Open Access
ARTICLE
Effect of Peroxiredoxin 1 on the biological function of airway epithelial cells and epithelial-mesenchymal transition
Department of Critical Care Medicine, Mindong Hospital Affiliated to Fujian Medical University, Ningde, 355000, China
* Corresponding Author: LING GU. Email:
(This article belongs to the Special Issue: Single Cell Technologies and Molecular Mechanisms of Diseases)
BIOCELL 2022, 46(12), 2671-2680. https://doi.org/10.32604/biocell.2022.018054
Received 25 June 2021; Accepted 15 April 2022; Issue published 10 August 2022
Abstract
Peroxiredoxin 1 (PRDX1) participates in tumor cell proliferation, apoptosis, migration, invasion, and the epithelial-to-mesenchymal transition (EMT). This study aimed to investigate the effect of PRDX1 on the EMT of airway epithelial cells stimulated with lipopolysaccharide (LPS) and transforming growth factor-beta 1 (TGF-β1). PRDX1 overexpression significantly increased the proliferation and migration of human bronchial epithelial (BEAS-2B) cells, reduced cell apoptosis (p < 0.01), and induced EMT and collagen deposition by upregulating the expression of the matrix metallopeptidase (MMP)2, MMP9, α-smooth muscle actin (α-SMA), N-cadherin, vimentin and twist proteins and inhibiting E-cadherin expression (p < 0.05). PRDX1 overexpression promoted TGF-β1-mediated inhibition of cell proliferation and migration and significantly enhanced the TGF-β1-induced EMT and collagen synthesis (p < 0.05). Knockdown of PRDX1 inhibited cell proliferation, migration, EMT, and collagen synthesis (p < 0.01), reversed LPS-mediated inhibition of cell proliferation and migration, and significantly suppressed LPS-induced EMT and collagen synthesis (p < 0.01). The result indicating that PRDX1 may be involved in LPS/TGF-1-induced EMT and collagen synthesis in human bronchial epithelial cells.Keywords
Peroxiredoxin 1 (PRDX1) is proposed to play an important role in the pathogenesis of respiratory diseases such as acute respiratory distress syndrome, acute lung injury, and lung cancer (Chang et al., 2001; Liu et al., 2014). PRDX1 expression is significantly increased in lung tissues with acute injury and promotes airway inflammation through the activation of NF-κB signaling (Liu et al., 2014). PRDX1 also plays a central role in maintaining cell homeostasis and responses by eliminating various reactive oxygen species. For instance, airway epithelial cells lacking PRDX1 exhibit increased oxidative stress in response to respiratory syncytial virus infection (Chang et al., 2001). Inhibition of the synthesis of the antioxidant proteins PRDX1 and catalase leads to oxidative stress, which induces occupational asthma, an inflammatory disease characterized by airway remodeling (Kim et al., 2010). PRDX1 is also a major 2-Cys protein of the peroxiredoxin family of antioxidant enzymes and plays an important role in regulating cell growth, differentiation, and apoptosis (Chenbo, Xiaobo and Guoqiu, 2016; Mark et al., 2018). For example, PRDX1 directly binds to nuclear p53 or transcription factors such as c-Myc, NF-κB, and AR to influence the biological activity of genes, thereby inducing or suppressing cell death (Chenbo, Xiaobo and Guoqiu, 2016). In lung cancer, oral squamous cell carcinoma, and head and neck squamous cell carcinoma, PRDX1 overexpression promotes tumor growth and metastasis by activating the epithelial-to-mesenchymal transition (EMT) and NF-κB or Wnt/β-catenin signaling (Niu et al., 2016; Song et al., 2020; Jiang et al., 2019). PRDX1 plays an antiapoptotic role through direct or indirect interactions with several reactive oxygen species-dependent effectors, including ASK1, p66Shc, GSTpi/JNK, and c-Abl kinase (Ding et al., 2016). When regulating cell differentiation, PRDX1 represses the transformation of activated fibroblasts into cancer-associated fibroblasts through JNK inhibition (Jezierska-Drutel et al., 2019). In summary, many genes participate in the formation of a complete PRDX1 signaling network to regulate cellular biological functions.
PRDX1 is released by various cells, such as airway epithelial cells, human embryonic kidney cells, and monocytic cells, upon exposure to inflammatory stimuli, such as lipopolysaccharide (LPS) and TNF-α (Liu et al., 2013; Mullen et al., 2015; Liu et al., 2014). In vitro experiments have also confirmed that PRDX1 is involved in the regulation of LPS-induced injury. For example, LPS + elastase-induced chronic obstructive pulmonary disease results in the infiltration of inflammatory cells into the lungs and increased levels of proinflammatory cytokines and PRDX-1 (Singla et al., 2020). PRDX1 deficiency strongly protects mice from LPS-induced death and the inflammatory response (Ying et al., 2020). It is an enzyme that reduces oxidative stress. It alleviates LPS-induced acute lung injury by reducing oxidative stress and the activity of the P38/JNK signaling pathway (Ying et al., 2020). However, overexpression of PRDX1 in acute lung injury enhances the LPS-induced increase in the levels of the proinflammatory cytokines IL-6, IL-8, and TNF-α (Liu et al., 2014). In addition, PRDX1 knockout enhances LPS-induced liver apoptosis by increasing levels of the cleaved caspase-3 protein, resulting in high lethality after the LPS challenge (Sun et al., 2018). LPS induces cell damage and apoptosis and affects cellular EMT and matrix remodeling. In human peritoneal mesothelial cell lines, LPS induces EMT and fibrosis by upregulating α-smooth muscle actin (α-SMA), vimentin, MMP2/9, and collagen 1/3 and reducing E-cadherin expression (Shao et al., 2019). PRDX1 also plays an important role in the EMT and matrix remodeling. Loss of PRDX1 increases collagen remodeling associated with breast cancer progression (Attaran et al., 2021) and induces the expression of the metastasis-associated genes MMP1 and MMP2 (Yang et al., 2019). In head and neck squamous cell carcinoma and oral squamous cell carcinoma, PRDX1 overexpression promotes EMT and migration by inducing Vimentin expression and inhibiting E-cadherin expression (Jiang et al., 2019; Niu et al., 2016). Overall, LPS induces PRDX1 expression, which modulates the LPS-induced EMT and collagen remodeling.
