Open Access
ARTICLE
Heat exposure promotes apoptosis and pyroptosis in Sertoli cells
School of Life Science, Bengbu Medical College, Bengbu, 233030, China
* Corresponding Author: MENG LIANG. Email:
BIOCELL 2023, 47(1), 155-164. https://doi.org/10.32604/biocell.2023.024657
Received 05 June 2022; Accepted 10 August 2022; Issue published 26 September 2022
Abstract
Heat stress is an important influence on the male reproductive organs. Therefore, the effects of heat stress on genes or pathways related to the reproductive system of male mice were experimentally explored in this paper to further determine the effects of heat stimulation on mammals. Herein, models of heat-exposed mouse testicular tissue and heat-excited cells were successfully established. Many scorched vesicles were found after heat excitation of testis supporting cells, testicular mesenchymal (TM4) cells. Western blot, in situ terminal deoxynucleotide transferase dUTP Nick end labeling (TUNEL) and transmission electron microscopy showed that membrane rupture, mitochondrial damage and autophagic vesicles occurred in TM4 cells after thermal excitation. The N-segment fragment of the associated protein shear was increased, and the TUNEL result was positive. In conclusion, thermal excitation induced apoptosis and scorch death in TM4 cells. Thus, the Hippo pathway and apoptosis-related pathway were significantly enriched after heat stimulation in mouse testis, and the scorch death effect in TM4 cells was induced by heat excitation.Keywords
The number of patients affected by infertility has been steadily increasing over the years, and infertility has become one of the major health challenges in modern society (Yu et al., 2021a). An analysis of male reproductive status worldwide from 1980 to 2015 and from 1981 to 2013 by Sengupta et al. (2017) and Levine et al. (2017), respectively showed that male sperm density has decreased by an average of 57% and sperm count decreased by 52.4%. The abundance of material goods also brings a greater possibility of infertility, as more than one in ten couples of marriage age worldwide are unable to have offspring; nearly 50% of the reasons behind this phenomenon are related to men (Kobori, 2019; Moye et al., 2019). The normal testicular function has often been strongly related to temperature and the normal degree of testicular function is an important basis for reproductive development. The effects of heat stress on reproductive organs, such as the testes and ovaries, currently stop at a relatively superficial level, and many molecular mechanisms are still waiting to be discovered. If the scrotum is exposed to too much cold or heat, the production of viable sperm may be blocked, and in severe cases, the male individual may suffer adverse reactions. The phenomenon of heat shock has been reported many times in past studies. We know that heat shock proteins (HSPs) are instrumental when cells are subjected to heat effects, and that activation of heat shock factor (HSF) plays an important role in this process. HSPs are proteins that are expressed in accordance with different external environmental changes. In the face of an unfavorable external environment, intracellular proteins form a correct fold with the assistance of HSPs to escape from the damage, while HSPs are required for spermatogenesis. The effect of germ cells (GCs) on spermatogenesis has been widely reported, but relatively little research has been done on somatic cells, such as Sertoli cells (SCs) and Leydig cells, which are particularly important for spermatogenesis as a major component of blood-testis barrier (BTB). Hippo pathway expression is closely related with the growth and development of biological individuals. Among the known reports of the Hippo pathway, we are concerned that when the pathway is inactive, its downstream serine/threonine kinases (AKT) and mitogen-activated protein (MAPK) are activated by phosphorylation, and both phosphorylations act together on large tumor suppressor ½ (LATS1/2), causing it to be phosphorylated as well. Because YAP/TAZ phosphorylation does not enter the nucleus, Vestigial-like family member 4 (VGLL4) binds to the transcriptional enhanced associate domain (TEAD) and shows transcriptional inhibition. In contrast, when the Hippo pathway is activated, YAP/TAZ dephosphorylates and enters the nucleus, occupying the position of VGLL4 originally bound to TEAD, thereby inducing the expression of genes that promote cell proliferation and inhibit apoptosis. A number of differential genes were found in this paper based on heat excitation sequencing results and the Hippo pathway which was associated with cell growth at all times was identified. These two were combined then to exhaustively investigate why heat excitation could bring a range of phenotypes to cells. Therefore, we chose to establish a TM4-supported cellular heat excitation model for validation. Previous studies have shown that testicular spermatogenic cells in mice exposed to heat are enlarged and vacuolated, and spermatozoa in the epididymis also have abnormal morphology (Yu et al., 2021b).
Therefore, in this study, an in vivo heat-stimulated mouse model and an in vitro heat-stimulated TM4 cell model were used to carry out experiments, which were validated by a series of experiments, and also, the markedly enriched Hippo signaling route was detected. The Hippo pathway was implicated in the modulation of apoptosis and cell scorching. The apoptosis and cell scorching-related indicators were detected, and it was found that heat stress may activate the Hippo signaling pathway to induce apoptosis and cell scorching in support cells of TM4.
All SPF-grade male ICR mice used in this experiment were purchased from Skbex Biotechnology Co., Ltd. (Anyang, Henan, China). The experimental mice were categorized into normal and heat-stimulated groups, with three mice within each experimental treatment group; the experiment was repeated three times. All mice were fed normally until two months for subsequent experiments. The experimental mice were kept on a normal diet in an animal room environment of 20°C–26°C, with a maximum temperature difference of less than or equal to 4°C, relative humidity of 40%–70%, ventilation greater than or equal to 15 times/h, and 12 h light and 12 h dark photoperiod. Permission for this study was obtained from the Laboratory Animal Management and Ethics Committee of Bengbu Medical College.
