Open Access
ARTICLE
Photoprotective Effects of D1 Protein Turnover and the Lutein Cycle on Three Ephemeral Plants under Heat Stress
1 College of Life Sciences, Shihezi University, Shihezi, 832003, China
2 State Key Laboratory of Desert and Oasis Ecology, Xinjiang Institute of Ecology and Geography, Chinese Academy of Sciences, Urumqi, 830011, China
* Corresponding Author: Li Zhuang. Email:
(This article belongs to the Special Issue: Plant–Environment Interactions)
Phyton-International Journal of Experimental Botany 2023, 92(6), 1841-1857. https://doi.org/10.32604/phyton.2023.027369
Received 28 October 2022; Accepted 09 January 2023; Issue published 11 April 2023
Abstract
To clarify the characteristics of photoinhibition and the primary defense mechanisms of ephemeral plant leaves against photodestruction under high temperature stress, inhibitors and the technology to determine chlorophyll fluorescence were used to explore the protective effects of D1 protein turnover and the lutein cycle in the high temperature stress of the leaves of three ephemeral plants. The results showed that the maximum light conversion efficiency (Fv/Fm) of the ephemeral plant leaves decreased, and the initial fluorescence (Fo) increased under 35°C ± 1°C heat stress for 1–4 h or on sunny days in the summer. Both Fv/Fm and Fo could be recovered after 8 h of darkness or afternoon weakening of the external temperature. Streptomycin sulfate (SM) or dithiothreitol (DTT) accelerated the decrease of Fv/Fm and the photochemical quenching coefficient (qP) in the leaves of three ephemeral plants at high temperature, and the decrease was greater in the SM than in the DTT treatment. When the high temperature stress was prolonged, the Y(II) values of light energy distribution parameters of PSII decreased, and the Y(NPQ) and Y(NO) values increased gradually in all the treatment groups of the three ephemeral plants. The results showed that the leaves of the three ephemeral plants had their own highly advanced mechanisms to protect against photodamage, which inhibited the turnover of D1 protein and xanthophyll cycle. This can damage the PSII reaction center in the leaves of the three ephemeral plants under high temperature. The protective effect of D1 protein turnover on heat stress in Erodium oxyrrhynchum and Senecio subdentatus was greater than that of the lutein cycle, while the protective effect of lutein cycle was greater than that of D1 protein turnover in Heliotropium acutiflorum subjected to heat damage.Keywords
Plants often suffer from various biological and abiotic stresses during their growth, which seriously affect their growth and development [1]. A large number of studies have generally shown that high temperature is one of the most important environmental stresses that affects crop growth, yield and quality [2,3]. When plants are under abiotic stress conditions, such as high temperature, the rate of synthesis of photosystem II is often unable to compensate for the rate of damage, which results in photoinhibition [4]. During the long process of evolution, plants have developed a series of defense mechanisms to protect the normal physiological functions of their photosynthetic organs to adapt to changing growth environments. Examples of these mechanisms include reducing the capture of light energy in leaves [5], preventing photoinhibition under strong light and CO2 deficiency through biochemical mechanisms, such as the photocall Mehler reaction [6], regulating the distribution of excitation energy between the two photosystems through the state transition of PSII protein phosphorylation [7], and increasing the dependence of lutein circulation and energy dissipation mechanism related to D1 protein turnover [8,9]. A large number of studies have shown that turnover of the D1 protein and heat dissipation that are dependent on the lutein cycle are the main ways used by plants to protect the plant photosynthetic apparatus from photoinhibition [10–14]. Demmig-Adams et al. [15] concluded that heat dissipation related to the lutein cycle could be the main way to defend against light damage. However, energy dissipation that depends on the lutein cycle may not be the only mechanism because even the use of dithiothreitol (DTT), which inhibits the lutein cycle, failed to completely block the dissipation of energy. Aro et al. compared various strategies of photosynthesis to protect against the damage of bright light. The rapid synthesis of D1 protein is the most important process to defend the plants against photodestruction [16]. The D1 protein is the most basic framework of the photosystem II (PSII) reaction center, and it is encoded by the chloroplast psbA gene. It not only provides binding sites for the electron transport cofactors of the PSII reaction center and maintains the stability of the PSII reaction center structure but also plays a very important role in the PSII reaction center by participating in the primary charge separation and electron transport [14,16]. The net loss of PSII function is due to the fact that the rate of degradation of the D1 protein exceeds its rate of synthesis [17]. Therefore, under normal conditions, although there is inactivation of the PSII reaction center, no net loss of PSII function will be observed because the rates of synthesis and degradation of the D1 protein reach a dynamic equilibrium. However, when the synthesis of D1 protein is inhibited by reactive oxygen species or lincomycin, the function of PSII will be severely reduced [18]. The lutein cycle refers to the mutual transformation of three pigment components on photosynthetic membrane, including violaxanthin (V), antheraxanthin (A) and zeaxanthin (Z), according to the excess of excitation energy absorbed [19]. When there is excessive excitation energy, violaxanthin epoxidase (VDE) can transform violaxanthin epoxide into non-epoxidated zeaxanthin through antheraxanthin, which contains a single epoxide in the intermediate product. When the excess excitation energy is no longer contained, zeaxanthin epoxidase and anther xanthin can form purple xanthin by catalysis by cyclooxygenase (ZE). As early as the late 1950s, the relationship between lutein cycle and changes in light and dark conditions was discovered [20,21]. However, it was not until 1987 when Demming et al. [22] proposed the possible role of the lutein cycle in dissipating excess light energy that interest in this research area was stimulated. Since Demmig et al. [23] found that the existence of zeaxanthin may be related to the dissipation of excess light energy, the research on the lutein cycle has become an area of intensive research and a frontier issue in the field of plant physiology.