The EMT allows epithelial cells to assume a mesenchymal cell phenotype and is a therapeutic target for several persistent inflammatory airway diseases related to tissue remodeling (Park et al., 2016; Lee et al., 2017). Transforming growth factor (TGF)-β1 is a multifunctional growth factor that regulates cell functions such as proliferation, differentiation, and migration and is involved in airway disease-related remodeling (Schleimer, 2017). TGF-β1 stimulates the expression of the EMT markers, including E-cadherin, fibronectin, vimentin, and α-SMA in airway cells and induces migration (Lee et al., 2017). In human conjunctival fibroblasts, TGF-β1 induced cell proliferation, collagen 1/3 synthesis, and MMP 2/9 expression (Sun et al., 2020). PRDX1 knockdown significantly inhibits the TGF-β1-induced EMT and A549 cell migration, whereas its overexpression enhances these processes (Ha et al., 2012). PRDX1 may be a key factor regulating the EMT and cell migration induced by TGF-β1.
Although PRDX1 has been implicated in inflammatory processes and tumor progression (e.g., cell growth, apoptosis, migration, EMT, and extracellular matrix synthesis), little is known about its role in the EMT and airway remodeling during inflammatory airway diseases. This study aimed to explore the role of PRDX1 in the acute injury of airway epithelial cells and extracellular matrix remodeling. Based on the results, PRDX1 knockdown improves the LPS- or TGF-β1-induced EMT and collagen synthesis in airway epithelial cells. Collectively, these findings provide new insight into the potential role of PRDX1 as a therapeutic target for inflammatory airway diseases.
Human bronchial epithelial cells (BEAS-2B) were purchased from the Shanghai Institute of Biochemistry and Cell Biology, Chinese Academy of Science (Shanghai, China) and cultured in high-glucose Dulbecco’s modified Eagle’s medium (DMEM; Gibco®, Grand Island, NY) containing 10% heat-inactivated fetal bovine serum (FBS; PAN Biotech, Germany), 1% 100 U/mL penicillin (MedChemExpress, USA) and 100 μg/mL streptomycin (MedChemExpress, USA). Cells were cultured in a humidified 5% CO2 incubator (SANYO, Japan) at 37°C.
Cell transfection and treatment
The PRDX1 overexpression plasmid pcDNA3.1-PRDX1, the knockdown plasmid pLVX-shRNA2-Puro-PRDX1, and the corresponding negative control plasmid (NC) were constructed by Zolgene Biotechnology (Fuzhou, China). Specifically, the sh-PRDX1, sh-NC, and PRDX1 sequences were amplified using the following primers: sh-PRDX1 5′-CACCGCACCATTGCTCAGGATTATGCGAACATAATCCTGAGCAATGGTGC-3′ (forward) and 5′-AAAAGCACCATTG CTCAGGATTATGTTCGCATAATCCTGAGCAATGGTGC-3′ (reverse), sh-NC 5′-GATCCGATCAATACTATTCATCAATTC AAGAGATTGATGAATAGTATTGATCTTTTTG-3′, and PRDX1 5’-CGGGATCCCGATGTCTTCAGGAAATGCTAA-3′ (forward) and 5′-CCCTCGAGGGTCACTTCTGCTTGGAGAAAT-3′ (reverse). Then, the DNA products were digested with the BamHI and EcoRI restriction enzymes (Fermentas, Lithuania) and inserted into the pLVX-shRNA2-Puro or pcDNA3.1 vector.
Before transfection, 5 × 105 BEAS-2B cells/well were inoculated into 6-well plates (Thermo Fisher Scientific, USA). When the cells grew to a density of approximately 60%–70%, transfection was performed along with Lipofectamine 2000 (Invitrogen, USA). After 6 h, DMEM containing 10% FBS was added to cells. After 24 h, the efficiency of PRDX1 knockdown and overexpression was analyzed by quantitative real-time polymerase chain reaction (qPCR) and western blotting. After transfection for 24 h, BEAS-2B cells were treated with 10 μg/mL LPS (Sigma–Aldrich, St. Louis, Missouri, USA) for 24 h or 10 ng/mL recombinant human TGF-β1 (PeproTech, Rock Hill, New Jersey, USA) (Liu et al., 2014; Sun et al., 2020). All experiments were repeated three times.
Quantitative real-time polymerase chain reaction
The cells were collected, and total RNA was extracted with TRIzol reagent (Invitrogen, USA). Reverse transcription was performed according to the manufacturer’s instructions for the PCR nucleotide mix (Promega, USA). Then, 2.0 μL of cDNAs, 2.0 μL of primers, and 8.5 μL of sterile water were mixed with 12.5 μL of SYBR Green qPCR master mix (A6001, Promega, USA) to make a final volume of 25 μL. Subsequently, the mixture was analyzed using an ABI 7500 PCR system (ABI, USA) to assess mRNA expression. The results were calculated using the 2−ΔΔCt method. The qPCR conditions were as follows: 95°C for 5 min, followed by 40 cycles of 95°C for 10 s, 60°C for 30 s, 72°C for 30 s, and 95°C for 15 s. The primers were designed and synthesized by Sangon Biotech (Shanghai, China). The PRDX1 primer sequences were 5′-CCCAACTTCAAAGCCACAGC-3′ (forward) and 5′-AAGCAATGATCTCCGTGGGG-3′ (reverse). The GAPDH primers were 5′-GTCATCCCTGAGCTGAACGG-3′ (forward) and 5′-CCACCTGGTGCTCAGTGTAG-3′ (reverse).