TM4 cell lines were purchased from Wuhan Procell Life Sciences Company (Procell, Wuhan, China). The TM4 cells were plated in Dulbecco’s modified Eagle medium/nutrient mixture F-12 medium (Gibco, New York, USA) complemented with 5% horse serum (Procell), 2.5% fetal bovine serum, and 1% penicillin-streptomycin (Beyotime, Shanghai, China). The cells were acclimated in a cell culture incubator set at 37°C and 5% CO2.
Testicular heat exposure model in mice
The scrotal heat excitation experiments were performed on mice using the hot water bath method as follws: first, we configured 0.01 mL/g of 5% chloral hydrate (Macklin, Shanghai, China) and turned on the 43°C and 33°C water baths for 1 h. The body weight of mice was measured and male ICR mice of similar body size were categorized into two groups to prepare for the heat stress experiment; mice were anesthetized sequentially according to the measured body weight at an injection volume of 10 µL/g 5% chloral hydrate. The scrotum of anesthetized mice was exposed to a water bath for 25 min at 33°C for the control group, 43°C for the high-temperature group as per the earlier published method (Akintayo et al., 2020), and 43°C for the hyperthermia group. The mice were sacrificed 24 h after testicular heat exposure, and finally, the testes and epididymis were isolated from the mice for histological examination and RNA isolation.
Terminal deoxynucleotidyl transferase dUTP nick-end-labeling staining
Paraffin sections prepared from the mouse testes obtained in Step 1 were used for terminal deoxynucleotidyl transferase dUTP nick-end-labeling (TUNEL) experiments. First, the sections were dewaxed by soaking for 10 min each in xylene I (Macklin) and xylene II, and then hydrated with gradient ethanol (100% × 5 min, 100% × 3 min, 9% × 3 min, 85% × 3 min, 70% × 3 min, 50% × 3 min). The slides were washed once with 0.85% NaCl solution and again with phosphate buffer saline (PBS; Procell) solution, and the tissue was fixed on the slides using 4% paraformaldehyde (Macklin) for 15 min. After fixing, the slides were washed twice with PBS for 5, and then 100 µL of 20 μg/mL proteinase K (Beyotime) was added to each slide near the tissue for 15 min at room temperature. Following the addition of proteinase K, the tissue was washed twice with PBS for 5 min, and the TUNEL reaction mix was prepared strictly according to the ratio of the instructions (Beyotime). A total of 50 μL TUNEL reaction solution was added dropwise to each sample, and then the sections were placed into the dark box covered with tin foil and incubated at 37°C for 60 min. After incubation, the samples were washed thrice with PBS for 5 min, and 50 μL of DAPI (Beyotime) staining solution was added dropwise to the sample and allowed to stain for 1 min. After staining, the samples were washed twice with PBS for 5 min; then, the slices were sealed with anti-fluorescence quenching sealing solution and observed under a fluorescence microscope (Zeiss, Oberkochen, Germany).
Cellular heat stimulation model
To further investigate the effect of thermal stimulation on spermatogenesis, the mouse TM4 cells were used to construct an in vitro thermal stimulation model. A thermometer was used to preset the temperature of the water bath to 43°C, and a 15 mL centrifuge tube was used to place part of the medium on the ultra-clean table, and the rest was preheated in the water bath. After the temperature of the water bath got stabilized, the cultured TM4 cells were taken out of the cell culture incubator, and the pre-warmed medium was taken out of the water bath. The tubes containing the preheated medium were removed from the water bath and sterilized by light spraying with alcohol, and placed on the ultra-clean bench. The medium in the cell culture dish was aspirated and discarded, and the pre-warmed medium was added. After successful addition, the cell culture dishes were sealed with a sealing film, placed in a 43°C water bath, and timed. TM4 cells were removed from the water bath at 10 min, 20 min, and 30 min after heat excitation, and the TM4 cell culture dishes completed by heat shock were sterilized by light spraying with alcohol, then the sealing film was removed, marked, and placed in an incubator at 37°C. After 12 h, cells were harvested for subsequent experiments.
