Open Access
ARTICLE
Effects of Allelochemicals on Root Growth and Pod Yield in Response to Continuous Cropping Obstacle of Peanut
1 Institute of Crop Germplasm Resources, Shandong Academy of Agricultural Sciences, Jinan, 250100, China
2 Shandong Key Lab of Genetic Improvement, Ecology and Physiology of Crops, Jinan, 250100, China
3 Scientific Observation and Experiment Station of Crop Cultivation in East China, Ministry of Agriculture and Rural Affairs, Dongying, 257100, China
4 Shandong Agricultural Engineering University, Jinan, 255300, China
5 Institute of Agricultural Resources and Environment, Shandong Academy of Agricultural Sciences, Jinan, 250100, China
* Corresponding Authors: Ping Liu. Email: ; Shubo Wan. Email:
Phyton-International Journal of Experimental Botany 2023, 92(1), 17-34. https://doi.org/10.32604/phyton.2022.022405
Received 08 March 2022; Accepted 15 April 2022; Issue published 06 September 2022
Abstract
Continuous cropping (CC) obstacle is a major threat in legume crops production; however, the underlying mechanisms concerning the roles allelochemicals play in CC obstacle are poorly understood. The current 2-year study was conducted to investigate the effects of different kinds and concentrations of allelochemicals, p-hydroxybenzoic acid (H), cinnamic acid (C), phthalic acid (P), and their mixtures (M) on peanut root growth and productivity in response to CC obstacle. Treatment with H, C, P, and M significantly decreased the plant height, dry weight of the leaves and stems, number of branches, and length of the lateral stem compared with control. Exogenous application of H, C, P, and M inhibited the peanut root growth as indicated by the decreased root morphological characters. The allelochemicals also induced the cell membrane oxidation even though the antioxidant enzymes activities were significantly increased in peanut roots. Meanwhile, treatment with H, C, P, and M reduced the contents of total soluble sugar and total soluble protein. Analysis of ATPase activity, nitrate reductase activity, and root system activity revealed that the inhibition effects of allelochemicals on peanut roots might be due to the decrease in activities of ATPase and NR, and the inhibition of root system. Consequently, allelochemicals significantly decreased the pod yield of peanut compared with control. Our results demonstrate that allelochemicals play a dominant role in CC obstacle-induced peanut growth inhibition and yield reduction through damaging the root antioxidant system, unbalancing the osmolytes accumulation, and decreasing the activities of root-related enzymes.
Keywords
Legume crops belong to the Leguminosae or Fabaceae family and rank third in global crop production after cereal and oilseed crop species [1,2]. Legume crops are also key components of healthy diets and productive crop rotations, which play important roles in global agricultural productivity and food security [3–6]. Continuous cropping (CC) is the concept that the same crop species or its relatives is grown on the same area of land over long-term (Long-term monoculture). CC obstacle is a global challenge that severely affects the growth and causes the degeneration of soil, induction of serious diseases, and decline in yields of legume crops even if the field management regime has been properly adopted [7–9]. CC obstacle, of which land degradation is considered a common type, is usually caused by overcultivation [10–12]. From the perspective of land, long-term CC could affect the microbial community structure and diversity in the soil which might lead to obstacle [13–15]. Consequently, CC obstacle could alter soil properties and ecological functions and ultimately prohibit the healthy growth and development of crop species especially legumes. However, the mechanisms regarding the side effects of CC obstacle in legume crops production are poorly understood.
In the past decades, the remediation effects on CC obstacle have been extensively investigated especially in legume crops [16–18]. Recently, Zeng et al. [15] reported that CC obstacle was ameliorated by intercropping patchouli with turmeric or ginger, probably through improving soil bacterial metabolism and the activities of soil enzymes. In another study, CC of strawberry led to three phases of changes in abiotic and biotic soil factors. Notably, the accumulation of phenolic acids have been demonstrated to be vital chemicals that significantly inhibited strawberry growth [10]. However, strategies in response to CC obstacle on legume crops especially in peanut production still remain elusive.