Cell viability was determined using the CCK-8 kit (Dojindo, China). BEAS-2B cells (1 × 105 cells/mL) were seeded in 96-well plates(Thermo Fisher Scientific, USA). The cells were incubated for 0 h, 24 h, 48 h, or 72 h at 37°C with 5% CO2. Approximately 100 μL of 10% CCK-8 reagent were added to each well of the plate. After incubation for 1 h, the optical density was detected at 450 nm using a microplate reader (DNM-9602, Beijing, China), and the reference wavelength was 650 nm.
The cells were harvested and washed with precooled PBS. The apoptosis ratio was detected using an Annexin V-FITC/PI kit (Solarbio, China). For this experiment, 100 μL of 1 × binding buffer and 5 μL of Annexin V-FITC were added to the cells and incubated at room temperature for 15 min. Then, 5 μL of PI was added and incubated at room temperature for 5 min in the dark. The number of apoptotic cells in each group was analyzed using a flow cytometer (Beckman Coulter, USA).
A total of 3 × 105 cells were cultured for 24 h in six-well plates. Subsequently, the Ibili-cell plug-in (Corning, USA) was removed. Then, the cells were cultured in a serum-free medium for 24 h and examined under an inverted microscope at 100 × magnification to measure the wound migration area with ImageJ software (National Institutes of Health, USA).
Protein samples were extracted from BEAS-2B cells with RIPA buffer containing PMSF (Beyotime, China). The total protein concentration was determined using a PC0020-500 BCA protein assay kit (Solarbio Technology Co., Ltd., Beijing, China). The proteins were separated on a 10% SDS-polyacrylamide gel and transferred to a pure nitrocellulose membrane (BioTrace, USA). The membrane was blocked with 5% bovine serum albumin (Beyotime, China) for 2 h and incubated with primary antibodies against PRDX1 (ab15571, 1:1000, 22 kDa), collagen 1 (ab34710, 1:1000, 130 kDa), collagen 3 (ab184993, 1:1000, 150 kDa), matrix metallopeptidase2 (MMP2, ab97779, 1:500, 74 kDa), MMP9 (ab38898, 1:1000, 92 kDa), Twist (ab175430, 1:1000, 21 kDa), α-SMA (ab5694, 1:500, 42 kDa), N-cadherin (ab76011, 1:5000, 100 kDa), glyceraldehyde 3-phosphate dehydrogenase (GAPDH) (ab8245, 1:5000, 36 kDa) (Abcam, Cambridge, UK), E-cadherin (3195S, 1:1000, 135 kDa), and vimentin (5741S, 1:1000, 57 kDa) (Cell Signaling Technology, USA) at 4°C overnight. The blots were then incubated with an HRP-conjugated secondary antibody (1:5000, Santa Cruz, USA) for 2 h. Subsequently, the chemiluminescence signal was detected using an electrochemiluminescence kit (Thermo Fisher Scientific, USA). The results were obtained with a Versa DocTM imaging system (Peiqing Technology Co., Ltd., Shanghai, China), analyzed using ImageJ software, and normalized to a housekeeping protein to determine the gray value of the target protein.
All statistical analyses were conducted using SPSS 20.0 statistical software. The data are presented as the means ± standard deviations. The paired t-test was used for comparisons between the two groups. One-way ANOVA with the LSD post hoc test was performed for comparisons between more than two groups. p < 0.05 was considered statistically significant.
Peroxiredoxin1-mediated promotion of the proliferation, migration, epithelial-mesenchymal transition, and collagen synthesis in BEAS-2B cells
Compared with the corresponding control group, transfection of pLVX-shRNA2-Puro-PRDX1 significantly inhibited the expression of the PRDX1 mRNA and protein, while transfection of pcDNA3.1-PRDX1 significantly increased PRDX1 mRNA (p < 0.01, Fig. 1A) and protein levels (p < 0.001, Fig. 1B). PRDX1 knockdown significantly inhibited cell proliferation (p < 0.01, Fig. 1C) and migration (p < 0.01, Fig. 1E) and significantly increased cell apoptosis (p < 0.001, Fig. 1D); however, PRDX1 overexpression exerted the opposite effects.
Figure 1: PRDX1 promoted the proliferation, migration, epithelial-mesenchymal transition (EMT), and collagen synthesis of BEAS-2B cells. PRDX1 mRNA (A) and protein (B) expression after transfection; PRDX1-mediated regulation of proliferation (C), apoptosis (D), and migration (E) of BESA-2B cells. (F) PRDX1 altered the expression of the EMT markers MMP2, MMP9, α-SMA, E-cadherin, N-cadherin, vimentin, and twist, and the synthesis of collagen 1 and collagen 3. All data are presented as the means ± standard deviations (n = 3). Compared with the NC group, *p < 0.05, **p < 0.01, and ***p < 0.001. sh, short hairpin; OE, overexpression; NC, negative control; OD, optical density; EMT: epithelial-mesenchymal transition; MMP: matrix metallopeptidase; α-SMA: alpha-smooth muscle actin; PRDX1: peroxiredoxin.
The western blot results showed that PRDX1 upregulated the expression of the EMT markers MMP2, MMP9, α-SMA, N-cadherin, vimentin, twist, and reduced collagen 1 and collagen 3 expression of the epithelial marker E-cadherin (p < 0.05, Fig. 1F). Downregulation of PRDX1 level inhibited the EMT and collagen biosynthesis (p < 0.01, Fig. 1F).