RNA extraction and reverse transcription
Total RNA was extracted from testicular tissue using Trizol (Invitrogen, California, USA) and the entire experiment was performed strictly in an enzyme-free environment by referring to the manufacturer’s instructions. Testicular samples were lysed in enzyme-free microfuge tubes after heat excitation, stripped of the white membrane, and 1 mL of Trizol and 2–3 small magnetic beads were added to each tube and homogenized using a grinder; the lysed samples were left at ambient temperature for 10 min. Then, 0.2 mL of chloroform solution (Macklin) was added to the samples, shaken, mixed for 15 s, and left at the ambient temperature for 3 min. These samples were centrifuged at 4°C and 12,000*g (Eppendorf, Hamburger, Germany) for 15 min. After centrifugation, 450 µL of the uppermost supernatant was aspirated into a new enzyme-free EP tube and labeled, and an equal volume of isopropanol (Macklin) was added to the EP tube, vortexed, mixed well, and then left at room temperature for 10 min. The samples were centrifuged at 4°C and 12,000*g for 10 min, and then the supernatant was discarded carefully to prevent aspiration to the bottom RNA. Ethanol (75%) was prepared using DEPC water (Beyotime) and used to wash the precipitated RNA. The solutions were centrifuged again at 4°C and 7,500*g for 5 min, and then the supernatant was discarded as much as possible (avoiding aspiration to the bottom RNA). The samples were allowed to dry at the ambient temperature for 3 min. The RNA was dissolved using 50 μL DEPC water and transferred to a new EP tube. The concentration was measured, and recorded using an enzyme-labeled instrument (Bio Tek, Vermont, USA). Then the samples were frozen at −80°C. The complementary DNA (cDNA) was obtained from total RNA by reverse transcription (15 min at 37°C, 5 s at 85°C) using a reverse transcription kit (Accurate, Changsha, China).
Real-time quantitative polymerase chain reaction (RT-qPCR)
The efficiency of the model after heat excitation of TM4 cells was tested by analyzing the expression level of Hspa1a, a member of the HSP70 family, by RT-qPCR (Applied Biosystems, Milwaukee, USA). Briefly, cDNA was generated from 500 ng of gross RNA using a reverse transcription kit. Subsequently, RT-qPCR kits (Accurate, Changsha, China) were performed to identify the targeted gene expression (Suppl. Table S1); the β-actin primers were designed with reference to the previous report (Hu et al., 2019; Yao et al., 2014). The expression was normalized to ACTB (β-actin) using the comparative 2-ΔΔ Ct method for the expression level of the target gene (Yao et al., 2014).
Total proteins were extracted from TM4 cells after heat stress by RIPA lysate (Millipore, Billerica, MA, USA) containing a 1% cocktail (Roche, Indianapolis, USA). The expression levels of heat-shock protein 70 (HSP70), gasdermin E (GSDME), actin beta (ACTB), and Hippo pathway-related proteins were finally detected as follows: the amount of loading for electrophoresis was determined using bicinchoninic acid quantification; a 10% sodium dodecyl sulfate-polyacrylamide gel (Beyotime) was configured and placed into the electrophoresis tank at the end of the gel and electrophoresis buffer. Then the comb was pulled, and the sample for electrophoresis was spotted (90 V for 20 min and then at 120 V for 70 min). After electrophoresis, the membrane was transferred using the wet transfer method (300 mA 90 min) and then sliced according to the marker. The membranes with the target protein bands were placed in the incubation box and closed for 2 h with the appropriate amount of closure solution. After the closure was finished, the membranes were washed three times with the washing solution and incubated overnight at 4°C in the refrigerator with the corresponding primary antibody, including the Hippo Signaling Antibody Sampler Kit (YAP/TAZ primary antibody, LATS1 primary antibody, MST1 primary antibody, MST2 primary antibody, phospho-YAP [Ser127] primary antibody, and MOB1 primary antibody; Cell Signaling Technology, Bostons, USA), HSP70 primary antibody (ABclonal, Wuhan, China), GSDME primary antibody (Abcam, Cambridge, UK), and ACTB primary antibody (ABclonal, Wuhan, China). The membrane was incubated overnight and placed on a shaker for 20 min, and then the primary antibody was recovered and washed three times using membrane wash solution. The secondary antibody of rabbit origin (Cell Signaling Technology) was added and incubated for 1 h at ambient temperature. After incubation, the secondary antibody was recovered, and the membrane was washed four times with the membrane wash solution. After the wash, the developing solution was prepared and the image was developed using a gel imager (Bio-Rad Corporation, California, USA) and saved.
Each experiment mentioned above was repeated at least thrice. Student’s t-test was used to analyze the data. The experimental data were represented as average ± standard error, and a P-value < 0.05 indicated a statistically meaningful relationship between the groups. P < 0.01 indicates a statistically significant difference between groups. P < 0.001 indicates an extremely highly pronounced statistical variance between groups.
Establishment of a mouse testicular heat exposure model
The study showed that nearly 2,000 mRNAs were upregulated and nearly 1,000 mRNAs were down-regulated, indicating that heat stress must cause some degree of damage to the mouse reproductive system. Thus, in this study, we re-established a mouse model of heat stress and enriched the genes affected by heat stress from known studies into the relevant pathways. From the cellular model of heat stress, we investigated some genetic changes that occur within individual mice and their effects on life activities from a molecular biology perspective. To explore the effects of heat exposure on the testis, mice were first anesthetized after scrotal heat exposure experiments, with the control group exposed to 33°C for 25 min and the high-temperature group exposed to 43°C for 25 min. After 24 h, TUNEL staining was performed, and mouse testes showed many apoptotic signals in the walls of the varicose tubules after heat treatment (Fig. 1), indicating the successful establishment of a testicular heat excitation model and that the heat does cause some harm to mouse reproductive system.