Allelochemicals are major components of root exudates in legume crops that have multiple biological functions. Most of the allelochemicals are secondary products released from plant root excretions or plant residues, such as phenolic acids, luteolin, and resveratrol, which severely accumulate in the soil of legumes CC systems [19–21]. Experiments indicated that allelochemicals were a group of promising compounds that could be taken as weed inhibitors due to their environmentally friendly properties [22–25]. As major components of allelochemicals induced by allelopathic effects, phenolic acids could influence the growth, productivity, and yield of the crops [26,27]. The underlying mechanisms concerning the allelopathic effects on crops including the inhibition of photosynthesis and enzyme action, the suppression of hormone activity, and the reduction of root growth and ion uptake rate [28–30]. To date, the mechanisms governing the roles allelochemicals play in CC obstacle of legume crops have not been determined in detail. In this regard, conducting research on the effects of allelochemicals especially phenolic acids on peanut growth and yield formation has important theoretical and practical significance for both scientific research and combating CC obstacle.
Therefore, the present study was carried out to interpret the causes of CC obstacle in relation to the allelochemicals (phenolic acids) in peanut growth and to clarify the physiological mechanisms governing this process. We hypothesize that allelochemicals might have negative effects on peanut growth, root physiology, and yield formation. Using different concentrations and kinds of allelochemicals, we investigated the changes in morphological characters, antioxidant systems, osmolytes accumulation, activities of NR and ATP, and nutrient content in peanut roots. The pod yield and yield components exposed to various allelochemicals were also compared with the control to test our hypothesis. This study revealed the underlying mechanism involved in allelochemicals on peanut root growth, thereby contributing to a better understanding of the involvement of allelochemicals in CC obstacle of legume crops.
2.1 Plant Materials and Growth Conditions
Peanut (Arachis hypogaea L.) cultivar Huayu 22 was used in this experiment. The 2-year pot-grown experiments were conducted at Yinmaquan experimental station, Jinan, China (36°43’15’’N, 117°5’50’’E) in 2016 and 2017. The seeds of uniform sizes were selected and soaked in a 2% (v/v) sodium hypochlorite for 15 min, and then thoroughly rinsed in sterile distilled water. The seeds were planted in polystyrene pots (with 28 cm inner diameter, 42 cm depth, and small holes at the bottom, each pot contained six seeds) on 12 May 2016 and 14 May 2017. Each pot was filled with 18 kg of garden soil (obtained from 0 to 20 cm below the soil level) and basal synthetic fertilizers of 975 kg hm−2 (N:P2O5:K2O = 1:1.5:1.5) before sowing. The main physical and chemical properties of the garden soil were as follows: pH, 7.02; bulk density, 1.21 g/cm3; organic matter, 22.8 g/kg; nitrogen, 85.6 mg/kg; phosphorous, 21.4 mg/kg; and potassium, 81.3 mg/kg. The soil was initially free from uncontrolled allelochemicals as verified by the method of gas chromatography-mass spectrometry. After sowing, each pot was filled with 2 L of distilled water and covered with plastic film. All the pots were placed in a greenhouse with the following conditions: photoperiod of 14/10 h (light/dark), photosynthetic photon flux density (PPFD) of 1200 µmol m−2s−1, air temperature of 25/18°C (day/night), and air humidity of 60%. Each pot was watered with 500 mL distilled water every three days. On 01 June 2016 and 03 June 2017, the seedlings were thinned out with one strong seedling left in each pot. At four-leaf stage, pots with similar-looking seedlings were selected for the subsequent experiments.