Peroxiredoxin1 enhanced lipopolysaccharide-mediated changes in BEAS-2B cell proliferation, migration, epithelial-mesenchymal transition, and collagen synthesis
LPS treatment significantly inhibited cell proliferation (p < 0.001, Fig. 2A) and migration (p < 0.001, Fig. 2C) and significantly increased cell apoptosis (p < 0.001, Fig. 2B). PRDX1 knockdown significantly reversed LPS-mediated inhibition of cell proliferation and migration (p < 0.05) and enhanced LPS-induced cell apoptosis (p < 0.01). However, PRDX1 overexpression rescued LPS-induced inhibition of cell proliferation and migration (p < 0.05) and suppressed LPS-induced cell apoptosis (p < 0.01).
Figure 2: PRDX1 enhanced LPS-mediated changes in BEAS-2B cell proliferation, migration, EMT, and collagen synthesis. After transfection for 24 h, BEAS-2B cells were treated with 10 μg/mL LPS for 24 h. PRDX1 rescued LPS-mediated inhibition of cell proliferation (A), suppressed LPS-induced cell apoptosis (B), and reversed the LPS-induced suppression of cell migration (C). (D) PRDX1 enhanced the LPS-induced expression of the EMT markers MMP2, MMP9, α-SMA, N-cadherin, vimentin, and twist, and collagen 1/3 synthesis, and the LPS-mediated inhibition of E-cadherin expression. All data are presented as the means ± standard deviations (n = 3). Compared with the control group, *p < 0.05, **p < 0.01, and ***p < 0.001. Compared with the LPS group, # p < 0.05, ## p < 0.01, and ### p < 0.001. sh, short hairpin; OE, overexpression; NC, negative control; OD, optical density; PRDX1: Peroxiredoxin1; LPS: lipopolysaccharide; EMT: epithelial-mesenchymal transition; MMP: matrix metallopeptidase; α-SMA: alpha smooth muscle actin.
Furthermore, LPS significantly led to an increase in the expression of the EMT markers MMP2, MMP9, α-SMA, N-cadherin, vimentin, and twist and the synthesis of collagen 1 and collagen 3 but decreased E-cadherin expression (p < 0.05, Fig. 2D). PRDX1 knockdown inhibited LPS-induced EMT marker expression and collagen synthesis, while PRDX1 overexpression enhanced the LPS-induced expression of these proteins (p < 0.05, Fig. 2D).
Peroxiredoxin1 enhanced TGF-β1-induced BEAS-2B cell proliferation, migration, epithelial-mesenchymal transition, and collagen synthesis
Cell proliferation (p < 0.001, Fig. 3A) and migration (p < 0.05, Fig. 3C) were significantly increased by TGF-β1, and cell apoptosis was significantly reduced (p < 0.01, Fig. 3B). Reduced expression of PRDX1 reversed TGF-β1-induced cell proliferation and migration and rescued TGF-β1-mediated inhibition of cell apoptosis (p < 0.05), while PRDX1 overexpression enhanced TGF-β1-mediated effects (p < 0.05).
Figure 3: PRDX1 enhanced TGF-β1-induced BEAS-2B cell proliferation, migration, EMT, and collagen synthesis. After transfection, BEAS-2B cells were treated with 5 ng/mL recombinant human TGF-β1 for 24 h. PRDX1 enhanced TGF-β1-induced cell proliferation (A), TGF-β1-mediated inhibition of cell apoptosis (B), and TGF-β1-induced cell migration (C). (D) PRDX1 promoted the TGF-β1-induced expression of MMP2, MMP9, α-SMA, vimentin, twist, collagen 1, and collagen 3 and enhanced TGF-β1-mediated inhibition of E-cadherin expression. All of the data are presented as the means ± standard deviations (n = 3). Compared with the control group, *p < 0.05, **p < 0.01, and ***p < 0.001. Compared with the TGF-β1 group, # p < 0.05, ## p < 0.01, and ### p < 0.001. sh, short hairpin; OE, overexpression; NC, negative control; OD, optical density; PRDX1: Peroxiredoxin1; EMT: epithelial-mesenchymal transition; MMP: matrix metallopeptidase; α-SMA: alpha-smooth muscle actin; TGF-β1: transforming growth factor β1.
Next, TGF-β1 treatment significantly increased the expression of the EMT markers MMP2, MMP9, α-SMA, N-cadherin, vimentin and twist, and the synthesis of collagen 1 and collagen 3 but reduced the level of E-cadherin (p < 0.05, Fig. 3D). PRDX1 knockdown inhibited TGF-β1-induced EMT and collagen synthesis, but increased PRDX1 level promoted the effect of TGF-β1 (p < 0.05).
PRDX1 is a key protein that regulates many physiological processes for the development of various respiratory diseases, cancers, septic shock, and other diseases (Mq et al., 2019; Sieńko et al., 2019). The respiratory epithelium forms an important barrier against inhaled pollutants and microorganisms, and its barrier function is often compromised during inflammatory airway diseases (Olson et al., 2011). LPS induces airway-centered inflammation and airway fibrosis and remodeling (Takahagi et al., 2020). According to recent studies, LPS induces EMT in BEAS-2B cells and mouse models, which are characterized by a decrease in E-cadherin levels, increased levels of α-SMA, vimentin, MMP2, and MMP9 proteins, and inflammatory response, leading to extracellular matrix deposition and lung fibrosis (Chen et al., 2020). TGF-β1 is a key growth factor involved in inducing inflammatory processes and EMT. TGF-β1 is upregulated in the airway epithelium of individuals with chronic obstructive pulmonary disease and induces the EMT in BEAS-2B cells (Zhu et al., 2021). In this study, PRDX1 was found to be involved in regulating the LPS- and TGF-β1-induced proliferation, EMT, and collagen synthesis in airway epithelial cells.
