (ii) Nail breaking; (iii) Panel tearing [33]
Figure 4: Comparison of test results: (a) Ultimate displacement; (b) Ultimate load [33]
In brief, the above research tested and compared the properties of commonly used panel materials through experiments. OSB and SPF with good performance were selected as structural plates to construct traditional wood-frame shear walls. However, plywood, ply-bamboo, and engineered bamboo represented by PBSL had been demonstrated to have good mechanical properties, which provided a new research direction for modern wood structures. If the advantages of local fast-growing bamboo resources were exerted, it could completely replace wood and be used in light wood structure buildings.
3.2 Effect of Wall Column on Wall Performance
The existence of the wall column could significantly improve the lateral resistance of the shear wall, and the mechanical properties of the wall column material would directly affect the failure form of the nail joints and the wall. As shown in Table 4, Du et al. [24] set imported SPF and domestic fir as the wall column for the comparative test, and found that the shear performance of the wall with SPF columns was generally better than that of the wall with domestic fir columns, and also that the ultimate displacement was smaller when the damage occurred. Furthermore, compared with the wall made entirely of imported materials, the wall made of domestic fir and board plywood had obvious disadvantages in shear strength, stiffness, and ultimate displacement.
Zhou et al. [34] studied in depth the relationship between the maximum bearing capacity and the load displacement of the wood skeleton in the shear wall when acting alone. Firstly, the bending properties of the nails were tested by the skeleton nail test, which verified that the material properties of the nails met the specification requirements; secondly, the skeleton nail joint test was carried out to detect the bending, shear, and pullout mechanical properties of the nail joints, and the calculation formula of the nail node was derived. Then, the lateral resistance performance of the empty wood skeleton was tested, and finally, the calculation formula for the lateral resistance performance of the wood skeleton was derived. Therefore, the conclusion that the lateral resistance of wood skeleton was largely determined by the bending and pulling resistance of nail joints was proposed. Zhang et al. [35] investigated the influence of wall columns on lightweight composite wood shear walls with glass fiber-enhanced cladding and concluded that wall columns could significantly improve the bearing capacity and stiffness of wall panels. In addition, the peak bearing capacity of wall columns under tension was higher than that under compression.
As mentioned above, there was little research to study the effect of wall columns on the performance of shear walls alone. However, there is no doubt that the wall column, as an important component of shear walls, had a direct impact on the deformation of shear walls by their bearing capacity. It should be noted that in the design and construction of shear walls, it was necessary to avoid nailing the panels on both sides of the wall to the same column. When the beam was placed on the top of the wall column, there should be a composite column composed of several wall columns directly below the beam. Besides, setting double top beam plates in load-bearing walls was also an effective method to enhance the lateral performance of shear walls.
3.3 Effect of Nailing Joint on Wall Performance
In the standard wood-frame shear walls, the nail joints are the most dominant form of joints in wood-frame houses. According to the different nail connection members, the nail connection nodes can be divided into panel-wood frame nail joints and wood frame nail joints. Since Countryman first conducted the related nail joint test in 1952, domestic and foreign scholars have carried out research in this field for nearly 70 years. Zhu et al. [27] fully demonstrated that nail joints technology is the key factor directly determining the bearing capacity of shear walls, and many studies have shown that most of the damage to the shear wall was caused by the failure of nail joints between the wall column and bottom beam plate.
Chen et al. [36] tested 268 specimens with nail joints in wood shear walls and found 6 failure modes, among which the specimens with nail joints pulled out had the highest bearing capacity and the best ductility. The test also found that the strength of the nail joints was not affected by the angle of the wood grain but by the insufficient margin. Bagheri et al. [37] explored the influence of structural details on the performance of shear walls and proposed that the diameter and spacing of nails have significant effects on wall strength. Moreover, the reduction of nail spacing did not affect the bearing capacity of the wall, but it would have an obvious impact on the stiffness. In order to promote the application of bamboo materials in the construction industry. Li et al. [31] discussed that shear walls with ply-bamboo sheathing panels should be made with two different types of nails: spiral nails and nail staples. The bearing capacity of two shear walls was 5.2 and 6.2 kN/m, respectively, which could meet the design value of 3.5 kN/m for the bearing capacity of ordinary wood-based structure plate shear walls with the same thickness, therefore leading Wang et al. [32] to introduce a new shear wall made with a laminated bamboo (glubam) stud frame and ply-bamboo sheathing panels, the tests of such shear walls with three different panel-frame nailing connections were performed. And the results demonstrated that when the failure mode of the panel-frame connection was the yielding of the nails, increasing the diameter of the nail effectively improved the bearing capacity of the wall. If the failure mode was changed to the pull-through of nails from the ply-bamboo panels, increasing the diameter of the nail was no longer effective. Table 5 compares the test results of shear walls with different connections.
Based on clarifying the important influencing factors of panel nail joints on the lateral performance of wood-frame shear walls, many scholars have begun to innovate conventional panel nail joints. Dinehart et al. [38] inserted the viscoelastic polymer between the sheathing and the stud framing, proving that the new panel nail joints had higher stiffness and higher energy consumption capacity. Wang et al. [39] used high-strength, high-toughness glass fiber reinforced plastic (GFRP), pasted with epoxy resin on both sides of the node range, to make reinforced nail connections. It turned out that GFRP had an outstanding reinforcing effect on the nail connection, and with the increase in the number of bonding layers, the reinforcing effect became larger, but the reinforcing amplitude decreased. Yang et al. [40] tried to strengthen the wood members’ end with powdered carbon fibers and found that under monotonic loading, the joints shearing resistance of Fraxinus mandshurica and Larix olgensis wood members’ reinforcement end had been increased by 102.54% and 78.23%, respectively, which also effectively reduced the generation and expansion of end cracks. Xia et al. [41] proposed that rubber connection could improve the bearing capacity and displacement of joints by pasting rubber strips between the stud and panel. Different failure modes are shown in Fig. 5. Wang et al. [42,43] designed a new type of nail joint with a silica gel strip, whose bearing capacity was significantly higher than that of the conventional one. Furthermore, the new type of shear wall with nail joints had relatively less damage and lower maintenance costs.
Figure 5: Failure modes of nails: (a) Nail yielding; (b) Nail cap penetration; (c) Rubber tearing; (d) Rubber tearing and nail yielding [41]
In case the shear walls were subjected to an earthquake, the connection between the wall column and the wall column, the wall panel and the wall column, and the wall and the main body were the most critical. Scholars have conducted extensive research on the lateral performance of wood-frame shear walls by focusing on the types, diameters, lengths, spacing, and other parameters of nail joints. In addition, they have also innovated based on conventional nail joints and designed a variety of new panel nail joints with more characteristics, further enhancing the performance of the wall.
3.4 Effect of Opening on Wall Performance
As the use of wood-frame building products expanded, building geometric irregularities increased, and more and larger wall openings gave rise to further research into the lateral performance of wood-frame buildings, including the form and size of the openings. After comparing them with intact shear walls without openings, the effect of openings on the mechanical performance of shear walls was analyzed. Doudak et al. [44] established a finite element model for analyzing shear walls with openings and tested the accuracy of the model through full-scale shear wall tests. Both experiments and modeling in Table 6 demonstrated that openings above shear walls affected the stiffness and strength of the structure, but without a certain proportion.
Based on the test results, Liu et al. [45] concluded that the size of the opening had little effect on the shear strength of the wall and the uplift of the wall column, but the change in the size of the opening changed the slenderness ratio of the wall limbs, which in turn changed the deformation shape of the whole wall. As the slenderness ratio of the wall increased, the deformation of the wall tended to be “bending.” As shown in Fig. 6, Xue et al. [46,47] investigated the performance of glued-laminated timber (GLT) frame and shear walls infilled with cross-laminated timber (CLT), and also analyzed the collaborative working mechanism of GLT frame and CLT shear walls. The results in Table 7 showed that the lateral bearing capacity and stiffness of the GLT frame were improved after the addition of CLT shear walls. In addition, the opening of the CLT shear wall had a marked weakening effect on the lateral performance, and the greater the ratio of door openings and window openings, the greater the weakening effect.
Figure 6: Different forms of wood-frame shear walls: (a) TFB; (b) TFBC-1; (c) TFBC-2; (d) TFBC-3 [46]
Fu et al. [48] established an analysis model on two kinds of wood-frame shear walls and found that the mechanical performance and deformation capacity of the wall with a door opening were inferior to those of the wall without an opening. The model better simulated the real stress state and deformation characteristics of wood shear walls, providing a meaningful reference for theoretical analysis.