In recent years, global warming has seriously affected plant growth and reproduction. According to some researchers, if the global average temperature increases by more than 1.5°C~2.5°C, 20%~30% of species will be endangered [24], which poses a substantial threat to the fragile desert ecosystem. The desert ecosystem has a single biodiversity, simple structure, and serious habitat fragmentation, which further aggravates desertification [25]. Ephemeral plants, as the main vegetation groups in the early summer, play an important role in controlling the expansion of soil desertification and maintaining a stable ecosystem. Its distribution is centered in Central Asia and concentrated in Junggar Central Asia, the Mediterranean coast, Western Asia, North Africa, North America, and other vast areas of deserts. It is primarily distributed in the desert of Junggar Basin and the Yili Valley in China, and the main herbaceous plants in such deserts even form clusters and become unique vegetation types that differ from those of other deserts in China [26,27]. In Gurbantunggut Desert, the number of short-lived plant species accounts for 37.1% of the total plant population, and the spring green yield accounts for more than 60% of the community yield [28], which is of great significance for the protection and restoration of the vegetation in desert ecological environment. Ephemeral plants are types of herbaceous plants with special ecological types that rapidly complete their life cycle from March to May every year using snow water or spring rain [26]. They are primarily distributed in Xinjiang and Northeast China [29]. The lifecycles of ephemeral plants have gradually shortened over the years [30,31]. In addition to human intervention, the recent increase in global temperature is also one of the important factors that affects the survival and reproduction of ephemeral plants. Therefore, breeding ephemeral plants with strong resistance to light damage is an urgent problem to be solved in the restoration and reconstruction of desert ecosystems [32]. However, to our knowledge, there have been no studies on the characteristics of photoinhibition and the mechanism of defense against light damage of ephemeral plants.
DTT can inhibit the activity of cyclooxygenase of the lutein cycle, so it can inhibit the generation of epoxidation components of the lutein cycle and the heat dissipation that depends on the lutein cycle14.Streptomycin sulfate (SM) inhibits the synthesis of chloroplast proteins and therefore D1 protein synthesis and turnover [33]. For example, the non-radiation energy dissipation that depends on the lutein cycle plays a dominant role in wheat (Triticum aestivum), and reversible inactivation of the PSII reaction center is the main protective mechanism of soybean (Glycine max) against damage from high light [34]. Jie Minhua et al. treated broccoli (Brassica oleracea var. italica) leaves with DTT and SM, and the results showed that D1 protein turnover plays an important role in the defense of plant leaves to light damage, and its role is greater than that of the lutein cycle. Lou et al. [35] found that both DTT and SM could inhibit the non-photochemical quenching (NPQ) of Phyllostachthus leaves, and DTT was significantly more effective than SM. Xing et al. [36] found that the inhibition of D1 protein turnover could seriously damage grape (Vitis vinifera) leaf PSII under ozone stress. Zhang et al. [12] found that inhibition of D1 protein turnover under salt stress not only reduced the proportion of electron transport after absorbing light energy but also destroyed the excess light energy dissipated by lutein cycle, indicating that the protective effect of D1 protein turnover on PSII in mulberry (Morus sp.) leaves was greater than that of the lutein cycle. In mutants resistant to the herbicide atrazine, plants with disrupted lutein cycles were found to be more sensitive to high temperature stress [37]. Therefore, in this study, DTT and SM inhibitors were used to compare the roles of D1 protein turnover and lutein cycling in the defense of the leaves of three ephemeral plants to damage from high temperature and to clarify the main light-damage defense mechanisms of the three ephemeral plants. These experiments provide an important theoretical basis for the study of physiological ecology of desert plants and the screening of flora during the restoration and reconstruction of desert ecosystems.
2.1 Overview of the Study Area and Experimental Materials
The field data measurement area of the experiment was located in the Moso Bay Research Station in the Gurbantunggut Desert (86°01′E, 45°06′N). The area has a typical temperate arid climate with hot summers and maximum temperatures that exceed 40°C and long cold winters. The annual precipitation ranges from 70 to 150 mm, and the annual evaporation is approximately 1,000 mm [38]. The soil texture is single, typical desert aeolian sand soil [39], and the primary shrub is Ammodendrum ammodendrum. In May, the average coverage of ephemeral and quasi-ephemeral plants reaches approximately 40% [40]. The primary distribution of ephemeral plants includes Erodium oxyrrhynchum, Ceratocarpus arenarius, Senecio subdentatus, Heliotropium acutiflorum and Malcolmia africana among others.
The properties and characteristics of Erodium oxyrrhynchum, Senecio subdentatus and Heliotropium acutiflorum are shown in Table 1:
2.2 Determination of the Diurnal Variation of Fv/Fm and Fo in Three Ephemeral Plants
At the end of May 2021, the Fv/Fm and Fo values of the three ephemeral plants were measured using a Dual-PAM-100 dual-channel chlorophyll fluorescence measuring system (Walz, Effeltrich, Germany) every 3 h on three consecutive sunny days. Healthy and fully expanded functional leaves were selected for measurement. The values were measured at 8:00, 11:00, 14:00, 17:00 and 20:00, respectively. Each test was conducted in triplicate for each ephemeral plant.
2.3 High Temperature Stress and Inhibitor Treatment of the Isolated Plant Leaves
This study was conducted in the Key Laboratory of Mountain Basin System Ecology Corps, Oasis Town, Shihezi University (Shihezi, China).
DTT and SM treatments: Three ephemeral plants were cut from the base, and another section was quickly cut off and immersed in 5 mmol·L−1 streptomycin sulfate (SM, an inhibitor of D1 protein synthesis) or in 12 mmol·L−1 of dithiothreitol (DTT, an inhibitor of the lutein cycle) and deionized water (CK) at 20~30 μmol·m at 25°C−2s−1. They were treated under low light for 8 h to allow the inhibitors to fully enter the plant leaves. The concentrations of DTT (12 mmol·L−1) and SM (5 mmol·L−1) were chosen because we performed pre-experiments in the early stage. The concentration gradients of DTT and SM were established at 2, 4, 6, 8, 10, and 12 mmol·L-1. Three short-lived plant plants were cut off from the base, and another section was quickly cut off in solution. It was immersed in concentrations of 2, 4, 6, 8, 10, and 12 mmol·L−1 of solutions of DTT and SM. The plants were treated at 25°C, 20~30 μmol·m−2s−1, and the qP values of three ephemeral plants at different concentrations were determined every hour. It was finally found that after treatment with 12 mmol·L−1 DTT and 5 mmol·L−1, the qP values of the three ephemeral plants treated by SM remained basically unchanged after 6~8 h of treatment. Thus, the DTT concentration selected in this experiment was 12 mmol·L−1, and the SM concentration was 5 mmol·L−1 in the late-stage trials.
High temperature inhibition treatment: After the plants had been treated with inhibitors, they were subjected to 400 mol·m−2s−1. A previous study found that the optimal light intensity for ephemeral plant growth was 80~400 μmol·m−2 s−1. Thus, the light intensity was selected as 400 μmol·m−2 s−1 during the temperature stress in this experiment and 35°C ± 1°C. In recent years, ephemeral plants have transitioned from the flowering and fruiting stages to the end of May, and the entire lifecycle has basically been completed in early June. It rarely experiences a high temperature of more than 40°C, and this experiment only simulated the ambient temperature when the plants grow. Thus, the stress temperature was selected as 35°C ± 1°C as the high temperature for 4 h. Samples were collected every hour to determine the chlorophyll fluorescence parameters. After high temperature treatment, the chlorophyll fluorescence parameters were measured at 25°C after recovery for 1, 2, 4 and 8 h. Three leaves at each time point were sampled for measurements.