In the present study, PRDX1 induced BEAS-2B cell proliferation and migration and inhibited cell apoptosis, consistent with the role in cervical cancer (Lu et al., 2020). Overexpression of PRDX1 significantly promoted proliferation by upregulating Nanog and proliferating cell nuclear antigen, inhibited cell apoptosis by increasing B-cell lymphoma 2 (Bcl-2) expression, and downregulating Bcl-2-associated X protein, and promoted cell migration by upregulating Snail and MMP-9 expression and downregulating E-cadherin expression (Lu et al., 2020). A lowered expression of PRDX1 inhibited cell proliferation and migration through the inhibition of the p38 mitogen-activated protein kinase (MAPK) signaling pathway (Cong et al., 2018). PRDX1 forms a heterodimer with p38α MAPK-14, stabilizing phospho-p38α to amplify hepatocyte growth factor-mediated signaling and stimulate actin cytoskeleton dynamics that promote cell migration (Wirthschaft et al., 2018). After the disruption of intact epithelial cells, airway epithelial cells migrate first to replace damaged cells (Mccormack et al., 2013). The airway epithelial cells migrating at the wound edge acquire an EMT-like phenotype, manifested by a loss of E-cadherin expression and increased vimentin and α-SMA levels (Mccormack et al., 2013). Then, airway epithelial cells may transform into myofibroblasts through the EMT to promote matrix remodeling and cell migration (Minor and Proud, 2017). Approximately 30% of fibroblasts/myofibroblasts detected during airway remodeling are derived from the EMT (Johnson et al., 2011). Myofibroblasts are highly active profibrotic cells that secrete excessive extracellular matrix (ECM). The expression of α-SMA is a characteristic of myofibroblasts. The number of α-SMA+ myofibroblasts increases in subjects with chronic obstructive pulmonary disease, which might lead to increased collagen deposition, EMT activity in epithelial cells, and decreased lung function (Eapen et al., 2021). The role of the MMP family has been implicated in the EMT. In particular, MMP-2/9 inhibit E-cadherin expression and increase N-cadherin and vimentin levels by upregulating epidermal growth factor receptor, protein kinase B (AKT), and extracellular-signal-regulated kinase (ERK)1/2 in airway epithelial cells (Agraval and Yadav, 2019). In cystic fibrosis, twist is considered the driver of the EMT in endogenous airway tissue and primary cells (Quaresma et al., 2020). Here, we found that PRDX1 increased the expression of MMP 2 and MMP 9, induced the expression of the mesenchymal markers α-SMA, vimentin, twist, and N-cadherin, and inhibited the expression of the epithelial marker, E-cadherin in vitro. The increased α-SMA expression indicated that airway cells transformed from an epithelial to a myofibroblast phenotype, which promoted ECM collagen 1 and collagen 3 secretions. These results are similar to those reported in previous studies, namely, PRDX1-mediated changes in the expression of EMT markers to induce the EMT and upregulation of MMP 2/9 to increase the invasion and migration of cancer cells (Li et al., 2018; Jiang et al., 2019).
LPS and TGF-β1 are both inducers of EMT and fibrosis (Lee et al., 2017; Sun et al., 2020). In the present study, LPS induced apoptosis in BEAS-2B cells and reduced cell proliferation and migration. This finding was consistent with LPS-mediated cellular activities (Pooladanda et al., 2019; Sun et al., 2018). LPS induces PRDX1 expression in airway epithelial cells and macrophagocytes (Bast et al., 2010; Liu et al., 2014). PRDX1, in turn, promotes acute lung injury (Liu et al., 2014). Loss of PRDX1 protects against LPS-induced acute lung injury (Ying et al., 2020). The effects of LPS on upregulating MMP 2/9 expression, inducing EMT, and promoting collagen deposition have been confirmed (Shao et al., 2019). Our results show that PRDX1 not only enhanced the LPS-induced expression of EMT markers but also promoted LPS-induced MMP 2/9 expression and collagen production. Airway epithelial cells lost the expression of the epithelial protein E-cadherin, which is responsible for tight junctions, and gained the expression of the mesenchymal markers N-cadherin (promotes cell migration), vimentin (helps form the extracellular matrix), and twist (promotes fibroblast proliferation), leading to the disordered epithelial barrier (Nathan et al., 2018). The EMT alters cellular characteristics, such as enhanced migration, invasion, and wound healing (Samy Lamouille and Derynck, 2014). In breast cancer, PRDX1 functions as a tumor suppressor to inhibit collagen remodeling (Attaran et al., 2021). A lowered expression of PRDX1 promotes the production of reactive oxygen species and activation of NF-κB in breast cancer, consequently inducing the expression of the metastasis-related genes C-X-C chemokine receptor 4, MMP1, and MMP2 (Yang et al., 2019). Thus, different functions of PRDX1 in airway epithelial cells and breast cancer cells may be derived from specific cell types or disease progression.
TGF-β1, a representative profibrotic factor, induces airway tissue remodeling by activating the EMT (Park et al., 2016; Lee et al., 2017). Herein, we found that TGF-β1 treatment induced the proliferation, migration, and EMT of airway epithelial cells and decreased the rate of apoptosis. This finding was consistent with those reported in previous studies (Park et al., 2016; Sun et al., 2020). In the present study, PRDX1 enhanced the effect of TGF-β1 on BEAS-2B cells. TGF-β1 promotes corneal cell proliferation and wound healing by inducing PRDX1 expression (Tchah et al., 2005). TGF-β1-mediated induction of PRDX1 secretion (Chang et al., 2006) might be one of the mechanisms by which TGF-β1 induces the EMT. In particular, PRDX1 overexpression enhances the TGF-β1-induced EMT and cell migration in airway epithelial cells by reducing E-cadherin and increasing N-cadherin expressions (Ha et al., 2012). PRDX1 knockdown promotes cell proliferation, migration, invasion, and EMT in nasopharyngeal carcinoma by activating the phosphoinositide 3-kinases/AKT/TNF receptor-associated factor −1 signaling (Xiao et al., 2020). In lung cancer, PRDX1 protects DNA from oxidative damage induced by the cigarette carcinogen NNK and inhibits tumor growth. This phenotypic discrepancy arises from distinct roles of PRDX1 in certain cell types and tumor stages.