The phenolic acid allelochemicals treatments were exposed to the seedlings on 13 June 2016 and 15 June 2017 (four-leaf stage). In order to simulate the actual CC obstacle in peanut production, the concentrations of allelochemicals used in this study were based on our preliminary experiments that were identical to those in the root exudates of peanut when continuous cropping for 5 successive years [31]. Each pot was well watered with 2 L of distilled water (CK), 360 μg L−1 of p-hydroxybenzoic acid (H1), 720 μg L−1 of p-hydroxybenzoic acid (H2), 360 μg L−1 of cinnamic acid (C1), 720 μg L−1 of cinnamic acid (C2), 360 μg L−1 of phthalic acid (P1), 720 μg L−1 of phthalic acid (P2), 360 μg L−1 of the mixture of H1, C1, P1 (4:10:7, w:w:w) (M1), and 720 μg L−1 of the mixture of H2, C2, P2 (4:10:7, w:w:w) (M2). The measurements and samples were taken at 75 d after emergence unless otherwise stated. In total, 9 treatments were composed with three replicates, and each replicate consisted of 12 plants.
2.3 Agronomic Characteristics Analysis
The height of the main stem was firstly determined from the base to the top of the main stem of the peanut plant. Meanwhile, the number of branches and the length of the lateral stems were also investigated. Then the samples were separated into stems and leaves, oven-heated at 105°C for 30 min to deactivate enzymes, and dried to constant weight at 75°C before the dry weights were recorded [32].
2.4 Antioxidant Enzyme Activity and MDA Content Assay
In order to assay the activities of antioxidant enzymes, the root samples (0.5 g) were immediately ground with 5 mL 25 mM HEPES-NaOH buffer (pH 7.8) using pre-chilled mortar and pestle. The main components of the buffer were as follows: 20% (v/v) glycerol, 1 mM ethylenediaminetetraacetic acid (EDTA), 1 mM ascorbic acid (AsA), 1 mM dithiothreitol (DTT), 5 mM MgCl2, and 1 mM reducing glutathione (GSH). The homogenates were centrifuged at 12,000 g and 4°C for 20 min using a centrifuge (5810R, Eppendorf, Germany), then the supernatants were collected for the determination of the enzymatic activity. All of the parameters were determined using a spectrophotometer (UV-2401, Shimadzu, Japan). According to Bradford [33], the protein content was determined prior to the determination of antioxidant enzymes. Superoxide dismutase (SOD) activity was determined by the measurement of the reduction of nitro-blue tetrazolium at 560 nm according to Stewart et al. [34]. Peroxidase (POD) activity was assayed by taken guaiacol oxidation as a substrate at 470 nm as modified by the method of Cakmak et al. [35]. Catalase (CAT) activity was measured as a decline at 240 nm based on the method of Patra et al. [36]. The lipid peroxidation was determined according to the thiobarbituric acid (TCA) reaction through determination of the malondialdehyde (MDA) content as mentioned by Hodges et al. [37]. MDA content was calculated at 532 nm by subtracting the absorbance at 600 nm for non-MDA compounds.
2.5 Root Morphology and Activity of Root System Assay
The root morphological characters were analyzed as modified by the method of Jiang et al. [38], the fresh roots were carefully washed twice with distilled water, and then scanned with a dual lens scanning system (Epson V700, Nagano-ken, Japan). The root images were analyzed with WinRHIZO software (Regent Instruments, Canada). The data of the total root length, average root diameter and root surface area were collected. Then the root samples were over-dried at 80°C for 2 d to get the root dry weight data.
The activity of root system was evaluated at 45, 75, and 105 d after emergence as previously described by Ruf et al. [39] and Begum et al. [40]. The fresh root samples were taken immediately and determined using the triphenyl tetrazolium chloride (TTC) method and the root activity was expressed as the deoxidization ability of TTC with the formula: TTC reducing intensity (μg TTC g−1 h−1) = TTC reduction mass (μg)/Root weight (g) × time (h).