2.4 Determination of PSI and PSII Chlorophyll Fluorescence Parameters in the Treated Plants
The parameters were calculated as follows: after the plants were treated and adapted to the dark for 30 min, a Dual-PAM-100 was used to determine the relevant chlorophyll fluorescence parameters according to the manufacturer’s instructions and those of Kramer et al. [41]. First, the parameters were established. ML was clicked, and the measurement light was turned on. The initial fluorescence (Fo) was recorded after dark adaptation, and the saturated pulse light (10,000 μmol·m−2s−1) for 600 ms was used to measure the maximum fluorescence (Fm) after dark adaptation. The photochemical light was then turned on. The maximum fluorescence signal (Fm′) and maximum P700 signal (Pm′) under actinic light were determined by treatment with a saturated pulse light with a duration of 300 ms every 20 s after the actinic light began. Pm is similar to Pm′, but the photochemical light needs to be replaced by far-red light and turned off when the photosystem reaches dynamic equilibrium. The computer automatically calculates the quantum yield and maximum photochemical efficiency (F/Fm) of optical system II (PS II) and optical system I (photosystem I [PS I]) using the parameters described above. The parameters were calculated as follows (Table 2):
Maximum photosynthetic efficiency of PS II Fv/Fm = (Fm − Fo)/Fm
Actual photosynthetic efficiency of PS II Y(II) = (Fm′ − Fs)/Fm′
PS II quantum yield of non-regulated energy dissipation Y(NO) = Fs/Fm
PS II regulates the quantum yield of sexual energy dissipation Y(NPQ) = 1 − Y(II) − Y(NO)
Actual photosynthetic efficiency of PS I Y(I) = (Pm′ − P)/Pm
PS I Donor heat dissipation efficiency Y(ND) = P/Pm
PS I receptor heat dissipation efficiency Y(NA) = (Pm − Pm′)/Pm
Microsoft Excel YEAR (Redmond, WA, USA) was used to sort and calculate the basic data. A one-way analysis of variance (ANOVA) was used to analyze the chlorophyll fluorescence parameters of three ephemeral plants by SPSS 25.0 (IBM, Inc., Armonk, NY, USA). Origin 2018 (OriginLab, Northampton, MA, USA) was used to create the maps.
3.1 Diurnal Variation of Fv/Fm and Fo under Sunny Summer Days
The diurnal changes in the values of Fv/Fm and Fo of the three ephemeral plants are shown in Fig. 1. With the increase in external temperature, the diurnal changes of Fo and Fv/Fm showed an inverted “V” pattern, and the diurnal changes of Fv/Fm values showed a “V” pattern. Among the three ephemeral plants, the Fo and Fv/Fm values in Erodium oxyrrhynchum fluctuated the most each day, and the Fo and Fv/Fm values of the three ephemeral plants basically returned to the morning levels at 20:00.
Figure 1: Diurnal variation of Fv/Fm and Fo of three ephemeral plants on sunny days in the summer. Fo, initial fluorescence; Fv/Fm, maximum photosynthetic efficiency of photosystem II. Mean values of 3 repetitions ± SE. Different lowercase letters represent statistically significant differences (p < 0.05)
3.2 Effects of SM and DTT on Fv/Fm and Fo during High Temperature Stress and Low Temperature Recovery
After treatment with 35°C ± 1°C for 4 h, the Fv/Fm values of the three ephemeral plants in all the treatment groups were significantly lower than those at the pre-stress level (p < 0.05), and the Fo values were significantly higher than those at the pre-stress level. The Fv/Fm value of the CK group (control) of Erodium oxyrrhynchum, Senecio subdentatus and Heliotropium acutiflorum were 31.21%, 23.24% and 25.26% lower than those before stress, respectively. The Fo values increased by 119.69%, 59.42% and 32.35% compared with those before stress, respectively. The Fv/Fm values of E. oxyrrhynchum, S. subdentatus and H. acutiflorum in the SM group (treated with inhibitor SM) were 69.34%, 46.36% and 29.04% lower than those before stress, respectively. The Fo values increased by 174.79%, 144.61% and 72.33% compared with those before stress, respectively. The Fv/Fm values of E. oxyrrhynchum, S. subdentatus and H. acutiflorum in the DTT group were 47.66%, 34.24% and 67.45% lower than those before stress, respectively. The Fo values increased by 105.02%, 105.62% and 217.50% compared with those before stress, respectively. After 4 h of high temperature stress, the Fv/Fm values of E. oxyrrhynchum and S. subdentatus were SM < DTT < CK, and the Fo values were SM > DTT > CK, respectively. The Fv/Fm value sizes of H. acutiflorum were DTT < SM < CK, and the Fo value sizes were DTT > SM > CK.
During the recovery process at 23°C ± 1°C for 8 h, the Fv/Fm values of the three ephemeral plants in each treatment group gradually increased (Figs. 2A–2C), but the degrees of recovery were different. The Fv/Fm values in the Erodium oxyrrhynchum, Senecio subdentatus and Heliotropium acutiflorum CK groups basically recovered to the pre-high temperature stress level. In the SM group, the E. oxyrrhynchum, S. subdentatus and H. acutiflorum Fv/Fm values recovered to 72.41%, 72.77% and 85.79%, respectively. The Fv/Fm values of the three ephemeral plants in the DTT group recovered to 80.60%, 82.92% and 67.33%, respectively, after 8 h of low temperature. During the process of low-temperature recovery, the Fo values of the three ephemeral plants in each treatment group decreased gradually (Figs. 2D–2F). After low-temperature treatment for 8 h, the Fo values of the three ephemeral plants in group CK recovered completely. In the SM group, the Fo values of E. oxyrrhynchum, S. subdentatus and H. acutiflorum recovered to 143.44%, 156.03% and 115.51%, respectively. In the DTT group, the Fo values of E. oxyrrhynchum, S. subdentatus and H. acutiflorum recovered to 122.94%, 126.35% and 165.41%, respectively.