In general, the above research showed that the opening of a door and window in the wood shear wall panel would significantly weaken the bearing capacity of the structure, and the larger the opening rate, the more the bearing capacity was reduced. It was found that the shear wall with an opening was more prone to lateral instability and bending at the edge of the door under the action of lateral load and vertical pressure. Therefore, it could be strengthened by adding angle steel and self-tapping screws.
3.5 Effect of the Composite Frame on Wall Performance
As early as the beginning of the 20th century, there were no guidelines for the design of light-gauge steel-frame wood panel shear walls. However, with the application of this composite structure becoming more and more widespread, many scholars took the lead in the study. Branston et al. [49] put forward the design method of the steel-frame wood-panel shear wall and conducted an extensive testing program to build a database of shear wall information. Boudreault et al. [50] selected two representative buildings to establish a shear wall model, scaled a set of seismic records into the model, and determined the corresponding seismic force correction coefficient.
Chen et al. [51] predicted the strength and deflection of the light-gauge steel-frame wood panel shear walls based on the existing analysis methods and then compared the predicted values with the test results of full-size shear walls and found that the two results were consistent. Li et al. [52,53] investigated the lateral performance of the steel-wood hybrid shear wall system through experiments and discovered the complementary effect between the infill wood wall and the steel frame, which could reduce the inter-story displacement of the hybrid system. Later on, they continued to study the seismic performance of the multi-story steel-wood hybrid structure and established a nonlinear numerical model considering damage accumulation and stiffness degradation, whose accuracy was verified by test.
In 1998, Varoglu et al. [54] in Canada first proposed the concept of a midply wood shear wall system, in which the wall studs were symmetrically arranged on both sides of the wall panel. And then, the “wall stud-wall panel-wall stud” was fixed together to obtain a new type of wood shear wall with high lateral force resistance. The process is shown in Fig. 7. Hong et al. [55] and Varoglu et al. [56] conducted lateral force tests on the shear wall with the size of 2.4 m × 2.4 m, and discovered that the lateral bearing capacity of midply wood shear wall was three times higher than that of ordinary standard wood shear walls, and the lateral stiffness resistance was about two to three times higher. Zheng et al. [57,58] pointed out that increasing panel thickness and decreasing nail spacing contributed to the improvement of lateral stiffness and shear strength of plywood wood shear walls, while changes in stud spacing had little effect.
Figure 7: Midply wood shear wall [55]
Zheng et al. [59] also concluded in the test that the lateral performance of the wood frame-midply shear wall hybrid system depended mainly on the performance of the shear wall infilled with midply. Benefiting from the constraint and protection effect of the outer post-and-beam frame, the uplift of the end studs was effectively suppressed, and the ductility of the system was improved. Moreover, the ultimate bearing capacity and lateral stiffness of the wood frame-midply shear wall hybrid system were about twice that of the wood frame shear walls infilled with normal wood panels. Similar conclusions were also obtained in the experiment of Xue et al. [60].
He et al. [61] experimentally informed that the order of energy consumption capacity, ultimate load, and failure load was the hybrid structures, the light wood frame constructions, and the post-beam constructions successively. However, the stiffness of the hybrid structures was still far lower than that of the light-frame wood shear wall. Similar tests were carried out by Shim et al. [62] and Zheng et al. [63], and it was proved that the lateral bearing capacity of hybrid structures was significantly higher, which could be calculated as the arithmetic summation of post-beam structure and the light frame shear wall. In the research about glued-laminated wood post-beam structures, Xiong et al. [64,65] proposed that the stiffness in the elastic stage of the pure frame structure system is too small to be used as an anti-lateral force structure system alone, and designed four lateral strengthened structure systems, all of which showed good lateral stiffness.
It could be found from the above literature that scholars had proposed composite frame structures systems including steel-frame, midply shear wall, glued-laminated wood frame, etc., based on wood-frame shear walls. When the system was subjected to an external load, it not only gave the shear wall a certain protective effect but also played a synergistic role with the shear wall, so that the lateral bearing capacity of the composite structure systems was greatly improved compared with that of the single structure.
4 Extrinsic Factors of Test Scheme
4.1 Effect of Upper Load on Wall Performance
Taking the traditional wood-frame shear wall without corner anchors as the object, Cheng et al. [66] found the upper load could act as a “corner anchor” at the ends of the wall. When the upper load was applied to the shear wall, it was not only able to restrain the uplift of the studs at the end and both sides of the opening, but also to increase the shear strength (42%) and elastic lateral stiffness (36%) of the wall significantly. When it acted on the flange wall, it could also reduce the uplift of the studs at the end of the flange wall, but it had less effect on the structural performance such as lateral stiffness and ultimate displacement. The schematic diagram of different loading positions was dawned in Fig. 8.
Figure 8: Position of upper load: (a) Load on the shear wall; (b) Load on the flange wall [66]
Liu et al. [67] proposed the stiffness of the load spreader connected to the top beam plate had a direct impact on the performance of the wood-frame shear wall with different sizes of openings, as shown in Table 8. Compared to the specimen with a flexible load spreader in Fig. 9, adding a rigid load spreader can effectively improve the shear strength of the wood-frame shear walls. However, since the load spreader outside the specimen had no constraint effect on the shear wall specimens [68], the shear strength was smaller than that of other specimens with the same structure.
Figure 9: Comparing wood shear walls using three load spreaders: (a) Shear strength; (b) Lateral stiffness [67]
It was obvious that the upper load positively contributed to the lateral performance of the wood-frame shear wall. Whether by applying vertical loads and a rigid load spreader on the top beam plate of the shear wall or by adding a second storey, it could be understood that a downward force was given to the shear wall at the top, making the connection between the studs and the top and bottom beam plates stronger. This not only effectively inhibited the uplift of the studs after the wall was subjected to lateral load but also protected the nail connection between the studs and the bottom beam plate, thus improving the lateral performance of the structure.
4.2 Effect of Diagonal Brace on Wall Performance
The addition of diagonal bracing in the frame of the shear wall could make the frame form a stable triangular unit composite structure, which effectively restrained the deformation of the frame and enhance its integrity. As shown in Fig. 10, Xiong et al. [64] designed four lateral strengthened structure systems based on the fact that the stiffness of the pure frame structure system was too small to bear the external load. The results confirmed that all four systems showed good lateral resistance, Similar conclusions can be found in the study of Wadi et al. [69]. Moreover, he proposed that diagonal struts were more convenient for practical applications. However, the performance and structure of each system still needed to be further optimized. Du et al. [70] added a cross brace made of soft steel bars to the frame and acquired experimentally the maximum uplift of end studs was only 22.7% of that in the wall without a cross brace. The cross brace played a considerable role in improving the stiffness of the wall.
Figure 10: Lateral strengthened structure systems: (a) Frame with X-brace; (b) Frame with K-brace; (c) Frame with knee-brace; (d) Frame filled with light wood shear walls [64]
Hou [71] and Zuo et al. [72] investigated the prestressed diagonal crossbar reinforced wood shear walls in Fig. 11, and confirmed that prestressing effectively enhanced the elastic lateral stiffness and ductility of the walls but had little effect on the shear strength. Furthermore, they concluded that setting corner anchorage members and cross bars could markedly improve the joint strength of the walls and strengthen the integrity.
Figure 11: Different types of light wood-frame shear wall: (a) Light wood-frame shear wall; (b) With corner anchorage members; (c) With cross bars (prestress) [71]
Yang et al. [40] used wood-plastic composites (WPC) and wood structures as the main material to produce wood-frame shear walls integrated with wood-plastic composites (WPC). It was the WPC integration method that affected the lateral performance of the wall. Moreover, the walls with a diagonal brace were superior to ordinary conventional walls in lateral performance, including the ∧-type, K-type, and X-type brace.
The main test results of shear walls with a different diagonal brace were summarized in Table 9. In summary, the above research tested diagonal brace of different materials, shapes, and connection methods separately and confirmed the contribution of diagonal brace to the improvement of shear wall performance. It was also found in the comparative tests that the installation of corner anchorage members, crossbars, and prestressing can effectively enhance the integrity of shear walls and improve lateral resistance.
4.3 Effect of Friction Dampers on Wall Performance
With the need for industrialization and the use of more eco-friendly materials, it was a wood hybrid structure that attracted much attention [73,74]. At present, the main forms of wood hybrid structures include concrete-wood and steel-wood hybrid structures [75]. Tesfamariam et al. [76] proposed a novel hybrid structure consisting of a steel moment-resisting frame (SMRF) with CLT infill panels. Loss et al. [77,78] introduced a highly-industrialized steel–wood hybrid shear wall with steel frames, composite steel-wood floors, and shear walls with CLT sheets. He et al. [79–81] investigated the seismic performance of the steel-timber hybrid systems, where prefabricated light wood frame shear walls were infilled in a steel moment-resisting frame.