Collectively, in this study, PRDX1 promoted the proliferation, migration, EMT, and collagen deposition of BESA-2B cells. PRDX1 overexpression enhanced the LPS- and TGF-β1-induced EMT and collagen synthesis. Based on these results, PRDX1 may be involved in LPS/TGF-β1-induces EMT and collagen synthesis, and it may represent a new target for the prevention and treatment of airway injury.
However, the present study had a few limitations. First, the conclusion that PRDX1 enhanced the LPS- and TGF-β1-induced EMT and collagen synthesis is based on results obtained only from a human bronchial epithelial cell line (BEAS-2B). Although the role of PRDX1 in regulating the TGF-β1-induced EMT and fibrosis has been previously documented in A549 cells (Ha et al., 2012), further validation in other airway epithelial cell lines or primary cells is still needed. Second, in the present study, we could not construct an animal model to verify the role of PRDX1 in airway damage. While PRDX1 protects against LPS-induced acute lung injury (Liu et al., 2014; Ying et al., 2020) and liver injury (Sun et al., 2018) in mice by reducing oxidative stress and inflammation, there is no evidence that the PRDX1-regulated EMT and fibrosis affect airway injury in vitro. Therefore, more animal experiments are needed to determine the importance of PRDX1 as a treatment target for airway injury and its effects on the biological functions of other airway cells. Finally, although there were no significant differences in cell viability and changes in protein expression between cells transfected with si-NC and vector plasmids, some differences between the two groups should still be considered. Therefore, this conclusion must be confirmed in different cell lines.
Availability of Data and Materials: Data sharing is not applicable to this article as no datasets were generated or analyzed during the current study.
Author Contribution: Ling Gu and Huo-gen Liu contributed to the study conception and design. Ling Gu, Yun-di Shi, Hai-lin Shu, Feng-ming Huang, Huo-gen Liu, Xin Wan, Ying Liu, and Zhen-bin Gong contributed to data acquisition, analysis, and interpretation. Ling Gu participated in drafting the manuscript, and Huo-gen Liu revised it critically for its intellectual content.
Ethics Approval: Not applicable.
Funding Statement: The study was supported by the Fujian Provincial Science and Technology Department of the Project Fund under Grant (Grant No. 2015J01573) and the Medical Innovation Projects of Fujian Province’s Health and Scientific Research Talent Training Project under Grant (Grant No. 2019-CXB-25).
Conflicts of Interest: The authors declare that they have no conflicts of interest to report regarding the present work.
References
Li HX, Sun XY, Yang SM, Wang Q, Wang ZY (2018). Peroxiredoxin 1 promoted tumor metastasis and angiogenesis in colorectal cancer. Pathology-Research and Practice 214: 655–660. DOI 10.1016/j.prp.2018.03.026. [Google Scholar] [CrossRef]
Agraval H, Yadav UCS (2019). MMP-2 and MMP-9 mediate cigarette smoke extract-induced epithelial-mesenchymal transition in airway epithelial cells via EGFR/Akt/GSK3β/β-catenin pathway: Amelioration by fisetin. Chemico-Biological Interactions 314: 108846. DOI 10.1016/j.cbi.2019.108846. [Google Scholar] [CrossRef]
Pooladanda V, Thatikonda S, Bale S, Pattnaik B, Sigalapalli DK et al. (2019). Nimbolide protects against endotoxin-induced acute respiratory distress syndrome by inhibiting TNF-α mediated NF-κB and HDAC-3 nuclear translocation. Cell Death & Disease 10: 81. DOI 10.1038/s41419-018-1247-9. [Google Scholar] [CrossRef]
Bast A, Fischer K, Erttmann SF, Walther R (2010). Induction of peroxiredoxin I gene expression by LPS involves the Src/PI3K/JNK signalling pathway. Biochim Biophys Acta 1799: 402–410. DOI 10.1016/j.bbagrm.2009.11.015. [Google Scholar] [CrossRef]
Attaran S, Skoko JJ, Hopkins BL, Wright MK, Neumann CA (2021). Peroxiredoxin-1 Tyr194 phosphorylation regulates LOX-dependent extracellular matrix remodelling in breast cancer. British Journal of Cancer 8: 1146–1157. DOI 10.1038/s41416-021-01510-x. [Google Scholar] [CrossRef]
Chang JW, Hong BJ, Lee JH, Yoo JS, Chun JS et al. (2001). Augmented expression of peroxiredoxin I in lung cancer. Biochemical and Biophysical Research Communications 289: 507–512. DOI 10.1006/bbrc.2001.5989. [Google Scholar] [CrossRef]
Chang JW, Lee SH, Yan L, Yoo YJ (2006). Transforming growth factor-beta1 induces the non-classical secretion of peroxiredoxin-I in A549 cells. Biochemical and Biophysical Research Communications 345: 118–123. DOI 10.1016/j.bbrc.2006.04.073. [Google Scholar] [CrossRef]
Chen D, Qiu YB, Gao ZQ, Wu YX, Wan BB et al. (2020). Sodium propionate attenuates lipopolysaccharide-induced epithelial-mesenchymal transition via PI3K/Akt/mTOR signaling pathway. Journal of Agricultural and Food Chemistry 68: 6554–6563. DOI 10.1021/acs.jafc.0c01302. [Google Scholar] [CrossRef]