Figure 1: Terminal deoxynucleotidyl transferase dUTP nick-end-labeling (TUNEL) staining after heat exposure of mouse testes shows massive apoptosis. Mouse testes were kept in a water bath at 33°C (control group) or 43°C (high-temperature group) for 25 min. After 24 h, paraffin-embedded sections of the testes were stained for TUNEL. As shown in the figure, a large number of cells in the varicose seminal tubules underwent apoptosis after heat excitation. Red represents TUNEL-positive apoptotic cells, and blue represents 4′,6-diamidino-2-phenylindole (DAPI)-labeled nuclei.
Heat stress induces activation of the Hippo pathway
In previous studies on heat excitation on reproduction, our sequencing results showed that a large proportion of genes with significantly different expressions were enriched to the Hippo pathway, which provides a new insertion point for us to further investigate the molecular mechanism of heat excitation (Hu et al., 2021). In the classical pathway, it is noticed that the Hippo pathway affects the growth, proliferation, and development of cells. So, it is speculated in the present study that once the Hippo pathway is disturbed, the cells malfunction would be present in the development, acquisition of specific functions, formation of organ development, and tissue constancy, which is one of the culprits of tumors. YAP and TAZ are transcriptional co-activators, the main downstream activators of the Hippo pathway. In our preliminary analysis of sequencing results, it has been found many differentially expressed genes get enriched in the Hippo pathway after heat excitation, suggesting that the Hippo pathway is involved in transcriptional regulation after heat excitation. In addition, in tumor cells, heat-treated cells have been reported to induce strong and rapid YAP dephosphorylation and activation; hence, this study is used to investigate whether heat stress can induce Hippo pathway activation and thus regulate downstream genes in mouse support cells. A TM4 cell heat stress model was established, and RT-qPCR results showed that the level of HSPA 1a RNA from the heat shock protein family in TM4 cells was significantly upregulated after 12 h of heat stress (Fig. 2a). Western blot experiments showed a significant increase in the level of HSP70 protein (Fig. 2b), indicating that a TM4 heat stress cell model was successfully established. Cells were also collected for western blotting experiments immediately after heat excitation of TM4 cells and it was found that the levels of LATS1 kinase activity, MOB-1, YAP/TAZ proteins decreased, and the phosphorylation level of YAP decreased (Figs. 3a and 3b). Immunofluorescence experiments demonstrated that YAP/TAZ clearly had an aggregation toward the nucleus after heat treatment (Fig. 4). Since YAP and TAZ are transcriptional co-activators, it can be speculated that after heat excitation, the dephosphorylation of YAP leads to the activation of the Hippo pathway, which in turn regulates the transcriptome changes after heat excitation.
Figure 2: Increase in mRNA and protein levels of TM4 cells after heat stress. (a) Heat-stimulated TM4 cells cause significant upregulation of Hspa1a RNA levels in the heat shock protein family. After heat stimulation for 20 min and incubation for 12 h, cells were collected to extract RNA for real-time quantitative polymerase chain reaction to detect the expression level of Hsp1a1, which was found significantly highly expressed after heat stimulation. (b) Western blot assay showed a significant increase in the HSP70 protein level. Proteins were collected from TM4 heat-excited cells after sodium dodecyl-polyacrylamide gel electrophoresis, and the developed results showed upregulation of the associated heat-excited protein levels. The results of the statistical analysis were also in line with expectations. *, p < 0.05; **, p < 0.01.
Figure 3: Heat stress induces activation of the Hippo pathway. (a) Changes in the levels of related proteins on the Hippo pathway after heat stress in TM4 cells. Immediately after different times of heat stress, cell extracts were collected for the Hippo pathway protein assays, and the phosphorylation level of YAP and the expression level of YAP/TAZ kinase were reduced. (b) The results of the statistical analysis were also in line with expectations. *, p < 0.05; **, p < 0.01; ***, p < 0.001.
Figure 4: TM4 cells after heat excitation may lead to activation of the Hippo pathway.
Immunofluorescence experiments revealed that the core transcription factor (yes-associated protein/transcriptional co-activator with PDZ-binding motif, YAP/TAZ) of the Hippo pathway clearly appeared to be aggregated in the nucleus.
Heat stress induces apoptosis and cell scorching in mouse TM4 support cells
Previous sequencing results have shown that many apoptosis-related pathways are enriched after heat stress, and the Hippo pathway is reported to regulate cell scorching and apoptosis (Cui et al., 2019; Zhao et al., 2020). To verify the effect of heat excitation on TM4 cells, an in vitro heat-stimulated mouse TM4 cell model was used and TUNEL experiments were performed; the staining results showed that a large number of TM4 cells underwent apoptosis after heat excitation (Fig. 5). We also found many scorched vesicles when culturing TM4 cells after heat excitation (Fig. 6a). Proteins were extracted from TM4 cells after heat excitation and a rise was found in the GSDME sheared N-segment fragment by western blotting assay (Fig. 6). Heat excitation is suggested to not only cause apoptosis in TM4 cells but also trigger cell scorch death. Meanwhile, TEM showed that TM4 cells showed cell membrane rupture, mitochondrial break damage, and autophagic vesicle production after heat excitation (Fig. 7). Combined with the previous experimental results, it can be concluded that heat excitation can induce apoptosis and cell scorch death in mouse TM4 support cells.