To achieve cooperation between the steel frame and wood shear wall, the most important thing was to ensure the reliability of the connection. Scholars [82–86] conducted studies on hybrid steel-wood connections and proposed different connection forms for different situations. He et al. [87] compared two different connection modes and proposed that both the stiffness and bearing capacity of the steel-wood hybrid wall with high-strength bolts were higher, while in the ultimate state, the deformability of the steel-wood hybrid wall with ordinary bolts was better.
Given this situation, some scholars tried to introduce slip-friction connectors originally developed for the steel frame industry into the steel-wood hybrid structure. Loo et al. [88] attempted to replace traditional hold-down connectors with slip-friction connectors, shown in Fig. 12, effectively limiting loads on the walls and providing ductility to the systems. It was evident that the hysteresis characteristics of lip-friction connectors enabled the wood shear walls to withstand greater seismic loads and avoid remarkable inelastic deformations.
Figure 12: Slip-friction connectors [88]
Pu et al. [89] experimentally found that the addition of dampers to wood structures could effectively protect the structure systems and reduce the damage of main structural members after load. Poh’sie et al. [90] applied a tuned mass damper (TMD) to a high-rise cross-laminated (X-Lam) wood building and tested the improvement effect of different TMD design parameters on the seismic performance of a seven-story full-scale structure. Through linear dynamic analysis, it was concluded that TMD could reduce the acceleration of the wood structure floor by up to 40%.
As shown in Fig. 13, the team of Tongji University studied an innovative self-centering steel-wood hybrid shear wall (SC-STHSW) system, which was composed of the post-tensioned (PT) steel frame, and the infilled light-frame wood shear wall. The friction damper was assembled into the connection between the steel frame and the infilled wall. Li et al. [91,92] tested the mechanical properties of friction dampers and found that the activation force of the damper was linearly positively correlated with the amount of torque applied to the high-strength bolt. Afterward, the damper was introduced into the steel-wood hybrid shear wall. The reasonable setting of damper activation force and slotted hole length could reduce the damage to the shear wall systems under earthquake action. Cui et al. [93] conducted analysis and tests to verify the self-centering mechanism of the systems and demonstrated that the systems could withstand large inter-story displacements, and no plastic zone was formed in steel frame members. Dong et al. [94] proposed a slip-frictional damper connection with a lock-up system between walls and frames and established a numerical model to further investigate the influence of wall-to-frame stiffness ratios on the lateral performance for shear walls.
Figure 13: Self-centering steel–wood hybrid shear wall [93]
To sum up, the steel-wood hybrid structure could meet the structural requirements of modern buildings, thus gaining more attention [95]. The innovative introduction of friction dampers in the steel-wood hybrid shear wall system not only provided higher energy dissipation and reduced the damage deformation but also improved the seismic performance of the wall.
5 Influence of Other Factors on Wall Performance
Compared to engineering wood products such as laminated wood, CLT had ideal in-plane compressive strength, high stiffness, good integrity, and similar properties in the in-plane orthogonal direction, which showed a good development prospect in mid-and high-rise buildings, thus attracted much attention.
During the study of the wallboard, a theoretical model was proposed to predict the performance of CLT suffered to lateral load [96], and the error of the prediction results was confirmed to be within the allowable range by experimental and simulation results. Wang et al. [97,98] measured the performance of hybrid CLT shear walls fabricated by lumber and laminated veneer lumber (LVL) and marked that the lateral stiffness of hybrid CLT shear walls was higher than that of generic CLT shear walls fabricated by lumber, but the shear strength was lower. For the research on CLT floors, Loss et al. [99,100] presented an innovative steel-wood hybrid floor and discussed the in-plane stiffness, concluding that the floor diaphragm combining CLT panels with steel members was an effective solution to replace ordinary wood or wood-concrete. In terms of CLT connection, Gavric et al. [101,102] conducted a study on the screwed connections and metal connectors, compared the test results with the analytical formula calculation results provided in the code, and put forward new suggestions for the design requirements of the two. Dong et al. [103] selected a self-tapping screw (STS) to connect CLT by direct connection and two-way inclined nails and concluded that increasing the length and diameter of STS could markedly enhance CLT shear strength.
In the preparation of the CLT shear wall structure, vertical split joints were set between CLT shear walls to build a large area of wall panels, thus meeting the design and construction requirements. The design principles of vertical split joints were introduced in the Canadian CLT handbook [104], and the method for calculating the lateral resistance of CLT shear walls with vertical split joints as specified in the North American Wood Structure Design Manual [105]. All these provided the theoretical and scientific basis for scholars to further study the performance of shear walls with vertical split joints.
Casagrande et al. [106] and Nolet et al. [107] proposed a corresponding theoretical calculation model for CLT shear walls with vertical joints and verified the rationality of the predicted mechanical properties of shear walls by analysis. Based on the elastic foundation model, Chen et al. [108] performed a parametric analysis of CLT shear walls and predicted the influence of parameters such as joint stiffness at vertical split joints on the lateral performance of CLT shear walls. As shown in Fig. 14, Wang et al. [109] further explored the effect of vertical split joints on the lateral performance of CLT shear walls. It was found that the performance of the CLT shear wall decreased in ultimate load-resisting capacity, initial stiffness, and energy dissipation capacity after vertical split joints were set. Whereas, under the same lateral displacement, the deformation of the shear wall without vertical split joints was more serious. Related tests are also being carried out in other studies [110,111].
Figure 14: Layout of CLT shear wall: (a) CLT shear wall with vertical split-joints; (b) CLT shear wall without vertical split-joints; (c) Section A-A; (d) Section B-B [109]
As far as the construction method of the CLT shear wall structure was concerned, it could be divided into platform-type and balloon-type shown in Fig. 15, according to the different connection modes of the wall and floor [112]. Among them, the platform type had the advantages of convenient construction and a clear force transmission path, while the balloon-type retained the integrity of the shear wall, which could effectively avoid the transverse bearing pressure of the CLT floor at the connection. Popovski et al. [113] investigated the platform-type CLT shear wall with different aspect ratios, connectors, and screws and determined the main performance of the wall through the defined Equivalent Energy Elastic Plastic (EEEP) curves. Based on the developed advanced analysis model, Gavric et al. [114] conducted parameter analysis on the CLT wall systems with different aspect ratios and wall segmentation. Yasumura et al. [115] performed a 3D nonlinear analysis via the finite-element method (FEM), accurately predicting the lateral performance of the wall structure. Ceccotti et al. [116,117] examined the seismic performance of platform-type shear walls through the destruction of the three-and seven-story full-scale CLT buildings and calculated the seismic performance coefficient. Related experiments were also conducted in the study of Ou et al. [118] and He et al. [119].
Figure 15: Construction methods of CLT structures: (a) Platform-type; (b) Balloon-type [119]
Previous studies [120–124] have shown that the early damage of CLT shear walls tended to occur at the connection between the panel and the foundation under lateral loads. Once the connection was damaged, the lateral bearing capacity of the wall would drop abruptly, leading to the failure of the whole system. In response to this phenomenon, the idea of interposing prestressed tendons in CLT shear walls had been proposed [125,126], and due to the presence of prestressing, static friction was generated when the wallboard was in contact with the foundation surface to resist the horizontal load, which not only prevented the connection from premature failure but also improved the lateral performance of the CLT shear wall.
Furthermore, the hybrid shear wall system, composed of a post-tensioned rocking CLT wall panel and conventional light-frame wood shear wall panels, was investigated [127–131]. In this case, the post-tensioned CLT panels provided the self-centering capability, and the conventional light-frame timber shear wall system played an energy dissipation role. By combining the advantages of both, the system exhibited excellent performance and could recover from damage after multiple earthquakes.
Until now, scholars have conducted extensive experimental studies on various factors, including wallboard, floor, connection, and construction method [132–135]. To further improve the performance of the shear wall to meet the higher building demand, different measures such as vertical joints, prestressing, and post-tensioned wall panels were considered based on the traditional shear wall. However, more comprehensive and systematic tests and analyses are still needed in the future to provide new ideas for the development of modern multi-story and high-rise wood structures.
According to the experimental studies on wood-frame shear walls in recent years, this paper analyzed the factors that affected the lateral performance of wood-frame shear walls and discussed the problems that still needed to be improved during the comparison. The following conclusions can be obtained:
(1) The material and size of the wood-frame shear wall members have an effect on its ultimate load and stability. It is better to select panel materials with high strength, set multiple wall columns at corners or junctions, and stagger the positions of nails. The combination of the composite frame can not only make up for the strength loss caused by the opening but also give full play to the performance of the material to achieve twice the result with half the effort.