Figure 2: Effects of SM and DTT treatments on the leaf Fv/Fm and Fo values of three ephemeral plants during high temperature stress and low temperature recovery. 1, 2, 3 and 4 were 35°C ± 1°C for 1, 2, 3 and 4 h, respectively. R1, R2, R4 and R8 were 23°C ± 1°C for 1, 2, 4 and 8 h, respectively. CK, control; DTT, dithiothreitol; Fo, initial fluorescence; Fv/Fm, maximum photosynthetic ability of photosytemt II; SM, streptomycin sulfate. Mean values of 3 repetitions ± SE. Different lowercase letters represent statistically significant differences (p < 0.05)
3.3 Effects of SM and DTT on the Fm during High Temperature Stress and Low Temperature Recovery
Under high temperature stress at 35°C ± 1°C, the Fm values of the three ephemeral plants in each treatment group decreased as the stress time was extended (Fig. 3). After 4 h of stress, the Fm values of the three ephemeral plants were significantly lower than those before the stress (p < 0.05). The Fm values of Erodium oxyrrhynchum, Senecio subdentatus and Heliotropium acutiflorum in the CK group were 39.89%, 43.68% and 49.34% lower than those before stress, respectively. The Fm values of E. oxyrrhynchum, S. subdentatus and H. acutiflorum in the SM group decreased by 60.07%, 57.27% and 75.29% compared with those before stress, respectively, which were significantly lower than those in the CK group (p < 0.05). In the DTT group, the Fm values of E. oxyrrhynchum, S. subdentatus and H. acutiflorum decreased by 56.32%, 54.20% and 66.14%, respectively, after 4 h of high temperature stress. After this treatment, the Fm values of the three ephemeral plants in each treatment group were SM < DTT < CK. During the process of low temperature recovery, the Fm values of the three ephemeral plants in each treatment group gradually increased, but the degree of recovery differed. The Fm values of E. oxyrrhynchum, S. subdentatus and H. acutiflorum in the CK group recovered to 90.41%, 102.91% and 98.44%, respectively, after 8 h of low temperature treatment. The Fm values of E. oxyrrhynchum, S. subdentatus and H. acutiflorum in the SM group recovered by 64.21%, 51.54% and 68.74%, respectively. The Fm values of E. oxyrrhynchum, S. subdentatus and H. acutiflorum in the DTT group recovered to 68.12%, 60.82% and 70.25%, respectively.
Figure 3: Effects of SM and DTT treatments on the Fm of three ephemeral plants during high temperature stress and low temperature recovery. CK, control; DTT, dithiothreitol; Fm, maximum fluorescence yield; SM, streptomycin sulfate. Mean values of 3 repetitions ± SE. Different lowercase letters represent statistically significant differences (p < 0.05)
3.4 Effects of the SM and DTT Treatments on NPQ and qP during High Temperature Stress and Low Temperature Recovery
After treatment with 35°C ± 1°C for 4 h, the NPQ values of the three ephemeral plants in each treatment group were significantly higher than those before stress (p < 0.05), and the qP values were significantly lower than those before stress. The NPQ values of Erodium oxyrrhynchum, Senecio subdentatus and Heliotropium acutiflorum in the CK group increased by 160.61%, 231.48 and 254.57%, respectively, compared with those before treatment. The qP values were 64.97%, 57.44% and 45.31% lower than those before stress, respectively. The NPQ values of E. oxyrrhynchum, S. subdentatus and H. acutiflorum in the SM group were 132.38%, 126.34% and 191.26% higher than those before stress, respectively. The qP values decreased by 95.72%, 68.93% and 51.16% compared with those before stress, respectively. The NPQ values of E. oxyrrhynchum, S. subdentatus and H. acutiflorum in the group treated with the inhibitor DTT increased by 91.85%, 84.94% and 107.10% compared with those before stress, respectively. The qP values decreased by 87.61%, 77.02% and 95.83% compared with those before stress, respectively. After 4 h of high temperature stress, the qP values of E. oxyrrhynchum treatment groups were SM < DTT < CK, and the qP values of the S. subdentatus and H. acutiflorum treatment groups were DTT < SM < CK. However, the NPQ values of the three ephemeral plants were DTT < SM < CK.
During the process of recovery at 23°C ± 1°C, the NPQ values of the three ephemeral plants in each treatment decreased to differing degrees, and the qP values gradually increased. After 8 h of low-temperature treatment, the NPQ values of E. oxyrrhynchum, S. subdentatus and H. acutiflorum in CK group recovered to 136.36%, 101.87% and 152.30%, respectively, compared with those before stress. The qP values recovered by 92.75%, 91.04%, and 87.42% compared with those before high temperature stress. In group SM, the NPQ values of E. oxyrrhynchum, S. subdentatus and H. acutiflorum recovered to 171.64%, 176.66% and 215.95%, respectively. The qP values recovered to 45.14%, 64.01% and 74.72%, respectively. The NPQ values of E. oxyrrhynchum, S. subdentatus and H. acutiflorum recovered by DTT were 137.84%, 147.57% and 154.02%, respectively. The qP values recovered to 53.08%, 57.04% and 35.36%, respectively (Fig. 4).
Figure 4: Effects of SM and DTT treatments on the removal of NPQ and qP in the leaves of three ephemeral plants during high temperature stress and low temperature recovery. CK, control; DTT, dithiothreitol; NPQ, non-photochemical quenching; qP, photochemical quenching; SM, streptomycin sulfate. Mean values of 3 repetitions ± SE. Different lowercase letters represent statistically significant differences (p < 0.05)
3.5 Effects of SM and DTT Treatments on the PII Light Energy Allocation Parameters in the Leaves during Heat Stress and Cold Recovery
The chlorophyll fluorescence parameters enable division of the light quantum yield of PSII into three parts: the actual light quantum yield Y(II), the regulated energy dissipation quantum yield Y(NPQ) and the non-regulated energy dissipation quantum yield Y(NO), and Y(II) + Y(NPQ) + Y(NO) = 1. As shown in Fig. 5, the Y(II) values of the three ephemeral plants in each treatment decreased as the time of stress was prolonged during high temperatures. After 4 h of stress, the Y(II) values of the three ephemeral plants were significantly lower than those before stress (p < 0.05). The Y(II) values of the Erodium oxyrrhynchum and Senecio subdentatus groups were CK > DTT > SM, and the Y(II) values of the Heliotropium acutiflorum groups were CK > SM > DTT. The Y(NPQ) and Y(NO) values of the three ephemeral plants increased gradually during the process of high temperature stress, and the range of change of Y(NO) was greater than that of Y(NPQ). After 4 h of stress, the values of Y(NPQ) and Y(NO) in the E. oxyrrhynchum treatment groups were DTT > SM > CK and SM > DTT > CK, respectively, and the values of Y(NPQ) and Y(NO) in the S. subdentatus treatment groups were as follows: SM > CK > DTT and DTT > SM > CK. The values of Y(NPQ) and Y(NO) in H. acutiflorum were SM > DTT > CK and DTT > SM > CK.