Ding CB, Fan XB, Wu GQ (2016). Peroxiredoxin 1-an antioxidant enzyme in cancer. Journal of Cellular and Molecular Medicine 21: 193–202. DOI 10.1111/jcmm.12955. [Google Scholar] [CrossRef]
Cong N, Huang W, Yuan JP, Li GZ, Li BS (2018). Peroxiredoxin1 promotes cell proliferation, migration and invasion of colorectal cancer via p38MAPK signaling. European Review for Medical and Pharmacological Sciences 22: 1922–1928. DOI 10.26355/eurrev_201804_14715. [Google Scholar] [CrossRef]
Eapen M, Lu W, Hackett T, Singhera G, Mahmood M et al. (2021). Increased myofibroblasts in the small airways, and relationship to remodelling and functional changes in smokers and COPD patients: Potential role of epithelial-mesenchymal transition. ERJ Open Research 7: 876–2020. DOI 10.1183/23120541.00876-2020. [Google Scholar] [CrossRef]
Ha B, Kim EK, KimJH Lee, Lee HN, Lee KO et al. (2012). Human peroxiredoxin 1 modulates TGF-β1-induced epithelial-mesenchymal transition through its peroxidase activity. Biochemical and Biophysical Research Communications 421: 33–37. DOI 10.1016/j.bbrc.2012.03.103. [Google Scholar] [CrossRef]
Park IH, Kang JH, Shin JM, Lee HM (2016). Trichostatin A inhibits epithelial mesenchymal transition induced by TGF-β1 in airway epithelium. PLoS One 11: e0162058. DOI 10.1371/journal.pone.0162058. [Google Scholar] [CrossRef]
Jezierska-Drutel A, Attaran S, Hopkins BL, Skoko JJ, Rosenzweig SA et al. (2019). The peroxidase PRDX1 inhibits the activated phenotype in mammary fibroblasts through regulating c-Jun N-terminal kinases. BMC Cancer 19: 812. DOI 10.1186/s12885-019-6031-4. [Google Scholar] [CrossRef]
Jiang Y, Cao W, Wu K, Qin X, Wang X et al. (2019). LncRNA LINC00460 promotes EMT in head and neck squamouHuman peroxiredoxin 1 into the nucleus. Journal of Experimental & Clinical Cancer Research 38: 365. DOI 10.1186/s13046-019-1364-z. [Google Scholar] [CrossRef]
Johnson JR, Abraham R, Tove B, Magnus N, Jonas F (2011). Chronic respiratory aeroallergen exposure in mice induces epithelial-mesenchymal transition in the large airways. PLoS One 6: e16175. DOI 10.1371/journal.pone.0016175. [Google Scholar] [CrossRef]
Kim SH, Choi GS, Ye YM, Jou I, Park HS et al. (2010). Toluene diisocyanate (TDI) regulates haem oxygenase-1/ferritin expression: Implications for toluene diisocyanate-induced asthma. Clinical and Experimental Immunology 160: 489–497. DOI 10.1111/j.1365-2249.2010.04118.x. [Google Scholar] [CrossRef]
Lee H, Kang J, Shin J, Lee S, Park I (2017). Chemical chaperone of endoplasmic reticulum stress inhibits epithelial-mesenchymal transition induced by TGF-1 in airway epithelium via the c-Src pathway. Mediators of Inflammation 2017: 8123281. DOI 10.1155/2017/8123281. [Google Scholar] [CrossRef]
Liu D, Mao P, Huang Y, Liu Y, Li Y (2014). Proteomic analysis of lung tissue in a rat acute lung injury model: Identification of PRDX1 as a promoter of inflammation. Mediators of Inflammation 2014: 469358. DOI 10.1155/2014/469358. [Google Scholar] [CrossRef]
Liu IT, Pu M, Liu XQ, He WQ, Li YM (2013). Lipopolysaccharide induced expression of peroxiredoxin 1 in airway epithelial cell. Zhonghua Wei Zhong Bing Ji Jiu Yi Xue 25: 136–139 (in Chinese). [Google Scholar]
Lu E, Hu X, Pan C, Chen J, Zhu X (2020). Up-regulation of peroxiredoxin-1 promotes cell proliferation and metastasis and inhibits apoptosis in cervical cancer. Journal of Cancer 11: 1170–1181. DOI 10.7150/jca.37147. [Google Scholar] [CrossRef]
Mark BH, Vick KA, Skoko JJ, Neumann CA (2018). Peroxiredoxin involvement in the initiation and progression of human cancer. Antioxidants & Redox Signaling 28: 591–608. DOI 10.1089/ars.2017.7422. [Google Scholar] [CrossRef]
Mccormack N, Molloy EL, O'Dea SJRR (2013). Bone morphogenetic proteins enhance an epithelial-mesenchymal transition in normal airway epithelial cells during restitution of a disrupted epithelium. Respiratory Research 14: 1–12. DOI 10.1186/1465-9921-14-36. [Google Scholar] [CrossRef]
Minor DM, Proud D (2017). Role of human rhinovirus in triggering human airway epithelial-mesenchymal transition. Respiratory Research 18: 110. DOI 10.1186/s12931-017-0595-9. [Google Scholar] [CrossRef]
Mq A, Li L, Lu Y, Chen H, Zhang M et al. (2019). Proteome profiling to identify peroxiredoxin 1 interacting protein partners in nicotine-associated oral leukoplakia. Archives of Oral Biology 108: 104537. DOI 10.1016/j.archoralbio.2019.104537. [Google Scholar] [CrossRef]
Mullen L, Hanschmann EM, Lillig CH, Herzenberg LA, Ghezzi P (2015). Cysteine oxidation targets peroxiredoxins 1 and 2 for exosomal release through a novel mechanism of redox-dependent secretion. Molecular Medicine 21: 98–108. DOI 10.2119/molmed.2015.00033. [Google Scholar] [CrossRef]
Nathan RP, Nigel F, David P, Martin D (2018). Epithelial mesenchymal transition (EMTU universal process in lung diseases with implications for cystic fibrosis pathophysiology. Respiratory Research 19: 136. DOI 10.1186/s12931-018-0834-8. [Google Scholar] [CrossRef]