(2) To face the challenges brought by the development of modern architecture, we must enhance the lateral performance of wood-frame shear walls to meet greater building demands. It is proved that the combination of shear walls with a diagonal brace or friction dampers can markedly enhance the integrity of systems and provide more energy dissipation to avoid serious damage.
(3) Different forms of hybrid structures have also been tried in conjunction with wood-frame shear walls, with more sophisticated connectors and anchorages. Therefore, more detailed experiments and theoretical analysis are required, and the corresponding finite element simulation also needs to be further optimized.
With the change in the housing concept, a diversified pattern of concrete structures, brick concrete structures, steel structures, wood structures, and bamboo structures will gradually take shape. To further study wood structures and apply the wood-frame shear walls, it is necessary to continue to carry out in-depth research work in many aspects.
(1) For the sustainable development of the construction industry, the combination of shear walls with resource-rich bamboo has been proven feasible. However, there is still a lack of detailed research on lateral performance, so the research in this field should be expanded.
(2) In practical application, to meet the mechanical performance requirements of modern high-rise wood structures, it is necessary to conduct research on the performance of hybrid structures through experiments and numerical simulation.
(3) To better predict the seismic performance of wood shear walls, more shaking-table tests can be conducted to obtain accurate data, which is a worthwhile research direction and can provide a further reference for the design and construction of wood structures.
Funding Statement: This work was supported by the National Natural Science Foundation of China (Nos. 51878354 & 51308301), the Natural Science Foundation of Jiangsu Province (Nos. BK20181402 & BK20130978), 333 Talent High-Level Project of Jiangsu Province, and Qinglan Project of Jiangsu Higher Education Institutions. Any research results expressed in this paper are those of the writer(s) and do not necessarily reflect the views of the foundations.
Conflicts of Interest: The authors declare that they have no conflicts of interest to report regarding the present study.
References
1. Li, H. T., Chen, B., Fei, B. H., Li, H., Xiong, Z. H. et al. (2022). Mechanical properties of aramid fiber reinforced polymer confined laminated bamboo lumber column under cyclic loading. European Journal of Wood and Wood Products, 80, 1057–1070. DOI 10.1007/s00107-022-01816-4. [Google Scholar] [CrossRef]
2. Zhang, D. Y., Gong, M., Zhang, S. J., Zhu, X. D. (2022). A review of tiny houses in North America: Market demand. Sustainable Structures, 2(1), 000012. DOI 10.54113/j.sust.2022.000012. [Google Scholar] [CrossRef]
3. Jian, B. Y., Mohrmann, S., Li, H. T., Li, Y. J., Ashraf, M. et al. (2022). A review on flexural properties of wood-plastic composites. Polymers, 14, 3942. DOI 10.3390/polym14193942. [Google Scholar] [CrossRef]
4. Cai, S. X., Li, Y. J., Huang, Y. P., Guo, Y. L. (2021). Study on correlation between mechanical measurement of wood cell wall and added load by nano-indentation. Journal of Forestry Engineering, 6(4), 64–71. DOI 10.13360/j.issn.2096-1359.202010021. [Google Scholar] [CrossRef]
5. Li, H. T., Xu, W., Chen, C., Yao, L. S., Lorenzo, R. (2022). Temperatures influence on the bending performance of laminated bamboo lumber. Journal of Materials in Civil Engineering (Accepted). DOI 10.1061/(ASCE)MT.1943-5533.0004730. [Google Scholar] [CrossRef]
6. Liu, W. Q., Yang, H. F. (2019). Research progress on modern timber structures. Journal of Building Structures, 40(2), 16–43. DOI 10.14006/j.jzjgxb.2019.02.002. [Google Scholar] [CrossRef]
7. Zhou, Y. H., Huang, Y. J., Sayed, U., Wang, Z. (2021). Research on dynamic characteristics test of wooden floor structure for gymnasium. Sustainable Structures, 1(1), 000005. DOI 10.54113/j.sust.2021.000005. [Google Scholar] [CrossRef]
8. Liu, Y. F., Hou, T. Y., Teng, Q. C., Lv, H. Y., Li, Z. R. et al. (2021). Quasi-static experiment on bracket complexes model of the main hall of the ancestor’s monastery in shaolin temple. Journal of Forestry Engineering, 6(5), 46–53. DOI 10.13360/j.issn.2096-1359.202011024. [Google Scholar] [CrossRef]
9. He, M. J., He, G. R., Liang, F., Li, Z. (2019). Development of timber structures in China during recent twenty years. Building Structure, 49(19), 83–90. DOI 10.19701/j.jzjg.2019.19.010. [Google Scholar] [CrossRef]
10. Peng, C., El Damatty, A. A., Musa, A., Hamada, A. (2020). Simplified numerical approach for the lateral load analysis of light-frame wood shear wall structures. Engineering Structures, 219, 110921. DOI 10.1016/j.engstruct.2020.110921. [Google Scholar] [CrossRef]
11. di Cesare, A., Ponzo, F. C., Pampanin, S., Smith, T., Nigro, D. et al. (2019). Displacement based design of post-tensioned timber framed buildings with dissipative rocking mechanism. Soil Dynamics Earthquake Engineering, 116, 317–330. DOI 10.1016/j.soildyn.2018.10.019. [Google Scholar] [CrossRef]
12. Shahnewaz, M., Popovski, M., Tannert, T. (2019). Resistance of cross-laminated timber shear walls for platform-type construction. Journal of Structural Engineering, 145(12), 04019149. DOI 10.1061/(ASCE)ST.1943-541X.0002413. [Google Scholar] [CrossRef]
13. Rinaldin, G., Amadio, C., Fragiacomo, M. (2013). A component approach for the hysteretic behaviour of connections in cross-laminated wooden structures. Earthquake Engineering & Structural Dynamics, 42(13), 2023–2042. DOI 10.1002/eqe.2310. [Google Scholar] [CrossRef]
14. Pozza, L., Scotta, R., Trutalli, D., Pinna, M., Polastri, A. et al. (2014). Experimental and numerical analyses of new massive wooden shear-wall systems. Buildings, 4(3), 355–374. DOI 10.3390/buildings4030355. [Google Scholar] [CrossRef]
15. Ashraf, M., Jobaer Hasan, M., Al-Deen, S. (2021). Semi-rigid behaviour of stainless steel beam-to-column bolted connections. Sustainable Structures, 1(1), 000002. DOI 10.54113/j.sust.2021.000002. [Google Scholar] [CrossRef]
16. Gong, M., Delahunty, S., Chui, Y. H. (2014). Influence of number of finger joints per stud on mechanical performance of wood shearwalls. Construction and Building Materials, 50, 335–339. DOI 10.1016/j.conbuildmat.2013.09.041. [Google Scholar] [CrossRef]
17. Zhang, X. F., Yang, R. Y., Liu, X. T., Sun, Y. F. (2021). Comparation of thermal insulation materials and steady state heat transfer performance of light-frame wood structures wall. Journal of Forestry Engineering, 6(2), 77–83. DOI 10.13360/j.issn.2096-1359.202008028. [Google Scholar] [CrossRef]
18. Casagrande, D., Rossi, S., Sartori, T., Tomasi, R. (2016). Proposal of an analytical procedure and a simplified numerical model for elastic response of single-storey timber shear-walls. Construction and Building Materials, 102, 1101–1112. DOI 10.1016/j.conbuildmat.2014.12.114. [Google Scholar] [CrossRef]
19. Rossi, S., Casagrande, D., Tomasi, R., Piazza, M. (2016). Seismic elastic analysis of light timber-frame multi-storey buildings: Proposal of an iterative approach. Construction and Building Materials, 102, 1154–1167. DOI 10.1016/j.conbuildmat.2015.09.037. [Google Scholar] [CrossRef]