Figure 5: Effects of SM and DTT treatments on PSII light energy allocation parameters in the leaves of three ephemeral plants during high temperature stress and low temperature recovery. DTT, dithiothreitol; PSII, photosystem II; SM, streptomycin sulfate, Y(II), actual photosynthetic efficiency of photosystem II; Y(NO), quantum yield of non-regulatory energy dissipation by photosystem II; Y(NPQ), photosystem II modulates the quantum yield of dissipation of regulatory energy. Mean values of 3 repetitions ± SE. Different lowercase letters represent statistically significant differences (p < 0.05)
3.6 Effects of SM and DTT Treatments on PSII Light Energy Allocation Parameters in the Leaves during Heat Stress and Cold Recovery
The effects of SM and DTT treatments on the quantum yield Y(I) of photochemical energy conversion, quantum yield Y(ND) of non-photochemical energy dissipation caused by donor side, and the quantum yield Y(NA) of non-photochemical energy dissipation caused by acceptor side restriction during the process of high temperature stress and low temperature recovery of the three ephemeral plants are shown in Fig. 6. It was found that Y(I) + Y(ND) + Y(NA) = 1. During the process of high temperature stress, the Y(I) values of the three ephemeral plants in each treatment decreased with the extension of time of stress. After 4 h of stress, the Y(I) values of Erodium oxyrrhynchum, Senecio subdentatus and Heliotropium acutiflorum in each treatment group were CK > SM > DTT. The Y(ND) values of the three ephemeral plants increased gradually during high temperature stress. After 4 h of stress, the Y(NA) values of E. oxyrrhynchum, S. subdentatus and H. acutiflorum in each treatment group were as follows: SM > DTT > CK. During the process of high temperature stress, the Y(ND) values of the three ephemeral plants decreased under SM treatment, while the Y(ND) values of the three ephemeral plants increased to some extent under DTT treatment, but the overall change was not significant.
Figure 6: Effects of SM and DTT treatment on the quantum yield Y(II) of photochemical energy conversion of PSII, the quantum yield Y(ND) of non-photochemical energy dissipation caused by the donor side, and the quantum yield Y(NA) of non-photochemical energy dissipation caused by acceptor side restriction in the three ephemeral plants during high temperature stress and low temperature recovery. DTT, dithiothreitol; PSI, photosystem I; SM, streptomycin sulfate. Mean values of 3 repetitions ± SE. Different lowercase letters represent statistically significant differences (p < 0.05)
In recent years, the photosuppression of plant photosynthesis has received substantial attention and become an important aspect of research on photosynthesis. The maximum light energy conversion efficiency Fv/Fm of PSII has proven to be a simple, quick, and sensitive indicator of light suppression by observation methods. Its downward trend is considered to be the primary condition for photosuppression in plants [42]. In general, in a healthy physiological state, the Fv/Fm value of mosthigher plants is approximately 0.8, and its Fv/Fm value decreases when subjected to stress [43]. Soybean grown under natural conditions [44], corn (Zea mays) [45] and rice (Oryza sativa) [46] and Linze small jujube (Zizyphus jujuba var. inermis) [47] Photoinhibition and Fv/Fm decreased in all the plants exposed to high light at noon. The Fv/Fm of all the plants recovered rapidly under low afternoon light, indicating that photoinhibition is a regulated and protective process of energy dissipation. In this study, the Fv/Fm value of the leaves of three ephemeral plants was found to decrease sharply with the increase of external temperature under sunny conditions in the summer. The Fv/Fm of leaves of the three fully dark acclimated plants decreased with the extension of treatment time under the artificial stress condition of 35°C ± 1°C, which all indicated that the light suppression occurred in the transient plants under high temperature stress. The fact that Fv/Fm can basically recover in the afternoon or at low temperature indicates that the leaves of the three ephemeral plants have robust mechanisms for photoprotection. However, the Fv/Fm values of the three ephemeral plants treated with DTT and SM were significantly lower than those of the CK group after high temperature stress (without inhibitor treatment), and the Fv/Fv values of the SM and DTT groups were significantly lower than those of the CK group after low temperature recovery, which was consistent with the results of research on broccoli and bamboo among others [6,35]. These results indicated that lutein cycling and D1 protein turnover play an important role in defending against the destruction of photosystem of three ephemeral plants under high temperature stress.
It has been shown that most plants can dissipate excessive excitation energy through the lutein cycle to protect them from strong light damage [48]. Rintamaki et al. through using inhibitors proved that the lightdependent D1 protein turnover is the mechanism of PSII defense against photodestruction [49]. In this study, the Fv/Fm values of the leaves of the three ephemeral plants decreased sharply when the D1 protein synthesis inhibitor SM was used at 35°C ± 1°C, and turnover of the D1 protein was blocked. After 4 h of stress, the Fv/Fm values of Erodium oxyrrhynchum, Senecio subdentatus and Heliotropium acutiflorum decreased by 69.34%, 46.36% and 29.04%, respectively. However, after inhibition of the lutein cycle with DTT, the Fv/Fm values of E. oxyrrhynchum, S. subdentatus and H. acutiflorum were 47.66%, 34.24% and 67.45% lower than those before stress, respectively. The Fv/Fm values of the E. oxyrrhynchum, S. subdentatus and H. acutiflorum CK groups were basically restored to the level before high temperature stress after 8 h of low temperature recovery. The Fv/Fm values of E. oxyrrhynchum in the SM group recovered to 72.41%, 72.77% and 85.79%, respectively. The Fv/Fm values of the three ephemeral plants in the DTT group recovered to 80.60%, 82.92% and 67.33%, respectively, after 8 h of low temperature. These results indicated that the inhibition of D1 protein turnover and the lutein cycle could enhance the damage of PSII reaction center in the leaves of the three ephemeral plants. E. oxyrrhynchum and S. subdentatus were affected more strongly by SM than by DTT. However, SM was less effective on H. acutiflorum than DTT, indicating that the D1 protein turnover was more effective at protecting E. oxyrrhynchum and S. subdentatus from heat stress than the lutein cycle. However, the role of lutein cycle in the response of H. acutiflorum to heat damage was greater than that of the D1 protein turnover.