Figure 5: Many TM4 cells undergo apoptosis after heat stress. Mouse support cells were exposed to a water bath at 43°C for 20 min with the same batch of normally cultured TM4 cells as the control. After 12 h, fixed cells were stained for terminal deoxynucleotidyl transferase dUTP nick-end-labeling (TUNEL). As shown in the figure, many TM4 support cells underwent apoptosis after heat excitation. Red represents TUNEL-positive apoptotic cells, and blue represents DAPI-labeled nuclei.
Figure 6: Thermal effects induce cellular scorching in mouse TM4 cells. (a) After heat stress, TM4 cells produce scorched vesicles. After TM4 cells were heat-stimulated for 20 min and placed in a cell incubator for 12 h, microscopic observation revealed many scorched death vesicles (indicated by red arrows). (b) Heat-stimulated TM4 cells exhibited upregulated levels of relevant scorch markers. Cells were collected after different times of heat excitation, and relevant proteins were extracted for electrophoresis to detect the expression of the scorch death marker GSDME/N-terminal, which was found to increase significantly. The results of the statistical analysis were also in line with expectations.
Figure 7: Transmission electron microscopy (TEM) reveals significant structural changes in TM4 cells after thermal excitation. TEM reveals structural changes in TM4 cells after heat excitation. The microstructure of TM4 cells was observed by TEM after 20 min of heat excitation and placed in a cell incubator for 12 h. The cell membrane and mitochondria were found altered, and autophagic vesicles were produced.
The maintenance of an appropriate scrotal temperature is essential for spermatogenesis in mammals because spermatogenic cells in the testis are particularly sensitive to temperature (Sun et al., 2021). Temperature discomfort can lead to varying degrees of abnormalities in sperm quantity, quality, and dynamics (Mieusset and Bujan, 1995). In daily human life, different habits and even differences in occupational types can have varying effects on the testes. In addition, during human fever, the thermoregulatory function of the testes fails, and the scrotal temperature consequently increases, coinciding with the increase in core body temperature. Looking through past cases, we found several patients with a damaged reproductive system may have had acute infections. Once the patient develops a fever, the sperm quality and morphology show a certain tendency to decrease (Buch and Havlovec, 1991; Sergerie et al., 2007). A physical examination of nearly 30 men without disease was brought to our attention, and the report clearly showed that the more frequent or warmer the post-meiotic fever, the less competitive the sperms were, including items such as concentration, number, and viability (Carlsen et al., 2003). Overall, the total duration of fever, the stage at which the fever occurs, and the magnitude of the temperature difference between the two states with and without fever seem to determine the severity of testicular damage in some way. Thus, elevated salivary temperatures caused directly or indirectly by various heat sources can negatively affect semen production and even induce male infertility (Hu et al., 2021).
On the other hand, spermatogenesis is a complex, ordered, and finely regulated process in which spermatogonia stem cells proliferate, differentiate, and morph into mature sperm cells (Cai et al., 2019; Cannarella et al., 2020; Dunleavy et al., 2019). These processes take place in the convoluted sperm ducts of the testis. Each testis contains 600–1,200 convoluted seminiferous tubules. There is a spermatogenic epithelium on each of these tubules, where sperm production takes place (Bustos-Obregon et al., 1975). In terms of ultrastructure, the human curvilinear sperm duct is much the same as that of other mammals. It consists of a basement membrane that is immediately adjacent to the SCs. GCs, which have an organized structure, are distributed in the seminiferous tubules and are highly mature, with the basement membrane being occupied almost exclusively by cells with high maturity, which gradually differentiate along with the membrane as they enter the luminal region (Kurth et al., 1991). GCs, like all cells, need environmental support to divide and differentiate, in which the BTB plays an important role in regulating the intercellular transport of molecules (Feng et al., 2022).
Various studies have long established that the testis is highly immunogenic but that its internal germ-related cells do not exhibit an immune response. In an exhaustive examination of the internal structure of the testis, SCs were found to occupy a pivotal position in the face of the immune response (Kaur et al., 2014; Mital et al., 2010). SCs usually require BTB as a mediator to function, which protects germ cells from the host immune system, the main components of which include adjacent SCs and tight junctions (TJs), gap junctions (GJs), and ectoplasmic specialization (ES) between SCs (Mital et al., 2011). In addition to acting as a barrier between mature germ cells and immune cells, SCs can also regulate the immune response by expressing a variety of immunomodulatory factors (Kaur et al., 2014).
Apoptosis is a significant hallmark of male germ cell formation. In the late embryonic stage, when testicular differentiation is required for adjustment to an ideal ratio of GCs to SCs, gonadal cells are thus eliminated by apoptosis and cleared. During this development, many pre-meiotic spermatogonia are removed by apoptosis during the first round of spermatogenesis in the testis (Rodriguez et al., 1997). In adulthood, apoptosis again plays an instrumental regulatory function in the evolution of germ cells. For example, once germ cells are damaged, they undergo apoptosis, and the body defines these cells as faulty cells, cleaning them so that they want to become mature sperm is almost impossible. An important factor in the transmission of species from generation to generation is the selection of superior cellular traits and the rejection of cellular mechanisms that are detrimental to reproductive development. Various physical factors, toxins, radioactivity, etc., have the chance to stimulate the activation of this mechanism (Alam et al., 2010; Etemadi et al., 2020; Wang et al., 2017; Xue et al., 2022). If spermatogenesis is compromised in any way, germ cells often go into apoptosis by default (Lin et al., 2010). In the study of the etiology of some primary male infertility, various conditions of apoptosis that occur during the maturation time of spermatozoa have attracted the attention of a wide range of scholars. In addition, relevant experiments have shown that apoptotic signaling molecules are present in the mature spermatozoa of male infertility patients, including caspase activation, phosphatidylserine externalization flip, and the presence of TUNEL-positive signals (Shukla et al., 2012; Yin et al., 2018).
