Open Access
ARTICLE
Generation of OAM Waves and Analysis of Mode Purity for 5G Sub-6 GHz Applications
1 Advanced Communication Engineering (ACE), Centre of Excellence, Faculty of Electronic Engineering Technology, Universiti Malaysia Perlis, Kangar, Perlis, Malaysia
2 Centre for Advanced Electrical & Electronic System (CAEES) Faculty of Engineering, Built Environment and Information Technology, SEGi University Petaling Jaya, Selangor, Malaysia
3 Department of Electrical Engineering, Universitas Sumatera Utara, Medan, 20155, Indonesia
4 School of Electrical and Electronic Engineering, Engineering Campus, Universiti Sains Malaysia, Nibong Tebal, 14300, Penang, Malaysia
5 Faculty of Engineering & Technology, Gomal University, 29050 kpk, Pakistan
* Corresponding Author: Mohd Najib Mohd Yasin. Email:
Computers, Materials & Continua 2023, 74(1), 2239-2259. https://doi.org/10.32604/cmc.2023.031170
Received 11 April 2022; Accepted 29 June 2022; Issue published 22 September 2022
Abstract
This article presents the generation of Orbital Angular Momentum (OAM) vortex waves with mode 1 using Uniform Circular Array (UCA) antenna. Two different designs, namely, UCA-1 (4-element array antenna) and UCA-2 (8-element array antenna), were designed and fabricated using FR-4 substrate to generate OAM mode 1 at 3.5 GHz (5G mid-band). The proposed antenna arrays comprised rectangular microstrip patch elements with inset fed technique. The elements were excited by a carefully designed feeding phase shift network to provide similar output energy at output ports with desired phase shift value. The generated OAM waves were confirmed by measuring the null in the bore sight of their 2D radiation patterns, simulated phase distribution and intensity distribution. The measurement results agree well with the simulation results. Moreover, a detailed mode purity analysis of the generated OAM waves was carried out considering different factors. The investigation found that the greater the number of elements, the higher the purity of the generated OAM wave. Compared with other previous works, the proposed antenna design of this paper is very simple to design and fabricate. In addition, the proposed antennas are compact in design even at lower frequency band with very wide bandwidth to meet the requirements of 5G mid-band applications.Keywords
Every year, the number of people subscribing to mobile broadband systems continues to increase substantially. People constantly prefer faster Internet connections, more appealing mobile phones, and rapid connectivity with others to share data [1]. As a result, wireless mobile devices and services have grown massively. Furthermore, mobile telecommunication providers are encountering an ever-increasing demand from new wireless applications for higher data rates, broader network capacity, high spectral efficiency, higher energy efficiency, and greater flexibility. Smartphone traffic is anticipated to expand tenfold between 2017 and 2022, while overall mobile traffic will increase eightfold [2]. With the limited capability of earlier wireless generation technologies, managing this massive quantity of mobile data traffic is difficult. With present technologies, 4G networks have almost reached the theoretical data rate limit and are inadequate to handle the above-stated difficulties [3]. To solve the above issue, researchers have decided to move to a higher unused frequency spectrum to replace 4G with 5G. Compared to the present 4G networks, 5G networks will enable 10- to 100-times faster data rates with up to 10-times lower latencies. This can be achieved through more efficient radio technology (spectral efficiency) and more spectrum bandwidth (spectral capacity). The latest fifth-generation (5G) system is expected to be 100 times faster than the previous 4G system with new services such as smart homes, smart cars, the Internet of Things (IoT), and so on. As an outcome, 5G technology has risen to the top of the 2020 generation’s demand list. The next generation of technology would enable high data rates, lower latency, additional capacity, and excellent quality of service. It is worth noting that 5G technology will open up new methods to overcome existing development limitations.