Niu W, Zhang M, Chen H, Wang C, Shi N et al. (2016). Peroxiredoxin 1 promotes invasion and migration by regulating epithelial-to-mesenchymal transition during oral carcinogenesis. Oncotarget 7: 47042–47051. DOI 10.18632/oncotarget.9705. [Google Scholar] [CrossRef]
Olson N, Hristova M, Heintz NH, Lounsbury KM, Vliet A (2011). Activation of hypoxia-inducible factor-1 protects airway epithelium against oxidant-induced barrier dysfunction. American Journal of Physiology-Lung Cellular and Molecular Physiology 301: L993–L1002. DOI 10.1152/ajplung.00250.2011. [Google Scholar] [CrossRef]
Wirthschaft P, Bode J, Simon AEM, Hoffmann E, van Laack R et al. (2018). A PRDX1-p38α heterodimer amplifies MET-driven invasion of IDH-wildtype and IDH-mutant gliomas. International Journal of Cancer 143: 1176–1187. DOI 10.1002/ijc.31404. [Google Scholar] [CrossRef]
Quaresma MC, Pankonien I, Clarke LA, Sousa LS, Silva IAL et al. (2020). Mutant CFTR Drives TWIST1 mediated epithelial-mesenchymal transition. Cell Death & Disease 11: 920. DOI 10.1038/s41419-020-03119-z. [Google Scholar] [CrossRef]
Samy Lamouille JX, Derynck R (2014). Molecular mechanisms of epithelial-mesenchymal transition. Nature Reviews Molecular Cell Biology 15: 178–196. DOI 10.1038/nrm3758. [Google Scholar] [CrossRef]
Schleimer RP (2017). Immunopathogenesis of chronic rhinosinusitis and nasal polyposis. Annual Review of Pathology-Mechanisms of Disease 12: 331–357. DOI 10.1146/annurev-pathol-052016-100401. [Google Scholar] [CrossRef]
Shao Q, Jiang C, Xia Y, Zhao M, Zhang Q et al. (2019). Dioscin ameliorates peritoneal fibrosis by inhibiting epithelial-to-mesenchymal transition of human peritoneal mesothelial cells via the TLR4/MyD88/NF-κB signaling pathway. International Journal of Clinical and Experimental Pathology 12: 867–875. [Google Scholar]
Sieńko J, Teliga-Czajkowska J, Przytula E, Czajkowski K, Smolarczyk R et al. (2019). Peroxiredoxin-1 as a prognostic factor in patients with ovarian cancer. Annals of Agricultural and Environmental Medicine 26: 415–419. DOI 10.26444/aaem/105899. [Google Scholar] [CrossRef]
Singla E, Puri G, Dharwal V, Naura AS (2020). Gallic acid ameliorates COPD-associated exacerbation in mice. Molecular and Cellular Biochemistry 476: 293–302. DOI 10.1007/s11010-020-03905-5. [Google Scholar] [CrossRef]
Song C, Xiong G, Yang S, Wei X, Ye X et al. (2020). PRDX1 stimulates non-small-cell lung carcinoma to proliferate via the Wnt/β-Catenin signaling. Panminerva Medica. DOI 10.23736/S0031-0808.20.03978-6. [Google Scholar] [CrossRef]
Sun H, Feng L, Wang A, Wang J, Liu L et al. (2018). Peroxiredoxin I deficiency increases LPS‐induced lethal shock in mice. Molecular Medicine Reports 18: 2427–2432. DOI 10.3892/mmr.2018.9170. [Google Scholar] [CrossRef]
Sun L, Cui R, Meng H, Liu X, Liu X et al. (2020). Gene suppression of the ClC-2 chloride channel suppressed TGF-β1-induced proliferation, collagen synthesis, and collagen gel contraction mediated by conjunctival fibroblasts. Ophthalmic Research 64: 775–784. DOI 10.1159/000507632. [Google Scholar] [CrossRef]
Takahagi A, Sato M, Chen-Yoshikawa TF, Miyamoto E, Saito M et al. (2020). LPS-induced airway-centered inflammation leading to BOS-like airway remodeling distinct from RAS-like fibrosis in rat lung transplantation. Transplantation 104: 1150–1158. DOI 10.1097/TP.0000000000003097. [Google Scholar] [CrossRef]
Tchah H, Kim MJ, Kim TI, Choi HJ, Kim JY et al. (2005). Regulation of 1-Cys peroxiredoxin expression in the process of stromal wound healing after photorefractive keratectomy. Investigative Ophthalmology & Visual Science 46: 2396–2403. DOI 10.1167/iovs.05-0107. [Google Scholar] [CrossRef]
Xiao H, Yang T, Yan L, Feng J, Huang B et al. (2020). Therapy PRDX1 is a tumor suppressor for nasopharyngeal carcinoma by inhibiting PI3K/AKT/TRAF1 signaling. OncoTargets and Therapy 13: 9123–9133. DOI 10.2147/OTT.S252286. [Google Scholar] [CrossRef]
Yang L, Hong Q, Xu XG, Kuang XY, Di GH et al. (2019). Downregulation of transgelin 2 promotes breast cancer metastasis by activating the reactive oxygen species/nuclear factor-κB signaling pathway. Molecular Medicine Reports 20: 4045–4258. DOI 10.3892/mmr.2019.10643. [Google Scholar] [CrossRef]
Ying HA, Yu PA, Lt B, Zp B, Hy A (2020). Peroxiredoxin-1 aggravates lipopolysaccharide-induced septic shock via promoting inflammation. Biochemical and Biophysical Research Communications 527: 861–865. DOI 10.1016/j.bbrc.2020.04.149. [Google Scholar] [CrossRef]
Zhu J, Wang F, Feng X, Li B, Ma L et al. (2021). Family with sequence similarity 13 member A mediates TGF-β1-induced EMT in small airway epithelium of patients with chronic obstructive pulmonary disease. Respiratory Research 22: 192. DOI 10.1186/s12931-021-01783-z. [Google Scholar] [CrossRef]
Cite This Article
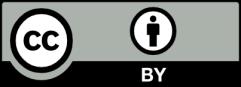