Initially, we designed the heat stress experiments from animal and cellular models to obtain the corresponding heat stress models and thus obtained relevant differentially expressed genes, which were subjected to raw letter analysis. The high expression of heat stress protein HSP70 was demonstrated, indicating the successful establishment of a mouse heat stress model. We also established a heat stress model supporting cellular TM4 through in vitro experiments. Heat stress was found to effectively induce protein degradation of LATS1 and dephosphorylation of YAP. YAP and TAZ play an important role in the heat shock response by enhancing the changes of TM4 after heat stress. It was suggested that the Hippo pathway may be involved in regulating the changes of heat shock in the supporting cells, in addition to apoptosis and cell scorching found in TM4 cells after heat shock. The Hippo pathway has also been shown to be associated with cell growth trajectory, so we combined the results of apoptosis and scorch death with this pathway and concluded that heat stress may activate Hippo signaling pathway and induce apoptosis and scorch death in TM4 support cells.
In the present study, it was found that several molecules on the Hippo pathway, such as Mob1, Myc, and Smad3, were differentially expressed, and molecules related to cell scorching and apoptosis, such as caspase-3, caspase-8, Apaf1, GSDMD, GSDME, and others were also significantly expressed (Hu et al., 2021). Because of its conserved evolution, the Hippo signaling plays a pivotal role in intracellular regulation, such as in cell development and homeostasis after tissue formation. Hippo signaling is one of the requisites if cells want to undergo division and proliferation, development, or even organ regeneration (Ma et al., 2019; Maejima et al., 2022; Meng et al., 2016). It facilitates the initiation of LATS1 kinase, which controls gene execution by repressing the activity of transcription coactivators (Zheng and Pan, 2019). The Hippo pathway is essential for cells to maintain homeostasis. On being exposed to different types of stress, cells respond through adaptations, such as reduced synthesis of proteins and fatty acids and increased synthesis of heat shock proteins and thioredoxin, in parallel with specific responses to stressors (Ibar and Irvine, 2020; Ma et al., 2019; Meng et al., 2016; Wang et al., 2020; Zheng and Pan, 2019). The stress faced by the cells beyond the adaptive limit leads to severe stress responses, such as apoptosis, autophagy, and cell scorching (Cui et al., 2019; Dutchak et al., 2022; Huang et al., 2005; Wang et al., 2020). In this study, a preliminary exploratory study on the activation of the Hippo pathway in heat-stimulated TM4 cells showed induction of the transfer of the YAP/TAZ complex from the cytoplasm to the nucleus, thereby regulating the activation of downstream target genes of the Hippo pathway. Since heat excitation is more damaging to the supporting cells, apoptosis and cell scorching induced by heat excitation may be due to the activation of the Hippo signaling pathway.
Availability of Data and Materials: All data generated or analyzed during this study are included in this published article (and its supplementary information files).
Author Contribution: ML designed this research. CW and ML wrote the manuscript. CW and CH performed the experiments. YG and KW reviewed the manuscript.
Ethics Approval: This study was approved by the Experimental Animal Ethics Committee of Bengbu Medical College (No. 2020-024).
Funding Statement: This research was funded by the Anhui Provincial Key Research and Development Project of the People’s Republic of China (202104j07020016), the 512 Talent Cultivation Plan of Middle-aged Backbone Teachers of Bengbu Medical College of China (by51201207), and the Climbing Plan of Natural Science General Project of Bengbu Medical College (China) (2021bypd003).
Conflicts of Interest: None of the authors have conflicts of interest to declare.