One possible way to utilize 5G wireless communication is by using Orbital Angular Momentum (OAM) vortex waves instead of conventional plane waves. The primary reason to use OAM waves instead of conventional plane waves is that OAM wave has the potential to allow infinite channels for wireless communication at Radio-Frequency (RF), giving it a new degree of freedom [4,5]. OAM wave has a large number of available topological charges, which are also known as OAM-modes. Different OAM-modes are orthogonal to each other, which would allow them to be multiplexed together to increase channel capacity [6]. Since an OAM wave has the potential to carry different modes, a new way can increase the spectrum efficiency. Accordingly, the OAM wave can be used for the upcoming 5G wireless communication system where higher data rates, spectrum efficiency, and security are in user demand. It was realized that the Orbital Angular Momentum (OAM) property (helical phased beams) of EM waves gives a rotational degree of freedom in the EM field by a phase factor
Figure 1: OAM vortex wave with mode 1 propagation
In literature, various methods are proposed to generate OAM waves [9]. However, the uniform circular array is considered the matured approach to generating OAM waves due to its great adaptability, capacity to generate multiple OAM modes, compactness, and low cost [10]. In [11], the authors have designed a circular phased antenna array to generate OAM mode 1, where four identical rectangular patches were used to operate at 2.5 GHz. Although the measurement results have proved that they have generated OAM waves based on the spiral wavefront, the purity of the generated OAM mode is not discussed. Ke bi et al. [12] designed a Global Positioning System (GPS) ceramic array antenna to generate a negative mode (−1) at 1.550 GHz. In this design, six antenna elements were placed on a ceramic substrate with a thickness of 4 mm and permittivity of 21.4; however, the obtained gain value is not disclosed in the paper. Moving to a frequency of 73.5 GHz, the authors in [13] have designed two dipole antenna arrays, one with eight elements and the other with 12 elements, to generate OAM mode +1 and +3, respectively. The substrate used in both designs was FR4 with a thickness of 0.127 mm and permittivity of 3.75. The simulated gain achieved by the 8-element dipole array for mode 1 was 3.81 dB, while the gain achieved by the 12-element dipole array for mode 3 was 2.53 dB. However, the measured values are not disclosed. From the literature, it can be seen that the majority of the authors ignored the OAM mode purity information. Previous works have only focused on generating OAM waves with a helical wavefront. So far, it has been understood that generating OAM beams with a spiral phase distribution is essential to make sure the modes are orthogonal to one another; however, very little research has been carried out on the purity of generated OAM modes. Only having spiral phase distribution is insufficient to utilize the intrinsic characteristics of OAM beams. Factors such as the number of elements, observation plane size, and receiver size must be considered to generate OAM modes with high purity. Regarding the practical potential of the OAM antenna, it is essential to ensure the generate mode is high in purity while maintaining a helical wavefront for the modes to be orthogonal to one another for efficient data transmission.
In this work, two uniform circular antenna arrays (UCA) at 5G mid-band (3.5 GHz) were designed, fabricated, measured, and compared in terms of bandwidth, OAM mode purity, and helical wavefront. The first design (UCA-1) comprises four identical radiating elements (patches), while the second design (UCA-2) comprises eight identical elements (patches). The antenna elements are rectangular microstrip patches with an inset feed technique. A comprehensive analysis of mode purity for different numbers of elements, observation plane sizes, and observation plane distances from the transmitting antenna array was carried out. Compared to previous works in [12–15], the proposed designs achieved broader bandwidth, meeting the 5G-mid-band requirement as well as it is much compact with simple design. The previous works did not carry out mode purity analysis, whereas a detailed mode purity analysis is carried out in this paper, which would assist the researchers and engineers in deciding the antenna size at receiving side to capture the incoming OAM waves from the transmitting side. This paper is organized as follows. The design of the antenna arrays is described in Section 2. Next, in the subsection of Section 2, the simulation results of the two designs are presented, such as phase difference and amplitude, as well as the fabrication process. The reflection coefficient (S11), bandwidth, phase distribution, current distribution, E-field vector, 2D radiation pattern, gain plot and mode purity are discussed in the subsequent section. Finally, conclusions are drawn in Section 4.
In this section, the design of the antenna arrays to generate OAM waves for the 5G mid-band is presented. The antenna arrays consist of rectangular microstrip patch antennas, which are uniformly arranged in a circle and excited with a common input feeding. The overall design specification is tabulated in Tab. 1.