PSII antenna pigments absorb light energy in three ways, including the energy used by PSII photochemical reactions Y(II), PSII regulatory energy dissipation Y(NPQ) and non-regulatory energy dissipation Y(NO) [36]. Analysis of the distribution of absorbed light energy will help to understand the strategy used by plant leaves to protect themselves against absorbed light energy [50]. A high Y(NPQ) indicates that the leaves have a highly regulated physiological metabolism, including heat dissipation and other methods used to dissipate excess light energy [36]. The results showed that Y(II) was significantly reduced and Y(NPQ) was significantly increased under high temperature stress, indicating that ephemeral plant leaves increased the proportion of heat dissipated energy to resist the accumulation of excess excitation energy during high temperature stress [51]. In addition, the Y(II) of the three ephemeral plants in the SM and DTT groups was significantly lower than that in the CK group, indicating that the inhibitor treatment significantly reduced the Y(II) value of the three ephemeral plants. The Y(NPQ) value gradually increased during the process of high temperature stress, but there was a low range of change. After 4 h of stress, the Y(NPQ) values of the Erodium oxyrrhynchum treatment groups were DTT > SM > CK, and the Y(NPQ) values of the Senecio subdentatus treatment groups were SM > CK > DTT. Heliotropium acutiflorum had Y(NPQ) values of SM > DTT > CK. These results indicated that treatment with inhibitors had different effects on the ability of the three ephemeral plants to dissipate heat. In E. oxyrrhynchum and H. acutiflorum, treatment with DTT and SM increased the ability of leaf PSII to dissipate heat under high temperature stress. In S. subdentatus, the SM treatment increased the ability of leaves to dissipate heat under high temperature stress, while the DTT treatment decreased the ability of leaves to dissipate heat under high temperature stress. A high Y(NO) value indicates that the photochemical reaction and protective regulatory mechanism of PSII do not play a role, and excessive excitation energy will cause PSII to undergo photodestruction. In this study, the Y(NO) values in each treatment group of the three ephemeral plants increased gradually with the extension of time of high temperature stress. After 4 h of stress, the Y(NO) values in each treatment group of E. oxyrrhynchum were as follows: SM > DTT > CK. S. subdentatus and H. acutiflorum had Y(NO) values of DTT > SM > CK. These results indicated that the SM and DTT treatments significantly increased the photoinhibition of the three ephemeral plants under high temperature stress.
Y(NA) represents the quantum yield of non-photochemical energy dissipation caused by restriction of the PSI electron acceptor side, which is one of the indicators of PSI photoinhibition [52]. The results showed that the Y(NA) values of the three ephemeral plants increased gradually after high temperature stress, and after 4 h of stress, the Y(NA) values of Erodium oxyrrhynchum, Senecio subdentatus and Heliotropium acutiflorum in each treatment group were as follows: SM > DTT > CK, indicating that high temperature stress also inhibited the activity of PSI in the leaves of three ephemeral plants to some extent, and the treatment with SM and DTT aggravated the degree of inhibition of PSI in the three ephemeral plants. The Fm value, which reflects the electron transport status of PSII, decreased significantly after high temperature stress and decreased further after treatment with the inhibitors SM and DTT, indicating that high temperature stress caused more damage to the acceptor side of PSII reaction center than the donor side of PSII reaction center. The introduction of inhibitors SM and DTT aggravated the damage to PSII reaction center of the three ephemeral plants [53]. After high temperature stress, the Y(I), Y(ND) and Y(NA) of the three treatment groups changed, but there was only a small range of change, indicating that high temperature stress caused severe photoinhibition of PSII and had some degree of influence on PSI of the three ephemeral plants.
All the leaves of the three ephemeral plants have their own highly evolved mechanism to defend against photodestruction. The inhibition of D1 protein turnover and xanthophyll cycle can damage the photosystem of the leaves of the three ephemeral plants to some extent at high temperature. Damage from the SM treatment of Erodium oxyrrhynchum and Senecio subdentatus was greater than that of the DTT treatment, while the damage to Heliotropium acutiflorum was less than that of the DTT treatment. The protective effect of D1 protein turnover in E. oxyrrhynchum and S. subdentatus against heat stress was greater than that of lutein cycle, and the role of lutein cycle was greater than that of D1 protein turnover in H. acutiflorum against heat stress.
Funding Statement: This work was supported by the Open Foundation of the State Key Laboratory of Desert and Oasis Ecology (KH0054).
Conflicts of Interest: The authors declare that they have no conflicts of interest to report regarding this study.
References
1. Suzuki, N., Rivero, R. M., Shulaev, V., Blumwald, E., Mittler, R. (2014). Abiotic and biotic stress combinations. The New Phytologist, 203(1), 32–43. https://doi.org/10.1111/nph.12797 [Google Scholar] [PubMed] [CrossRef]
2. Boyer, J. S. (1982). Plant productivity and environment. Science, 218(4571), 443–448. https://doi.org/10.1126/science.218.4571.443 [Google Scholar] [PubMed] [CrossRef]
3. Allakhverdiev, S. I., Kreslavski, V. D., Klimov, V. V., Los, D. A., Carpentier, R. et al. (2008). Heat stress: An overview of molecular responses in photosynthesis. Photosynthesis Research, 98(1–3), 541–550. https://doi.org/10.1007/s11120-008-9331-0 [Google Scholar] [PubMed] [CrossRef]