20. Ponzo, F. C., di Cesare, A., Lamarucciola, N., Nigro, D. (2019). Seismic design and testing of post-tensioned timber buildings with dissipative bracing systems. Frontiers in Built Environment, 5, 104. DOI 10.3389/fbuil.2019.00104. [Google Scholar] [CrossRef]
21. Zhang, S. D., Ren, Z. Z., Wang, M. Z. (2021). Effect of zeolite precursor on properties of oriented strand board. Journal of Forestry Engineering, 6(2), 49–56. DOI 10.13360/j.issn.2096-1359.202007038. [Google Scholar] [CrossRef]
22. Shen, Y. L., Schneider, J., Tesfamariam, S., Stiemer, S. F., Mu, Z. G. (2013). Hysteresis behavior of bracket connection in cross-laminated-timber shear walls. Construction and Building Materials, 48, 980–991. DOI 10.1016/j.conbuildmat.2013.07.050. [Google Scholar] [CrossRef]
23. He, M. J., Zhou, N. N. (2011). Experimental study on racking performance of light-frame shear walls with various sheathing materials. Journal of Tongji University (Natural Science), 39(3), 340–345. DOI 10.3969/j.issn.0253-374x.2011.03.006. [Google Scholar] [CrossRef]
24. Du, M., Xie, B. Y., Fei, B. H., Wang, X. H., Liu, Y. (2013). Shear performance of wood-frame shear walls made of domestic material. Journal of Building Materials, 16(3), 487–490. DOI 10.3969/j.issn.1007-9629.2013.03.019. [Google Scholar] [CrossRef]
25. Chen, Z. Y., Chui, Y. H., Doudak, G., Nott, A. (2016). Contribution of type-X gypsum wall board to the racking performance of light-frame wood shear walls. Journal of Structural Engineering, 142(5), 04016008. DOI 10.1061/(ASCE)ST.1943-541X.0001468. [Google Scholar] [CrossRef]
26. Lafontaine, A., Chen, Z. Y., Doudak, G., Chui, Y. H. (2017). Lateral behavior of light wood-frame shear walls with gypsum wall board. Journal of Structural Engineering, 143(8), 04017069.1–04017069.8. DOI 10.1061/(ASCE)ST.1943-541X.0001798. [Google Scholar] [CrossRef]
27. Zhu, E. C., Chen, Z. Y., Chen, Y. K., Yan, X. Y. (2010). Testing and FE modeling of lateral resistance of shear walls in light wood frame structures. Journal of Harbin Institute of Technology, 42(10), 1548–1554. [Google Scholar]
28. Zhou, N. N. (2018). Experimental investigation on lateral performance of light-frame timber shear walls with different sheathing panels. Special Structures, 35(4), 8–12+21. DOI 10.3969/j.issn.1001-3598.2018.04.002. [Google Scholar] [CrossRef]
29. van de Lindt, J. W. (2004). Evolution of wood shear wall testing, modeling, and reliability analysis: Bibliography. Practice Periodical on Structural Design and Construction, 9(1), 44–53. DOI 10.1061/(ASCE)1084-0680(2004)9:1(44). [Google Scholar] [CrossRef]
30. Sinha, A., Gupta, R. (2009). Strain distribution in OSB and GWB in wood-frame shear walls. Journal of Structural Engineering, 135(6), 666–675. DOI 10.1061/(ASCE)0733-9445(2009)135:6(666). [Google Scholar] [CrossRef]
31. Li, Z., Xiao, Y., Wang, R., Shan, B. (2013). Experimental studies of light weight wood frame shear walls with ply-bamboo sheathing panels. Journal of Building Structures, 34(9), 142–149. DOI 10.14006/j.jzjgxb.2013.09.018. [Google Scholar] [CrossRef]
32. Wang, R., Xiao, Y., Li, Z. (2017). Lateral loading performance of lightweight glubam shear walls. Journal of Structural Engineering, 143(6), 04017020. DOI 10.1061/(ASCE)ST.1943-541X.0001751. [Google Scholar] [CrossRef]
33. Di, J., Zuo, H. L. (2021). Lateral performance of panel-frame connections in New-type strengthened light wood framed shear walls. Journal of Northeast Forestry University, 49(4), 81–84. DOI 10.13759/j.cnki.dlxb.2021.04.015. [Google Scholar] [CrossRef]
34. Zhou, H., Bo, J., Wen, W., Ren, T. L., Zhou, H. (2021). Lateral performance of shear wall frame of light timber structure. Journal of Forestry Engineering, 6(4), 72–79. DOI 10.13360/j.issn.2096-1359.202010020. [Google Scholar] [CrossRef]
35. Zhang, W. J., Sun, X. Y., Li, A. Y. (2020). Study on quasi-static test of lightweight composite wood shear walls with glass fiber-enhanced cladding. Building Structure, 50(16), 94–99. DOI 10.19701/j.jzjg.2020.16.016. [Google Scholar] [CrossRef]
36. Chen, S. L., Chen, Z. Y., Fan, C. M., Pan, J. L., Wang, H. D. (2008). Experimental study on nail fasteners in wood shear walls. Editorial Department of Journal of Sun Yatsen University, 4, 133–138. [Google Scholar]
37. Bagheri, M. M., Doudak, G. (2020). Structural characteristics of light-frame wood shear walls with various construction detailing. Engineering Structures, 205, 110093. DOI 10.1016/j.engstruct.2019.110093. [Google Scholar] [CrossRef]
38. Dinehart, D. W., Blasetti, A. S., Iii, H. (2008). Experimental cyclic performance of viscoelastic gypsum connections and shear walls. Journal of Structural Engineering, 134(1), 87–95. DOI 10.1061/(ASCE)0733-9445(2008)134:1(87). [Google Scholar] [CrossRef]
39. Wang, Q., Zhou, X. Y., Liu, Z. Y. (2015). Experimental research on rift grain compression property of glulam members and reinforced property of nailed connections. Structural Engineers, 31(1), 142–146. DOI 10.15935/j.cnki.jggcs.2015.01.022. [Google Scholar] [CrossRef]
40. Yang, X. J., Hao, D., Fu, S., Wang, J. Y., Zhang, L. et al. (2020). Lateral resistance of wood frame shear walls integrated with wood-plastic composites. Journal of Beijing Forestry University, 42(5), 155–163. DOI 10.12171/j.1000−1522.20190391. [Google Scholar] [CrossRef]
41. Xia, G. Y., Xu, D. L., Lu, W. D., Liu, W. Q. (2018). Experimental study on new type of stud-to-sheathing joints in light wood frame wall. Building Structure, 48(10), 4. DOI 10.19701/j.jzjg.2018.10.012. [Google Scholar] [CrossRef]
42. Wang, L., Qu, X. K. (2021). Experimental study on a new type of panel nailing joint for light wood-framed shear walls. Science Technology and Engineering, 21(2), 658–663. [Google Scholar]
43. Wang, L., Qu, X. K., Hu, X. Y., Liu, T., Zhu, Z. Y. (2021). Experimental study on the lateral resistance of a new type of shear wall of wood structure with nail joints with silica gel strip. Building Science, 37(5), 75–81. DOI 10.13614/j.cnki.11-1962/tu.2021.05.01. [Google Scholar] [CrossRef]
44. Doudak, G., Smith, I., Mcclure, G., Mohammad, M., Lepper, P. (2010). Tests and finite element models of wood light-frame shear walls with openings. Progress in Structural Engineering and Materials, 8(4), 165–174. DOI 10.1002/pse.223. [Google Scholar] [CrossRef]
45. Liu, Y. (2013). Research on the mechanical properties of wood-framed shear walls. Nanjing, China: Nanjing Forestry University. [Google Scholar]
46. Xue, J. Y., Ren, G. Q., Yuan, Z., Wang, P. F. (2021). Experimental study on seismic performance of glued-laminated timber frame infilled with CLT shear wall. Journal of Building Structures, 1–12. DOI 10.14006/j.jzjgxb.2021.0370. [Google Scholar] [CrossRef]
47. Xue, J. Y., Ren, G. Q., Qi, L. J., Wu, C. W., Yuan, Z. (2021). Experimental study on lateral performance of glued-laminated timber frame infilled with cross-laminated timber shear walls. Engineering Structures, 239(3), 112354. DOI 10.1016/j.engstruct.2021.112354. [Google Scholar] [CrossRef]
48. Fu, Y. X., Liu, Y., Miao, X. C., Song, Y. L., Tian, R. X. (2018). Finite element analysis of the mechanical properties of the wood frame shear wall under lateral load. China Forest Products Industry, 45(07), 10–13+46. DOI 10.19531/j.issn1001-5299.201807002. [Google Scholar] [CrossRef]