The UCAs comprise identical microstrip patch antennas, which are uniformly placed in a circle on an FR-4 substrate with a thickness of 1.6 mm and a dielectric constant of 4.3. Microstrip patch antennas mainly comprise a radiating layer, substrate, and ground plane [16,17]. The substrate itself is located on a ground plane. The microstrip patch antenna design is selected for this experiment due to its low profile, low cost, easy fabrication, and lightweight [18]. The dimension of the single antenna element is calculated based on equations 1-5 [19]. Once the calculated dimensions were obtained, the following procedure was to design the antenna using CST Studio Suite 2019 software. However, the calculated antenna design did not operate at the desired frequency of 3.5 GHz. In addition, the reflection coefficient (S11) of the calculated design at 3.5 GHz was −7.10 dB which showed a poor impedance matching, and sufficient power could not be transferred to the patch. Consequently, the antenna dimensions were optimized, and the inset feed technique was applied. Inset Feed causes high impedance matching so that maximum power from the generator side reaches near the center of the patch [20]. The dimensions of the optimized patch antennas are shown in Fig. 2a, which are chosen to meet the requirements in Tab. 1. The simulated parameter obtained using CST 2019 studio is shown in Fig. 2b. It can be seen that the patch antenna has a resonance at the desired frequency of 3.5 GHz. Reflection coefficient (S11) value of −42.76 dB at the operating frequency indicated sufficient power is transferred to the patch antenna from the source.
Figure 2: Individual patch antenna (a) Optimized dimension (b) S11 value at 3.5 GHz for the optimized antenna
Two uniform circular arrays (UCA) are designed, one with four elements (UCA-1) and the other with eight elements (UCA-2). For an
For each of the UCAs, their elements are excited by a single source. Equal power distribution to the elements is achieved using a feed network with T-junction dividers. The desired phase difference between adjacent elements is achieved by manipulating the feed line’s lengths and the structure’s symmetry. The feed network of UCA-1 is shown in Fig. 3a with patches and Fig. 3b without patches, consisting of five ports. Port 1 is the input port, whereas Port 2, Port 3, Port 4, and Port 5 are the output ports used to excite Patch 1, Patch 2, Patch 3, and Patch 4, respectively. The feed network design to achieve the desired phase difference between adjacent elements proceeds as follows. The feed network is divided into the lower part (Port 2 and Port 3) and the upper part (Port 4 and Port 5), as illustrated in Fig. 3b. First, the feed network for the lower part is designed. Power of equal amplitude to Port 2 and Port 3 is achieved using a T-junction divider. A phase difference between the power at Port 2 and Port 3 is achieved by optimizing the lengths of the feedlines. The upper part of the feed network is then constructed, identical to the lower part but rotated, as shown in Fig. 3b. The patches are then attached to their respective ports, with the patches in the lower part-oriented upside down concerning those in the upper part. That means, being fed with the power of the same phase, Patch 1 and Patch 3 have a phase difference of 180°. Therefore, the requirement for the 90° phase shift increment from Patch 1 to Patch 4 is satisfied. The same technique was applied for UCA-2 feeding network and the overall feeding network of UCA-2 is shown in Figs. 3c and 3d.
Figure 3: Simulated circular array antenna (a) UCA-1 radiating patch configuration (b) The feeding network of UCA-1 (c) UCA-2 radiating patch configuration (d) The feeding network of UCA-2
For UCA-1, the overall phase difference for patch 1 (port 2), patch 2 (port 3), patch 3 (port 4), and patch 4 (port 5) were 4.53°, 95.76°, 6.63°, and 96.9° as shown in Fig. 4a. The additional 180° phase shift due to the mirror image of the feeding network gives port four an overall phase shift of 184.53° and port five a phase shift of 276.9°. As a consequence, the incremental phase shift for UCA-1 is 4.53°, 95.76°, 184.53°, and 276.9°. The phase difference between patch one and patch 2 is 91.23°, patch two and patch 3 is 88.77°, patch three and patch 4 are 92.37°, and patch four and patch 1 are 87.63°. Based on the calculated value, the phase difference among the adjacent elements should be 90°, and from the simulation results, it has been observed that the obtained values are close to 90°. Some differences between calculated and simulated values are due to the misalignment of the output ports. Moreover, the amplitude was almost similar for all the four radiating elements, as shown in Fig. 4b. The amplitude for patches 1 (port 2), 2 (port 3), 3 (port 4), and 4 (port 5), were −8.91 dB, −9.76 dB, −8.84 dB and −9.6 dB.