4. Nishiyama, Y., Allakhverdiev, S. I., Murata, N. (2011). Protein synthesis is the primary target of reactive oxygen species in the photoinhibition of photosystem II. Physiologia Plantarum, 142(1), 35–46. https://doi.org/10.1111/j.1399-3054.2011.01457.x [Google Scholar] [PubMed] [CrossRef]
5. Ge, J. L., Shi, L. Gu, W., B., Tang, Y. D., Zhang, J. Z. et al. (2007). Photosynthetic characteristics and the regulation of photosystemII function in salt-stressed sweet sorghum seedlings. Acta Agronomica Sinica, 33(8), 1272–1278. [Google Scholar]
6. Jie, M. H., Zhang, J. S., Yu, J. H. (2009). The role of D1 protein turnover and lutein cycle in the defense of bright light damage in broccoli leaves. China Agriculture Science, 42(5), 1582–1589. [Google Scholar]
7. Zhao, L., Zheng, X. Y., Sun, G. Y. (2010). Adaptability of morphological structure and photosynthetic characteristics of low-light mulberry seedlings to light intensity. Journal of Economic Forest Research, 28(3), 6–15. [Google Scholar]
8. Ebbert, V., AdamsIII, W., Mattoo, A., Sokolenko, A., Demming-Adams, B. (2005). Up-regulation of a photosystem II core protein phosphatase inhibitor and sustained D1 phosphorylation in zeaxanthin-retaining, photoinhibited needles of overwintering Douglas fir. Plant, Cell and Environment, 28(2), 232–240. https://doi.org/10.1111/j.1365-3040.2004.01267.x [Google Scholar] [CrossRef]
9. Pieters, A. J., Tezara, W., Herrera, A. (2003). Operation of the xanthophyll cycle and degradation of D1 protein in the inducible CAM plant, Talinum triangulare, under water deficit. Annals of Botany, 92(3), 393–399. https://doi.org/10.1093/aob/mcg153 [Google Scholar] [PubMed] [CrossRef]
10. Guo, Z. G., Wang, M. X., Cui, L., Han, B. Y. (2016). Research progress on plant photodestruction defense mechanism. Subtropical Plant Science, 45(3), 5–14. [Google Scholar]
11. Xu, J. X., Zhou, H. F., Qiu, C. H., Guo, Y. P., Ji, W. W. (2011). Studies on the relationship among photosynthetic apparatus operation, xanthophylls cycle and D1 protein turnover in satsuma mandarin leaves under high temperature and strong light. Acta Horticultural Sinica, 38(2), 10–18. [Google Scholar]
12. Zhang, H. H., Zhang, X. L., Li, X., Xu, N., Sun, G. Y. (2013). Effects of D1 protein turnover and lutein cycle on PSII in mulberry leaves under salt stress. Forestry Science, 49(1), 99–106. [Google Scholar]
13. Walters, R. G. (2005). Towards an understanding of photosynthetic acclimation. Journal of Experimental Botany, 56(411), 435–447. https://doi.org/10.1093/jxb/eri060 [Google Scholar] [PubMed] [CrossRef]
14. Jiang, C. D., Gao, H. Y., Zou, Q. (2002). D1 protein turnover and its regulation of energy dissipation. Plant Physiology Journal, 38(3), 207–212. [Google Scholar]
15. Demmig-Adams, B., Adams, W. W., Heber, U., Neimanis, S., Winter, K. et al. (1990). Inhibition of zeaxanthin formation and of rapid changes in radiationless energy dissipation by dithiothreitol in spinach leaves and chloroplasts. Plant Physiology, 92(2), 293–301. https://doi.org/10.1104/pp.92.2.293 [Google Scholar] [PubMed] [CrossRef]
16. Aro, E. M., McCaffery, S., Anderson, J. M. (1994). Recovery from photoinhibition in peas (Pisum sativum L.) acclimated to varying growth irradiances (Role of D1 Protein Turnover). Plant Physiology, 104(3), 1033–1041. https://doi.org/10.1104/pp.104.3.1033 [Google Scholar] [PubMed] [CrossRef]
17. Guan, X. Q., Li, D. Q., Shu, H. R. (2004). Photoprotective function of photorespirationin grapevine under drought stress. Shandong Agricultural University, Shandong, China. [Google Scholar]
18. Wei, A. L., Wang, Z. M. (2004). Research progress on photosuppression and photodestruction of PSII. in higher plants. Northwestern Botanical Journal, 24(7), 6–14. [Google Scholar]
19. Dong, F., Chen, Y. Z., Jiang, Y. M. (1999). Plant lutein cycle and nonradiative energy dissipation. Plant Physiology Journal, 35(2), 141–144. [Google Scholar]
20. Sapozhnikov, D. I., Krasovskaya, T. A., Maevskaya, A. N. (1957). Change in the interrelationship of the basic carotenoids of the plastids of green leaves under the action of light. Doklady Akademii Nauk SSSR, 113(2), 465–467. [Google Scholar]
21. Yamamoto, H. Y., Nakayama, T. O., Chichester, C. O. (1962). Studies on the light and dark interconversions of leaf xanthophylls. Archives of Biochemistry and Biophysics, 97(1), 168–173. https://doi.org/10.1016/0003-9861(62)90060-7 [Google Scholar] [PubMed] [CrossRef]
22. Xu, D. Q. (1988). Photosynthetic efficiency. Plant Physiology Communications, 37(5), 1–7. [Google Scholar]
23. Demmig, B., Winter, K., Krüger, A., Czygan, F. C. (1987). Photoinhibition and zeaxanthin formation in intact leaves: A possible role of the xanthophyll cycle in the dissipation of excess light energy. Plant Physiology, 84(2), 218–224. https://doi.org/10.1104/pp.84.2.218 [Google Scholar] [PubMed] [CrossRef]
24. Zheng, L. P. (2009). Low carbon economy: The inevitable choice of scientific development. Jiangnan Forum, 15(8), 3–11. [Google Scholar]
25. Peng, M., He, H., Wang, Z., Li, G., Lv, X. et al. (2022). Responses and comprehensive evaluation of growth characteristics of ephemeral plants in the desert-oasis ecotone to soil types. Journal of Environmental Management, 316(3), 115–288. https://doi.org/10.1016/j.jenvman.2022.115288 [Google Scholar] [PubMed] [CrossRef]
26. Mao, Z. M., Zhang, D. M. (1994). The conspectus of ephemeral flora in Northern Xinjiang. Arid Zone Research, 11(3), 1–26. [Google Scholar]
27. Wang, X. Q., Jiang, J., Lei J. Q., Zhang, W. M., Qian, Y. B. et al. (2003). Distribution of short-lived plants in the Gurbantunggut Desert and its significance of sand surface stability. Acta Geographica Sinica, 58(4), 8–18. [Google Scholar]
28. Zhang, L. Y., Cheng, C. D. (2002). On the general characteristics of plant diversity of Gurbantunggut Sandy Desert. Acta Ecologica Sinica, 22(11), 1923–1932. [Google Scholar]
29. Yang, G. P., Lai, X. H. (2020). Research progress on cold resistance of spring ephemeral. Modern Agricultural Science and Technology, 13(2), 167–168. [Google Scholar]