49. Branston, A. E., Boudreault, F. A., Chen, C. Y., Rogers, C. A. (2006). Light-gauge steel-frame wood structural panel shear wall design method. Canadian Journal of Civil Engineering, 33(7), 872–889. DOI 10.1139/L06-036. [Google Scholar] [CrossRef]
50. Boudreault, F. A., Blais, C., Rogers, C. A. (2007). Seismic force modification factors for light-gauge steel-frame-wood structural panel shear walls. Canadian Journal of Civil Engineering, 34(1), 56–65. DOI 10.1139/L06-097. [Google Scholar] [CrossRef]
51. Chen, C. Y., Boudreault, F. A., Branston, A. E., Rogers, C. A. (2011). Behaviour of light-gauge steel-frame-wood structural panel shear walls. Canadian Journal of Civil Engineering, 33(5), 573–587. DOI 10.1139/L06-015. [Google Scholar] [CrossRef]
52. Li, Z., He, M. J., Lam, F., Li, M. H. (2015). Load-sharing mechanism in timber-steel hybrid shear wall systems. Frontiers of Structural and Civil Engineering, 9(2), 203–214. DOI 10.1007/s11709-015-0293-y. [Google Scholar] [CrossRef]
53. Li, Z., He, M. J., Wang, X. J., Li, M. H. (2018). Seismic performance assessment of steel frame infilled with prefabricated wood shear walls. Journal of Constructional Steel Research, 140, 62–73. DOI 10.1016/j.jcsr.2017.10.012. [Google Scholar] [CrossRef]
54. Varoglu, E., Stiemer, S. F. (1998). Wood wall structure: US patent 5782054. [Google Scholar]
55. Hong, J. P., Ni, C., Vinson, M. (2012). Midply truss wall system: High-performance shear wall for midrise wood-frame buildings. Journal of Structural Engineering, 138(9), 1120–1127. DOI 10.1061/(ASCE)ST.1943-541X.0000536. [Google Scholar] [CrossRef]
56. Varoglu, E., Karacabeyli, E., Stiemer, S., Ni, C. (2006). Midply wood shear wall system: Concept and performance in static and cyclic testing. Journal of Structural Engineering, 132(9), 1417–1425. DOI 10.1061/(ASCE)0733-9445(2006)132:9(1417). [Google Scholar] [CrossRef]
57. Zheng, W., Lu, W. D., Liu, W. Q., Liu, X. X. (2016). Lateral force resistance performance of sheathing sandwiched wood shear walls. Journal of Vibration and Shock, 35(19), 94–100. DOI 10.13465/j.cnki.jvs.2016.19.016. [Google Scholar] [CrossRef]
58. Zheng, W., Lu, W. D., Liu, W. Q. (2016). Lateral performance and force mechanism of sheathing sandwiched wood shear walls. Journal of Nanjing Technology University (Natural Science Edition), 38(5), 13–20. DOI 10.3969/j.issn.1671-7627.2016.05.003. [Google Scholar] [CrossRef]
59. Zheng, W., Lu, W. D., Liu, W. Q., Wang, Z. Q., Li, Y. (2019). Experiment study on lateral performance of timber frame-midply shear wall hybrid system. Journal of Building Structures, 40(10), 180–187+206. DOI 10.14006/j.jzjgxb.2018.0556. [Google Scholar] [CrossRef]
60. Xue, J. C., Chen, Z. Q., Yang, H. F., Liu, W. Q. (2019). Experiment on seismic behavior of CLT infilled glulam frame structure. Journal of Civil Engineering and Management, 36(5), 150–156. DOI 10.13579/j.cnki.2095-0985.2019.05.022. [Google Scholar] [CrossRef]
61. He, M. J., Tao, L. (2011). Research on lateral performance of hybrid structure based on post-beam construction and light wood frame construction. International Conference on Multimedia Technology, pp. 4523–4527. Hangzhou, China. DOI 10.1109/ICMT.2011.6001749. [Google Scholar] [CrossRef]
62. Shim, K. B., Hwang, K. H., Park, J. S., Park, M. J. (2010). Shear performance of hybrid post and beam wall system infilled with structural insulation panel (SIP). Journal of the Korean Wood Science and Technology, 38(5), 405–413. DOI 10.5658/wood.2010.38.5.405. [Google Scholar] [CrossRef]
63. Zheng, W., Liu, X. X., Lu, W. D. (2014). Experimental study on lateral load resistance of glulam frame-shear wall structure. Earthquake Engineering and Engineering Dynamics, 34(2), 104–112. DOI 10.13197/j.eeev.2014.02.104.zhengw.014. [Google Scholar] [CrossRef]
64. Xiong, H. B., Liu, Y. Y., Yang, C. M., Qin, H. J. (2014). Experimental study on lateral resistance of glued-laminated timber post and beam systems. Journal of Tongji University (Natural Science), 42(8), 1167–1175. DOI 10.3969/i.issn.0253-374x.2014.08.004. [Google Scholar] [CrossRef]
65. Xiong, H. B., Li, B. Y., Liu, Y. Y., Yao, Y. (2015). Experimental study on timber post and beam frame-brace structure systems under lateral cyclic loading. Structural Engineers, 31(1), 147–154. DOI 10.15935/j.cnki.jggcs.2015.01.023. [Google Scholar] [CrossRef]
66. Cheng, H. J., Ni, C., Lv, X. L. (2006). Performance of perforated wood-frame shear walls with transverse walls and vertical load. China Civil Engineering Journal, (12), 33–40+47. DOI 10.15951/j.tmgcxb.2006.12.006. [Google Scholar] [CrossRef]
67. Liu, Y., Lu, W. S., Lv, X. L., Zhou, D. G. (2008). Effect of variable upper stiffness on the performance of wood shear walls. China Civil Engineering Journal, (11), 63–70. DOI 10.3321/j.issn:1000-131X.2008.11.010. [Google Scholar] [CrossRef]
68. Liu, Y., She, C. G., Zou, X. J. (2011). Test research on the mechanical properties of wood-frame shear walls. Structure Engineers, 27(S1), 184–189. DOI 10.15935/j.cnki.jggcs.2011.s1.03. [Google Scholar] [CrossRef]
69. Wadi, H., Amziane, S., Taazount, M. (2018). The lateral load resistance of unclassified cross-laminated timber walls: Experimental tests and theoretical approach. Engineering Structures, 166, 402–412. DOI 10.1016/j.engstruct.2018.03.077. [Google Scholar] [CrossRef]
70. Du, M., Xie, B. Y., Fei, B. H., Wang, X. H., Liu, Y. (2012). Shear performance of wood-frame shear walls with cross brace. Chinese Journal of Applied Mechanics, 29(03), 314–320+356. DOI CNKI:SUN:YYLX.0.2012-03-018. [Google Scholar]
71. Hou, J. (2017). The test research on lateral performance of prestressed cross bar timber-shear wall under monotonic load. Nanjing, China: Nanjing Forestry University. [Google Scholar]
72. Zuo, H. L., Di, J., Wang, T., Guo, N. (2019). Lateral performance of prestressed diagonal cross-bar reinforced timber shear walls under cyclic loading. Journal of Northeast Forestry University, 47(6), 61–64. DOI 10.13759/j.cnki.dlxb.2019.06.012. [Google Scholar] [CrossRef]
73. Yang, R. Y., Li, H. T., Dauletbek, A., Ashraf, M., Lorenzo, R. et al. (2021). Effect of freeze-thaw cycles on physical and mechanical properties of glulam exposed to outdoor environment. Journal of Renewable Materials, 9(7), 1293–1307. DOI 10.32604/jrm.2021.015296. [Google Scholar] [CrossRef]
74. Jiang, H. L., Liu, W., Huang, H. Y., Wang, Y. Q. (2022). Parametric design of developable structure based on yoshimura origami pattern. Sustainable Structures, 2(2), 000019. DOI 10.54113/j.sust.2022.000019. [Google Scholar] [CrossRef]
75. Liu, Y. Y., Li, A., Cao, J. X., Yu, D., Zhang, J. L. (2022). Mechanical properties of timber-concrete connections with steel tube connectors. Sustainable Structures, 2(2), 000017. DOI 10.54113/j.sust.2022.000017. [Google Scholar] [CrossRef]
76. Tesfamariam, S., Stiemer, S. F., Dickof, C., Bezabeh, M. A. (2014). Seismic vulnerability assessment of hybrid steel-timber structure: Steel moment-resisting frames with CLT infill. Journal of Earthquake Engineering, 18(5–6), 929–944. DOI 10.1080/13632469.2014.916240. [Google Scholar] [CrossRef]
77. Loss, C., Piazza, M., Zandonini, R. (2016). Connections for steel–timber hybrid prefabricated buildings. Part I: Experimental tests. Construction and Building Materials, 122, 781–795. DOI 10.1016/j.conbuildmat.2015.12.002. [Google Scholar] [CrossRef]