References
- Akintayo, A., Liang, M., Bartholdy, B., Batista, F., & Aguilan, J. (2020). The golgi glycoprotein MGAT4D is an intrinsic protector of testicular germ cells from mild heat stress. Scientific Reports, 10, 2135. [Google Scholar] [CrossRef]
- Alam, MS., Ohsako, S., Tay, TW., Tsunekawa, N., & Kanai, Y. (2010). Di(n-butyl) phthalate induces vimentin filaments disruption in rat Sertoli cells: A possible relation with spermatogenic cell apoptosis. Anatomia, Histologia, Embryologia, 39, 186-193. [Google Scholar] [CrossRef]
- Buch, JP., & Havlovec, SK. (1991). Variation in sperm penetration assay related to viral illness. Fertility and Sterility, 55, 844-846. [Google Scholar] [CrossRef]
- Bustos-Obregon, E., Courot, M., Flechon, JE., Hochereau-de-Reviers, MT., & Holstein, AF. (1975). Morphological appraisal of gametogenesis. Spermatogenetic process in mammals with particular reference to man. Andrologia, 7, 141-163. [Google Scholar] [CrossRef]
- Cai, B., Sun, DL., Deng, WM., & Jin, BF. (2019). Roles of cyclins in the progression of spermatogenesis. Zhonghua Nan Ke Xue, 25, 168-171. [Google Scholar]
- Cannarella, R., Condorelli, RA., Mongioi, LM., La Vignera, S., & Calogero, AE. (2020). Molecular biology of spermatogenesis: Novel targets of apparently idiopathic male infertility. International Journal of Molecular Sciences, 21, 1728. [Google Scholar] [CrossRef]
- Carlsen, E., Andersson, AM., Petersen, JH., & Skakkebaek, NE. (2003). History of febrile illness and variation in semen quality. Human Reproduction, 18, 2089-2092. [Google Scholar] [CrossRef]
- Cui, J., Zhou, Z., Yang, H., Jiao, F., & Li, N. (2019). MST1 suppresses pancreatic cancer progression via ROS-induced pyroptosis. Molecular Cancer Research, 17, 1316-1325. [Google Scholar] [CrossRef]
- Dunleavy, JEM., O’Bryan, MK., Stanton, PG., & O’Donnell, L. (2019). The cytoskeleton in spermatogenesis. Reproduction, 157, R53-R72. [Google Scholar] [CrossRef]
- Dutchak, K., Garnett, S., Nicoll, M., de Bruyns, A., & Dankort, D. (2022). MOB3A bypasses BRAF and RAS oncogene-induced senescence by engaging the hippo pathway. Molecular Cancer Research, 20, 770-781. [Google Scholar] [CrossRef]
- Etemadi, T., Momeni, HR., & Ghafarizadeh, AA. (2020). Impact of silymarin on cadmium-induced apoptosis in human spermatozoa. Andrologia, 52, e13795. [Google Scholar] [CrossRef]
- Feng, R., Adeniran, SO., Huang, F., Li, Y., & Ma, M. (2022). The ameliorative effect of melatonin on LPS-induced Sertoli cells inflammatory and tight junctions damage via suppression of the TLR4/MyD88/NF-kappaB signaling pathway in newborn calf. Theriogenology, 179, 103-116. [Google Scholar] [CrossRef]
- Hu, K., He, C., Ren, H., Wang, H., & Liu, K. (2019). LncRNA Gm2044 promotes 17beta-estradiol synthesis in mpGCs by acting as miR-138-5p sponge. Molecular Reproduction and Development, 86, 1023-1032. [Google Scholar] [CrossRef]
- Hu, K., He, C., Sun, X., Li, L., & Xu, Y. (2021). Integrated study of circRNA, lncRNA, miRNA, and mRNA networks in mediating the effects of testicular heat exposure. Cell and Tissue Research, 386, 127-143. [Google Scholar] [CrossRef]
- Huang, J., Wu, S., Barrera, J., Matthews, K., & Pan, D. (2005). The Hippo signaling pathway coordinately regulates cell proliferation and apoptosis by inactivating Yorkie, the Drosophila Homolog of YAP. Cell, 122, 421-434. [Google Scholar] [CrossRef]
- Ibar, C., & Irvine, KD. (2020). Integration of Hippo-YAP signaling with metabolism. Developmental Cell, 54, 256-267. [Google Scholar] [CrossRef]
- Kaur, G., Thompson, LA., & Dufour, JM. (2014). Sertoli cells—immunological sentinels of spermatogenesis. Seminars in Cell & Developmental Biology, 30, 36-44. [Google Scholar] [CrossRef]
- Kobori, Y. (2019). Home testing for male factor infertility: A review of current options. Fertility and Sterility, 111, 864-870. [Google Scholar] [CrossRef]
- Kurth, BE., Klotz, K., Flickinger, CJ., & Herr, JC. (1991). Localization of sperm antigen SP-10 during the six stages of the cycle of the seminiferous epithelium in man. Biology of Reproduction, 44, 814-821. [Google Scholar] [CrossRef]
- Levine, H., Jorgensen, N., Martino-Andrade, A., Mendiola, J., & Weksler-Derri, D. (2017). Temporal trends in sperm count: A systematic review and meta-regression analysis. Human Reproduction Update, 23, 646-659. [Google Scholar] [CrossRef]
- Lin, YC., Yao, PL., & Richburg, JH. (2010). FasL gene-deficient mice display a limited disruption in spermatogenesis and inhibition of mono-(2-ethylhexyl) phthalate-induced germ cell apoptosis. Journal of Toxicological Sciences, 114, 335-345. [Google Scholar] [CrossRef]