30. Mao, Z. M. (1992). Floristic characteristics of spring ephemeral. Arid Zone Research, 9(1), 2–11. [Google Scholar]
31. Si, W., Zhuang, D. L., Zhang, Y. W., Zhao, J. M. (2020). Ecological adaptability of ephemeral plants and evolutionary significance. Guangdong Sericulture, 54(10), 13–17. [Google Scholar]
32. Jiang, C. D., Gao, H. Y., Zou, Q., Jiang, G. M. (2003). Disequilibrium of excitation energy distribution between two photosystems in soybean leaves induced by dithiothreitol treatment. Plant Physiology and Molecular Biology Bulletin, 39(6), 221–226. [Google Scholar]
33. Dao, J. C., Yuan, G. H., Qi, Z. (2003). Effects of streptomycin treatment on chlorophyll fluorescence parameters and xanthophyll de-epoxidation level in maize leaves. Acta Photophysiologica Sinica, 29(3), 221–226. [Google Scholar]
34. Hong, S. S., Xu, D. Q. (1997). Differences in response of leaf fluorescence parameters to high light in wheat and soybean. Chinese Science Bulletin, 42, 753–756. [Google Scholar]
35. Lou, Y. F., Gao, Z. M. (2020). The role of lutein cycle and D1 protein turnover in photoprotection of leaves of P. edulis. Plant Science Journal, 38(1), 9–20. [Google Scholar]
36. Xing, H., Hao, G. M., Chen, Z. W., Sun, Y. J., Qu, H. (2017). Effects of ozone stress on photosystem activity of grape leaves and defense of D1 protein turnover. Plant Physiology Journal, 53(10), 8–18. [Google Scholar]
37. Varadi, G., Darko, E., PoLos, E., Szigeti, Z., Lehoczki, E. (1994). Xanthophyll cycle patterns and in vivo photoinhibition in herbicide-resistant biotypes of conyza canadensis. Journal of Plant Physiology, 144(6), 669–674. https://doi.org/10.1016/S0176-1617(11)80658-9 [Google Scholar] [CrossRef]
38. Feng, X. L., Liu, R., Li, C. J., Wang, Y. G., Kong, L. et al. (2022). Branches of T. Photosynthesis of branches of T. and its main influencing factors. Chinese Journal of Applied Ecology, 33(2), 344–352. [Google Scholar]
39. Hu, S. J., Zhen, B. W. (2021). Energy balance and evapotranspiration characteristics of Haloxylon ammodendron community at the southern edge of the Gurbantunggut desert. Chinese Journal of Ecology, 41(1), 92–100. [Google Scholar]
40. Feng, X. L., Liu, R., Ma, J., Xu, Z., Wang, Y. G. et al. (2021). Photosynthesis of the branches of the Batuantunggut Desert and its influencing factors. Acta Ecologica Sinica, 41(24), 9784–9795. [Google Scholar]
41. Kramer, D. M., Johnson, G., Kiirats, O., Edwards, G. E. (2004). New fluorescence parameters for the determination of QA redox state and excitation energy fluxes. Photosynthesis Research, 79(2), 209–218. https://doi.org/10.1023/B:PRES.0000015391.99477.0d [Google Scholar] [PubMed] [CrossRef]
42. Liu, J. X., Sun, Z. Y., Gou, P., Qian, Y. Q., Gu, G. S. et al. (2012). Response of photosynthetic physiology of perennial ryegrass (Loliumperenne) to Cd2+ stress. Acta Prataculturae Sinica, 21(3), 191–197. [Google Scholar]
43. Wang, Y. B., Xu, S. P., Ma, J., Cui, B. (2018). Effects of heat and drought stress on the photosynthetic characteristics and chlorophyll fluorescence parameters of Bletillastriata. Journal of Henan Agricultural University, 52(2), 199–205. [Google Scholar]
44. Hong, S. S., Xu, D. Q. (1999). Light-induced increase in initial chlorophyll fluorescence Fo level and the reversible inactivation of PS II reaction centers in soybean leaves. Photosynthesis Research, 61(3), 269–280. https://doi.org/10.1023/A:1006357203466 [Google Scholar] [CrossRef]
45. Li, C. F., Dong, S. T., Liu, R. X., Ren, H., Ding, Z. S. et al. (2019). Diurnal variation of gas exchange, chlorophyll fluorescence, and photosynthetic response of six parental lines of maize released in three eras. Journal of Integrative Agriculture, 18(12), 2732–2741. https://doi.org/10.1016/S2095-3119(19)62693-6 [Google Scholar] [CrossRef]
46. Wang, Q., Zhang, Q. D., Zhu, X. G., Lu, G. M., Kuang, T. Y. et al. (2002). PSII photochemistry and xanthophyll cycle in two superhigh-yield rice hybrids, Liangyoupeijiu and Hua-an 3 during photoinhibition and subsequent restoration. Journal of Integrative Plant Biology, 44, 1297–1302. [Google Scholar]
47. Su, P., Liu, X. (2005). Photosynthetic characteristics of linze jujube in conditions of high temperature and irradiation. Scientia Horticulturae, 104(3), 339–350. https://doi.org/10.1016/j.scienta.2004.08.012 [Google Scholar] [CrossRef]
48. Lin, R. C., Xu, C. C., Li, l B., Kuang, T. Y. (2002). Xanthophyll cycle and its molecular mechanism in photoprotection. Acta Botanica Sinica, 44(4), 379–383. [Google Scholar]
49. Rintamaki, E., Salo, R., Aro, E. M. (1994). Rapid turnover of the D1 reaction-center protein of photosystem II as a protection mechanism against photoinhibition in a moss, Ceratodon purpureus (Hedw.) Brid. Planta, 193(4), 520–529. https://doi.org/10.1007/BF02411557 [Google Scholar] [CrossRef]
50. Hou, X. F., Shang, L. T., Zhang, L. C., Guo, S. M. (2021). Comparative study on chlorophyll fluorescence parameters of Rubus chingiin different habitats. South China Forestry Science, 49(3), 33–36. [Google Scholar]
51. Baker, N. R. (2008). Chlorophyll fluorescence: A probe of photosynthesis in vivo. Annual Review of Plant Biology, 59(1), 89–113. https://doi.org/10.1146/annurev.arplant.59.032607.092759 [Google Scholar] [PubMed] [CrossRef]
52. Huang, W., Yang, Y. J., Zhang, J. L., Hu, H., Zhang, S. B. (2017). Superoxide generated in the chloroplast stroma causes photoinhibition of photosystem I in the shade-establishing tree species Psychotria henryi. Photosynthesis Research, 132(3), 293–303. https://doi.org/10.1007/s11120-017-0389-4 [Google Scholar] [PubMed] [CrossRef]
53. Lu, C., Zhang, J. (1999). Effects of water stress on photosystem II photochemistry and its thermostability in wheat plants. Journal of Experimental Botany, 50(336), 1199–1206. https://doi.org/10.1093/jxb/50.336.1199 [Google Scholar] [CrossRef]
Cite This Article
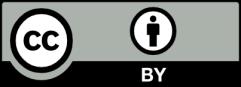