78. Loss, C., Piazza, M., Zandonini, R. (2016). Connections for steel–timber hybrid prefabricated buildings. Part II: Innovative modular structures. Construction and Building Materials, 122, 796–808. DOI 10.1016/j.conbuildmat.2015.12.001. [Google Scholar] [CrossRef]
79. He, M. J., Luo, Q., Li, Z., Dong, H. L., Li, M. H. (2018). Seismic performance evaluation of timber-steel hybrid structure through large-scale shaking table tests. Engineering Structures, 175, 483–500. DOI 10.1016/j.engstruct.2018.08.029. [Google Scholar] [CrossRef]
80. He, M. J., Li, Z., Lam, F., Ma, R., Ma, Z. (2014). Experimental investigation on lateral performance of timber-steel hybrid shear wall systems. Journal of Structural Engineering, 140(6), 04014029. DOI 10.1061/(ASCE)ST.1943-541X.0000855. [Google Scholar] [CrossRef]
81. Li, Z., He, M. J., Lam, F., Zhou, R. R., Li, M. H. (2017). Seismic reliability evaluation of steel-timber hybrid shear wall systems. Earthquakes and Structures, 13(3), 289–297. DOI 10.12989/eas.2017.13.3.289. [Google Scholar] [CrossRef]
82. Tomasi, R., Zandonini, R., Piazza, M., Andreolli, M. (2008). Ductile end connections for glulam beams. Structural Engineering International, 18(3), 290–296. DOI 10.2749/101686608785096595. [Google Scholar] [CrossRef]
83. Andreolli, M., Piazza, M., Tomasi, R., Zandonini, R. (2011). Ductile moment-resistant steel–timber connections. Proceedings of the Institution of Civil Engineers-Structures and Buildings, 164(2), 65–78. DOI 10.1680/stbu.9.00098. [Google Scholar] [CrossRef]
84. Komatsu, K., Mori, T., Kitamori, A., Araki, Y. (2014). Evaluation on dynamic performance of glulam frame structure composed of slotted bolted connection system. World Conference on Timber Engineering, Quebec City, Canada. http://hdl.handle.net/2433/235492. [Google Scholar]
85. Gohlich, R., Erochko, J., Woods, J. E. (2018). Experimental testing and numerical modelling of a heavy timber moment-resisting frame with ductile steel links. Earthquake Engineering and Structural Dynamics, 47(6), 1460–1477. DOI 10.1002/eqe.3025. [Google Scholar] [CrossRef]
86. Gilbert, C. F., Erochko, J. (2019). Development and testing of hybrid timber-steel braced frames. Engineering Structures, 198, 109495. DOI 10.1016/j.engstruct.2019.109495. [Google Scholar] [CrossRef]
87. He, M. J., Dong, W. C., Li, Z. (2018). Research on lateral performance of timber-steel hybrid walls with two different connection types under cycling loading. Building Structure, 48(10), 6. DOI 10.19701/j.jzjg.2018.10.009. [Google Scholar] [CrossRef]
88. Loo, W. Y., Quenneville, P., Chouw, N. (2012). A numerical study of the seismic behaviour of timber shear walls with slip-friction connectors. Engineering Structures, 34, 233–243. DOI 10.1016/j.engstruct.2011.09.016. [Google Scholar] [CrossRef]
89. Pu, W., Liu, C., Zhang, H., Kasai, K. (2016). Seismic control design for slip hysteretic timber structures based on tuning the equivalent stiffness. Engineering Structures, 128, 199–214. DOI 10.1016/j.engstruct.2016.09.041. [Google Scholar] [CrossRef]
90. Poh’sie, G. H., Chisari, C., Rinaldin, G., Amadio, C., Fragiacomo, M. (2016). Optimal design of tuned mass dampers for a multi-storey cross laminated timber building against seismic loads. Earthquake Engineering and Structural Dynamics, 45(12), 1977–1995. DOI 10.1002/eqe.2736. [Google Scholar] [CrossRef]
91. Li, Z., Chen, F., Dong, H. L., He, M. J., Li, M. H. (2020). Lateral performance of timber-steel hybrid shear wall with friction dampers under cyclic loading. Journal of Building Structures, 41(10), 1–10. DOI 10.14006/j.jzjgxb.2019.0259. [Google Scholar] [CrossRef]
92. Li, Z., Chen, F., He, M. J., Zhou, R. R., Cui, Y. et al. (2021). Lateral performance of self-centering steel-timber hybrid shear walls with slip-friction dampers: Experimental investigation and numerical simulation. Journal of Structural Engineering, 147(1), 04020291. DOI 10.1061/(ASCE)ST.1943-541X.0002850. [Google Scholar] [CrossRef]
93. Cui, Y., Shu, Z., Zhou, R. R., Li, Z., Chen, F. et al. (2020). Self-centering steel-timber hybrid shear wall with slip friction dampers: Theoretical analysis and experimental investigation. The Structural Design of Tall and Special Buildings, 29(15), e1789. DOI 10.1002/tal.1789. [Google Scholar] [CrossRef]
94. Dong, H. L., He, M. J., Christopoulos, C., Li, Z. (2021). Quasi-static tests and parametric simulations of hybrid steel frame and light wood shear walls with frictional dampers. Engineering Structures, 228, 111485. DOI 10.1016/j.engstruct.2020.111485. [Google Scholar] [CrossRef]
95. Yang, R. Y., Li, H. T., Lorenzo, R., Sun, Y. F., Ashraf, M. (2021). Flexural behaviour of steel timber composite (STC) beams. Steel and Composite Structures, 41(2), 193–207. DOI 10.12989/scs.2021.41.2.193. [Google Scholar] [CrossRef]
96. Izzi, M., Polastri, A., Fragiacomo, M. (2018). Investigating the hysteretic behavior of cross-laminated timber wall systems due to connections. Journal of Structural Engineering, 144(5), 04018035. DOI 10.1061/(ASCE)ST.1943-541X.0002022. [Google Scholar] [CrossRef]
97. Wang, Z. Q., Gong, M., Chui, Y. H. (2015). Mechanical properties of laminated strand lumber and hybrid cross-laminated timber. Construction and Building Materials, 101, 622–627. DOI 10.1016/j.conbuildmat.2015.10.035. [Google Scholar] [CrossRef]
98. Wang, Z. Q., Luo, D., Zheng, W., Yao, Y., Lu, X. N. (2017). Effect of hybrid structure on lateral load resistance of cross-laminated timber shear wall. Journal of Forestry Engineering, 2(2), 145–151. DOI 10.13360/j.issn.2096-1359.2017.02.024. [Google Scholar] [CrossRef]
99. Loss, C., Frangi, A. (2017). Experimental investigation on in-plane stiffness and strength of innovative steel-timber hybrid floor diaphragms. Engineering Structures, 138, 229–244. DOI 10.1016/j.engstruct.2017.02.032. [Google Scholar] [CrossRef]
100. Loss, C., Rossi, S., Tannert, T. (2018). In-plane stiffness of hybrid steel–cross-laminated timber floor diaphragms. Journal of Structural Engineering, 144(8), 04018128. DOI 10.1061/(ASCE)ST.1943-541X.0002105. [Google Scholar] [CrossRef]
101. Gavric, I., Fragiacomo, M., Ceccotti, A. (2015). Cyclic behavior of typical screwed connections for cross-laminated (CLT) structures. European Journal of Wood and Wood Products, 73(2), 179–191. DOI 10.1007/s00107-014-0877-6. [Google Scholar] [CrossRef]
102. Gavric, I., Fragiacomo, M., Ceccotti, A. (2015). Cyclic behaviour of typical metal connectors for cross-laminated (CLT) structures. Materials and Structures, 48(6), 1841–1857. DOI 10.1617/s11527-014-0278-7. [Google Scholar] [CrossRef]
103. Dong, W. Q., Yao, Y., Song, H., Qi, Y. Y., Ren, H. Q. et al. (2018). Shear strength of cross-laminated timber connected with self-tapping screws. China Wood Industry, 32(5), 1–5. DOI 10.19455/j.mcgy.20180501. [Google Scholar] [CrossRef]
104. Karacabeylie, Gagnons (2019). Canadian CLT handbook (2019 edition).Quebec: FPInnovations. [Google Scholar]
105. Canadian Wood Council (2017). Wood design manual.Ottawa: CSA Commentary. [Google Scholar]
106. Casagrande, D., Doudak, G., Mauro, L., Polastri, A. (2018). Analytical approach to establishing the elastic behavior of multipanel CLT shear walls subjected to lateral loads. Journal of Structural Engineering, 144(2), 04017193. DOI 10.1061/(ASCE)ST.1943-541X.0001948. [Google Scholar] [CrossRef]
107. Nolet, V., Casagrande, D., Doudak, G. (2019). Multipanel CLT shearwalls: An analytical methodology to predict the elastic-plastic behaviour. Engineering Structures, 179, 640–654. DOI 10.1016/j.engstruct.2018.11.017. [Google Scholar] [CrossRef]