Figure 4: The Simulated results of UCA-1 (a) Phase shift among the elements (b) Amplitude of radiating patches
For UCA-2, the phase difference for patch 1 (port 2), patch 2 (port 3), patch 3 (port 4), patch 4 (port 5), patch 5 (port 6), patch 6 (port 7), patch 7 (port 8) and patch 8 (port 9) were 8.04°, 51.22°, 97°, 142.8°, 9.5°, 49.22°, 90.4° and 134.7° respectively as depicted in Fig. 5a. Due to the mirror image of the feeding network, an additional 180° phase shift is added from patch 5 to patch 8. Hence, the incremental phase shift for UCA-2 is 8.04° (patch 1), 51.22° (patch 2), 97° (patch 3), 142.8° (patch 4), 189.5° (patch 5), 229.22° (patch 6), 270.4°(patch 7) and 314.7°(patch 8). According to the calculated value, the phase difference between the adjacent elements for UCA-2 should be 45°, and it is observed from the simulation results that the obtained values are close to 45°. Furthermore, the amplitude at port 2 to port 9 were –12.75 dB, –12.30 dB, –12.75 dB, –11.53 dB, –14.27 dB, –14.29 dB, –11.73 dB and –13.64 dB as shown in Fig. 5b.
Figure 5: The simulated results of UCA-2 (a) Phase shift among the elements (b) Amplitude of radiating patches
Once the simulation result was obtained, the next task was to fabricate the prototypes, as shown in Figs. 6a and 6b. Moreover, 50 Ω SMA was connected at the standard input port to excite the radiating elements, as illustrated in Fig. 6c.
Figure 6: Prototype of the proposed designs (a) UCA-1 (b) UCA-2 (c) Soldering 50 Ω SMA connector
3 Experimental Setup, Results and Discussion
In this section, the results obtained from the simulation and experiment are discussed. Moreover, discussions regarding simulated and measured S11 values as well as bandwidth are summarized in this section. The simulated and measured 2D radiation pattern results prove that the proposed designs generated OAM mode 1 at the desired frequency band, and both the designs are compared with each other. Furthermore, the simulated gain obtained by UCA-1 and UCA-2 is also summarized in this section. Lastly, a detailed analysis of OAM mode purity was carried out based on the simulated designs where factors such as the number of antenna elements, observation plane radius, and distance from the transmitting antenna were taken into consideration to find out how it influences OAM mode purity.
3.1 Phase and Intensity Distribution
To verify that the designed UCAs would generate OAM wave with mode 1, we first simulated the phase distributions of the radiation produced by the UCAs, on a transverse observation plane with a radius of 75 mm, located 600 mm away from the UCAs. Figs. 7a and 7b illustrated the simulated phase distributions of the radiation generated by the UCAs from 0° to 360°. From Figs. 7a and 7b, it is evident that both UCAs exhibit a spiral phase distribution with a continuous phase change around the centre, which is a characteristic feature of OAM wave. In addition, it can be observed from Fig. 7c that there is a donut structure at the centre (black dot inside the blue circle shape) of the beam, which depicts the intensity of the generated OAM mode. As reported in [22,23] that OAM vortex beams with mode (
Figure 7: Simulated phase distribution of the radiation generated by (a) UCA-1 (b) UCA-2 (c) Simulated intensity of OAM mode 1
3.2 Reflection Coefficients and Bandwidth of the Proposed Designs
The measurement setup to measure the reflection coefficient (S11) and bandwidth of UCA-1 and UCA-2 are shown in Figs. 8a and 9a, respectively. The instrument used was Vector Network Analyzer (VNA) E5071C by Agilent Technologies. Fig. 8b shows the simulated and measured for UCA-1. It has been observed that the simulated S11 value was −15.92 dB with a bandwidth value of 560.40 MHz for UCA-1. Nevertheless, during actual measurement, the obtained S11 value was −10.80 dB with a bandwidth value of 420 MHz. For UCA-2, the simulated and measured results were very close. As shown in Fig. 9b, the simulated S11 value was −22.07 dB with a bandwidth value of 547.4 MHz, while the measured S11 value was −22 dB with a bandwidth value of 551.4 MHz. For UCA-2, the simulated and measured results matched very well compared to UCA-1. The simulated and measured results mismatch is attributed to fabrication error and measurement device uncertainty due to unequal lengths of coaxial lines and cables twisting. Nevertheless, both the designs have obtained an accepted S11 value and minimum 100 MHz bandwidth as per as the requirement of 5G mid-band deployment. It can observe that, for the same OAM mode 1, UCA-2 performed much better in terms of S11 value and bandwidth than UCA-1.