- Ma, S., Meng, Z., Chen, R., & Guan, KL. (2019). The Hippo pathway: Biology and pathophysiology. Annual Review of Biochemistry, 88, 577-604. [Google Scholar] [CrossRef]
- Maejima, Y., Zablocki, D., Nah, J., & Sadoshima, J. (2022). The role of the Hippo pathway in autophagy in the heart. Cardiovasc Research, 94, 1287. [Google Scholar] [CrossRef]
- Meng, Z., Moroishi, T., & Guan, KL. (2016). Mechanisms of Hippo pathway regulation. Genes & Development, 30, 1-17. [Google Scholar] [CrossRef]
- Mieusset, R., & Bujan, L. (1995). Testicular heating and its possible contributions to male infertility: A review. International Journal of Andrology, 18, 169-184. [Google Scholar] [CrossRef]
- Mital, P., Hinton, BT., & Dufour, JM. (2011). The blood-testis and blood-epididymis barriers are more than just their tight junctions. Biology of Reproduction, 84, 851-858. [Google Scholar] [CrossRef]
- Mital, P., Kaur, G., & Dufour, JM. (2010). Immunoprotective Sertoli cells: Making allogeneic and xenogeneic transplantation feasible. Reproduction, 139, 495-504. [Google Scholar] [CrossRef]
- Moye, AR., Bedoni, N., Cunningham, JG., Sanzhaeva, U., & Tucker, ES. (2019). Mutations in ARL2BP, a protein required for ciliary microtubule structure, cause syndromic male infertility in humans and mice. PLoS Genetics, 15, e1008315. [Google Scholar] [CrossRef]
- Rodriguez, I., Ody, C., Araki, K., Garcia, I., & Vassalli, P. (1997). An early and massive wave of germinal cell apoptosis is required for the development of functional spermatogenesis. EMBO Journal, 16, 2262-2270. [Google Scholar] [CrossRef]
- Sengupta, P., Dutta, S., & Krajewska-Kulak, E. (2017). The disappearing sperms: Analysis of reports published between 1980 and 2015. American Journal of Mens Health, 11, 1279-1304. [Google Scholar] [CrossRef]
- Sergerie, M., Mieusset, R., Croute, F., Daudin, M., & Bujan, L. (2007). High risk of temporary alteration of semen parameters after recent acute febrile illness. Fertility and Sterility, 88, 970.e1-970.e7. [Google Scholar] [CrossRef]
- Shukla, KK., Mahdi, AA., & Rajender, S. (2012). Apoptosis, spermatogenesis and male infertility. Frontiers in Bioscience (Elite Edition), 4, 746-754. [Google Scholar] [CrossRef]
- Sun, T., Zhang, Y., Li, J., Yu, H., & Song, L. (2021). Addition of peroxiredoxin 6 (PRDX6) to IVF fertilization medium maintains motility and longevity of human spermatozoa. BIOCELL, 45, 705-710. [Google Scholar] [CrossRef]
- Wang, C., Zhang, J., Li, Q., Zhang, T., & Deng, Z. (2017). Low concentration of BPA induces mice spermatocytes apoptosis via GPR30. Oncotarget, 8, 49005-49015. [Google Scholar] [CrossRef]
- Wang, D., He, J., Huang, B., Liu, S., & Zhu, H. (2020). Emerging role of the Hippo pathway in autophagy. Cell Death and Disease, 11, 880. [Google Scholar] [CrossRef]
- Wang, S., Zhou, L., Ling, L., Meng, X., & Chu, F. (2020). The crosstalk between Hippo-YAP pathway and innate immunity. Frontiers in Immunology, 11, 323. [Google Scholar] [CrossRef]
- Xue, H., Huo, Y., Hu, Y., Zhang, J., & Deng, C. (2022). The role of ALOX15B in heat stress-induced apoptosis of porcine Sertoli cells. Theriogenology, 185, 6-15. [Google Scholar] [CrossRef]
- Yao, G., Liang, M., Liang, N., Yin, M., & Lu, M. (2014). MicroRNA-224 is involved in the regulation of mouse cumulus expansion by targeting Ptx3. Molecular and Cellular Endocrinology, 382, 244-253. [Google Scholar] [CrossRef]
- Yin, J., Ni, B., Yang, YD., Tang, ZW., & Gao, ZQ. (2018). Elevation of autophagy rescues spermatogenesis by inhibiting apoptosis of mouse spermatocytes. Reproduction, 156, 545-558. [Google Scholar] [CrossRef]
- Yu, LEI., Fan, Z., Wang, H., Li, W., & Jing, T. (2021a). Ala-Gln improves varicocele-induced testicular injury by increasing HSP70 and antioxidant activity in male rats. BIOCELL, 45, 323-329. [Google Scholar] [CrossRef]
- Yu, P., Zhang, X., Liu, N., Tang, L., & Peng, C. (2021b). Pyroptosis: Mechanisms and diseases. Signal Transduction and Targeted Therapy, 6, 128. [Google Scholar] [CrossRef]
- Zhao, H., Liu, M., Liu, H., Suo, R., & Lu, C. (2020). Naringin protects endothelial cells from apoptosis and inflammation by regulating the Hippo-YAP pathway. Bioscience Reports, 40, BSR20193431. [Google Scholar] [CrossRef]
- Zheng, Y., & Pan, D. (2019). The Hippo signaling pathway in development and disease. Developmental Cell, 50, 264-282. [Google Scholar] [CrossRef]
Supplementary Materials
Cite This Article
WANG, C., HE, C., GAO, Y., WANG, K., LIANG, M. (2023). Heat exposure promotes apoptosis and pyroptosis in Sertoli cells. BIOCELL, 47(1), 155–164. https://doi.org/10.32604/biocell.2023.024657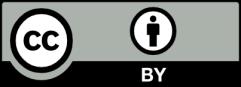