108. Chen, Z. Y., Popovski, M. (2020). Mechanics-based analytical models for balloon-type cross-laminated timber (CLT) shear walls under lateral loads. Engineering Structures, 208, 109916. DOI 10.1016/j.engstruct.2019.109916. [Google Scholar] [CrossRef]
109. Wang, X. J., Li, Z., He, M. J. (2021). Influence of wall-to-wall vertical connections on the lateral performance of cross-laminated timber shear walls. Journal of Nanjing Tech University (Natural Science Edition), 43(3), 366–371. DOI 10.3969/j.issn.1671-7627.2021.03.012. [Google Scholar] [CrossRef]
110. Liu, J. J., Lam, F. (2018). Experimental test of coupling effect on CLT angle bracket connections. Engineering Structures, 171, 862–873. DOI 10.1016/j.engstruct.2018.05.013. [Google Scholar] [CrossRef]
111. Liu, J. J., Lam, F. (2019). Experimental test of coupling effect on CLT hold-down connections. Engineering Structures, 178, 586–602. DOI 10.1016/j.engstruct.2018.10.063. [Google Scholar] [CrossRef]
112. Karacabeyli, E., Douglas, B. (2013). CLT handbook: Cross-laminated timber.USA: FPInnovations and American Wood Council. [Google Scholar]
113. Popovski, M., Schneider, J., Schweinsteiger, M. (2010). Lateral load resistance of cross-laminated wood panels. World Conference on Timber Engineering, 20, 24. [Google Scholar]
114. Gavric, I., Fragiacomo, M., Ceccotti, A. (2015). Cyclic behavior of CLT wall systems: Experimental tests and analytical prediction models. Journal of Structural Engineering, 141(11), 04015034. DOI 10.1061/(ASCE)ST.1943-541X.0001246. [Google Scholar] [CrossRef]
115. Yasumura, M., Kobayashi, K., Okabe, M., Miyake, T., Matsumoto, K. (2016). Full-scale tests and numerical analysis of low-rise CLT structures under lateral loading. Journal of Structural Engineering, 142(4), E4015007. DOI 10.1061/(ASCE)ST.1943-541X.0001348. [Google Scholar] [CrossRef]
116. Ceccotti, A. (2008). New technologies for construction of medium-rise buildings in seismic regions: The XLAM case. Structural Engineering International, 18(2), 156–165. DOI 10.2749/101686608784218680. [Google Scholar] [CrossRef]
117. Ceccotti, A., Sandhaas, C., Okabe, M., Yasumura, M., Minowa, C. et al. (2013). SOFIE Project-3D shaking table test on a seven-storey full-scale cross-laminated timber building. Earthquake Engineering and Structural Dynamics, 42(13), 2003–2021. DOI 10.1002/eqe.2309. [Google Scholar] [CrossRef]
118. Ou, J. J., Yang, X. B., Wang, X. J., Li, Z. (2020). Lateral performance of cross-laminated timber walls with two different construction types. Industrial Construction, 50(3), 84–87+113. DOI 10.13204/j.gyjz202003014. [Google Scholar] [CrossRef]
119. He, M. J., Wang, X. J., Li, Z. (2020). Cyclic load capacity and deformation mode of cross-laminated timber shear walls. China Civil Engineering Journal, 53(9), 60–67. DOI 10.15951/j.tmgcxb.2020.09.007. [Google Scholar] [CrossRef]
120. Rinaldin, G., Fragiacomo, M. (2016). Nonlinear simulation of shaking-table tests on 3- and 7-storey X-Lam timber buildings. Engineering Structures, 113, 133–148. DOI 10.1016/j.engstruct.2016.01.055. [Google Scholar] [CrossRef]
121. Christovasilis, I. P., Brunetti, M., Follesa, M., Nocetti, M., Vassallo, D. (2016). Evaluation of the mechanical properties of cross laminated timber with elementary beam theories. Construction and Building Materials, 122, 202–213. DOI 10.1016/j.conbuildmat.2016.06.082. [Google Scholar] [CrossRef]
122. He, M. J., Sun, X. F., Li, Z. (2018). Bending and compressive properties of cross-laminated timber (CLT) panels made from Canadian hemlock. Construction and Building Materials, 185, 175–183. DOI 10.1016/j.conbuildmat.2018.07.072. [Google Scholar] [CrossRef]
123. Sun, X. F., He, M. J., Li, Z., Shu, Z. (2018). Performance evaluation of multi-storey cross-laminated timber structures under different earthquake hazard levels. Journal of Wood Science, 64(1), 23–39. DOI 10.1007/s10086-017-1667-7. [Google Scholar] [CrossRef]
124. Li, H., Wang, B. J., Wei, P., Wang, L. B. (2019). Cross-laminated timber (CLT) in China: A state-of-the-art. Journal of Bioresources and Bioproducts, 4(1), 22–31. DOI 10.21967/jbb.v4i1.190. [Google Scholar] [CrossRef]
125. Sun, X. F., He, M. J., Li, Z. (2020). Lateral performance of post-tensioned cross-laminated timber shear walls. China Civil Engineering Journal, 53(3), 11–18. DOI 10.15951/j.tmgcxb.2020.03.002. [Google Scholar] [CrossRef]
126. Sun, X. F., He, M. J., Li, Z. (2020). Experimental and analytical lateral performance of posttensioned CLT shear walls and conventional CLT shear walls. Journal of Structural Engineering, 146(6), 04020091. DOI 10.1061/(ASCE)ST.1943-541X.0002638. [Google Scholar] [CrossRef]
127. Ho, T., Dao, T., Aaleti, S., van de Lindt, J. W., Rammer, D. (2016). Hybrid system of unbonded post-tensioned CLT panels and light-frame wood shear walls. Journal of Structural Engineering, 143(2), 04016171. DOI 10.1061/(ASCE)ST.1943-541X.0001665. [Google Scholar] [CrossRef]
128. Akbas, T., Sause, R., Ricles, J. M., Ganey, R., Berman, J. et al. (2017). Analytical and experimental lateral-load response of self-centering posttensioned CLT walls. Journal of Structural Engineering, 143(6), 04017019. DOI 10.1061/(ASCE)ST.1943-541X.0001733. [Google Scholar] [CrossRef]
129. Ganey, R., Berman, J., Akbas, T., Loftus, S., Daniel Dolan, J. et al. (2017). Experimental investigation of self-centering cross-laminated timber walls. Journal of Structural Engineering, 143(10), 04017135. DOI 10.1061/(ASCE)ST.1943-541X.0001877. [Google Scholar] [CrossRef]
130. Anandan, Y. K., van de Lindt, J. W., Amini, M. O., Dao, T. N., Aaleti, S. (2021). Experimental dynamic testing of full-scale light-frame-CLT wood shear wall system. Journal of Architectural Engineering, 27(1), 04020042. DOI 10.1061/(ASCE)AE.1943-5568.0000443. [Google Scholar] [CrossRef]
131. Nguyen, T. T., Dao, T. N., Aaleti, S., van de Lindt, J. W., Fridley, K. J. (2018). Seismic assessment of a three-story wood building with an integrated CLT-lightframe system using RTHS. Engineering Structures, 167, 695–704. DOI 10.1016/j.engstruct.2018.01.025. [Google Scholar] [CrossRef]
132. Ponzo, F. C., Antonio, D. C., Nicla, L., Nigro, D. (2021). Experimental estimation of energy dissipated by multistorey post-tensioned timber framed buildings with anti-seismic dissipative devices. Sustainable Structures, 1(2), 000007. DOI 10.54113/j.sust.2021.000007. [Google Scholar] [CrossRef]
133. Ugalde, D., Almazán, J. L., Santa María, H., Guindos, P. (2019). Seismic protection technologies for timber structures: A review. European Journal of Wood and Wood Products, 77(2), 173–194. DOI 10.1007/s00107-019-01389-9. [Google Scholar] [CrossRef]
134. di Cesare, A., Ponzo, F. C., Lamarucciola, N., Nigro, D. (2020). Dynamic seismic response of nonlinear displacement dependent devices versus testing required by codes: Experimental case studies. Applied Sciences, 10(24), 8857. DOI 10.3390/app10248857. [Google Scholar] [CrossRef]
135. Quintana Gallo, P., Carradine, D. M., Bazaez, R. (2021). State of the art and practice of seismic-resistant hybrid timber structures. European Journal of Wood and Wood Products, 79(1), 5–28. DOI 10.1007/s00107-020-01556-3. [Google Scholar] [CrossRef]
Cite This Article
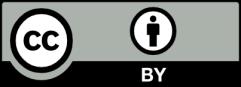