Figure 8: S11 and bandwidth measurement (a) UCA-1 measurement setup (b) Simulated and measured results of UCA-1
Figure 9: S11 and bandwidth measurement setup (a) UCA-2 setup (b) Simulated and measured results of UCA-2
The experimental setup to measure the 2D radiation patterns in the E-plane of the UCAs is shown in Fig. 10a. The proposed designs acted as the transmitting antenna while the horn antenna at the other end acted as the receiving antenna, as shown in Figs. 10b and 10c. The entire setup was done inside the Anechoic chamber of Advanced Communication Engineering (ACE), Universiti Malaysia Perlis, Malaysia. The radiation pattern setup for UCA-1 and UCA-2 are shown in Figs. 10b and 10c, respectively.
Figure 10: 2D radiation pattern measurement (a) Overall graphical presentation of the measurement setup (b) UCA-1 setup (c) UCA-2 setup
The simulated and measured normalized 2D polar radiation pattern at phi = 0 (E-plane or xz plane) is shown in Fig. 11. As shown in Fig. 11, the simulation and measurement results of the radiation pattern are in good agreement for UCA-1 and UCA-2. An intensity null in the center of the radiation pattern, characteristic of OAM waves [24], is apparent in the plot. For UCA-2, the intensity null in the centre is more pronounced, suggesting that a more significant number of elements produces a purer OAM mode. However, there are some differences between the simulation and measurement results due to variations in fabrication and the presence of the Antenna Under Test (AUT) stand. Moreover, it can be observed that the measured beamwidth is narrower than the simulated beamwidth for both UCA-1 and UCA-2. In addition, compared to UCA-1, there are more sidelobes present in UCA-2, as shown in Fig. 11b for the same OAM mode 1. It is because there are eight radiating elements in UCA-2 which caused the array to be more extensive in size, while there are four radiating elements in UCA-1. When the array size increases, the beamwidth becomes more directive; however, the sidelobes increase. Another significant observation can be made from Fig. 11b. When the antenna number radiating element increases and array size enlarges, the sidelobes become closer to the main lobe. If the sidelobes become close to the main lobe, it causes a significant energy loss. Therefore, several radiating elements and overall array size must be considered while generating OAM waves using circular array antennas. In addition, the simulated gain and directivity of UCA-1 were 3.245 dBi and 9.265 dBi, respectively. For UCA-2, the gain obtained was 2.346 dBi, and directivity was 9.161 dBi. The simulated gain for the proposed designs is illustrated in Fig. 12. The gain is lower for UCA-2 because of the side lobes [25] since the array radius for UCA-2 is 84 mm while the radius is 73 mm for UCA-1. This increased array radius size will narrow the main lobe beam width and increase the side lobe radiation [26].
Figure 11: Simulated and measured 2D radiation pattern in E-plane for (a) UCA-1 (b) UCA-2
Figure 12: Simulated gain for UCA-1 and UCA-2
3.4 Current Distribution, Vector and Magnitude of Electric Field
The simulated current distribution of UCA-1 and UCA-2 designs at 3.5 GHz is shown in Fig. 13. The charges must accelerate and decelerate to create a time-varying current to create radiation. Therefore, on a patch, how the charges are accumulated and where the charges are accumulated are explained by the current distribution in Fig. 13. It can be observed from Fig. 13a that the current distribution for patches 1 and 3 takes place together along the edges, while there is no distribution for patches 2 and 4. Furthermore, it can be seen from Fig. 13b that the current distribution is along the edges of patches 2 and 4, while there is the current distribution from the edges of patches 1 and 3. It is due to the difference in phase and feedline length. Similar behaviour can be observed in Figs. 13c and 13d. The current distribution illustrates that the wave is launched from the transmission line to the patch. Furthermore lastly, the wave is radiated from the edges of the patch. The current distribution at the edge of the patch element has also been observed in [27]. As modal currents are focussed on the edges, the length of the edges significantly impacts the resonance frequency of the characteristic modes.
Figure 13: Surface current distribution at 3.5 GHz (a) Patch 1 and 3 of UCA-1 (b) Patch 2 and 4 of UCA-1(c) Patch 1,2,5 and 6 of UCA-2 (d) Patch 3,4,7 and 8 of UCA-2
The vector and magnitude of the electric field at 3.5 GHz of UCA-1 and UCA-2 at three different phases, namely, phases 0, 180, and 360, are shown in Tab. 3. The concerned area, where the vector and magnitude of electric field (V/m) are plotted, is placed above the proposed circular array antennas and perpendicular to the
Cite This Article
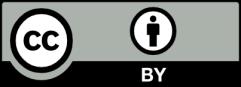