2.6 NR and ATPase Activity Assay
The nitrate reductase (NR) activity was assayed at 45, 75, and 105 d after emergence with some modifications as originally described by Scheible et al. [41]. Briefly, frozen leaf tissues (0.3 g FW) were ground in 100 mM phosphate buffered solution (pH 7.5). The determination was started by mixing one part of extract with five parts of reaction buffer (0.25 mM NADH, 5 mM KNO3) and incubated at 25°C for 30 min. The reaction was then stopped by adding 0.1 M zinc acetate. After reaction for 15 min, the tubes were centrifuged at 13,000 g for 10 min. The nitrite produced was then measured by adding 1 mL of 1% (w/v) sulfanilamide in 3 M HCl plus 1 mL of 0.02% (v/v) N-(1-naphthyl)-ethylenediamine in distilled water using spectrophotometry analysis at 520 nm.
The ATPase activity was determined at 45, 75, and 105 d after emergence as previously described [42,43] with some modifications. This method was to determine the Pi released by the microsomal ATPase during ATP hydrolysis after incubation in the assay medium (50 mM BTP-Cl (pH 6.5), 100 mM KCl, 5 mM BTP-ATP, and 5 mM MgSO4) at 30°C for 30 min. Then the reaction solution was determined using a spectrophotometer at 820 nm.
2.7 Determination of the Contents of the Total Soluble Sugars and Total Protein
For the assay of the contents of the total soluble sugars and total protein, the third fully expanded leaves on the main stem grown in the same positions were firstly oven-dried for 15 min at 105°C and then 85°C for 3 d. Then the samples were ground in a high-speed ball mill (MM400; Retsch, Haan, Germany) and mixed thoroughly. The root instances (0.1 g) were extracted twice with 80% (v/v) ethanol at 80°C for 10 min and centrifuged at 3,000 × g for 30 min. The supernatant was then collected (repeated three times) in a 30 ml glass tube and the contents of soluble sugars and free amino acids were measured consequently. The total soluble sugar content was assayed at 620 nm using anthrone method according to the method of Buysse et al. [44]. The total protein content was determined following the trichloroacetic acid (TCA) propanone sediment method as described by Si et al. [45].
2.8 Determination of the Nutrient Uptake
Dried materials from harvests were weighed and ground in a high-speed ball mill (MM400; Retsch, Haan, Germany) and sifted through a 0.5 mm screen for the analysis of total nitrogen, total phosphorus, and total potassium. After slaking with nitric-perchloric acid (5:3), the total nitrogen concentration was analyzed by the micro-Kjeldahl method according to Aljazairi et al. [46]. After acid digestion, the concentration of phosphorus was quantified by colorimetry following the method of Chi et al. [47]. The potassium concentration was determined by using an atomic adsorption spectrophotometer based on the protocol of Guo et al. [4]. Concentrations of all nutrients were expressed on a dry weight percentage basis.
2.9 Pod Yield and Yield Components
The peanut pods were manually harvested upon maturity on 26 September 2016 and 27 September 2017. The pod yield and yield components of peanut including pods number per plant, 100-seed weight and shelling rate were measured for all plants in the sampling area after sun-drying for 15 d.
The experimental design was a completely randomized block design with three replicates. Statistical analysis of the bioassays was performed using the SPSS statistical package, and differences between the treatment means were compared by Tukey’s test at a level of P < 0.05.
3.1 Effect of Allelochemical Treatment on Peanut Agronomic Characteristics
To examine the effect of allelochemicals on peanut growth, we determined the agronomic characteristics of peanut seedlings. As shown in Table 1, both the low (360 μg L−1) and high (720 μg L−1) concentration of p-hydroxybenzoic acid (H), cinnamic acid (C), phthalic acid (P), and their mixtures (M) induced a substantial decrease in the height of the main stem, the number of branches, and the length of the lateral stem in peanut seedlings. Meanwhile, allelochemical treatment also significantly decreased the dry weight of the stems and leaves in peanut seedlings (Table 1). Notably, high allelochemical treatment such as H2, C2, P2, and M2 induced higher reduction of these agronomic characteristics as compared with low allelochemical treatment. Particularly, plants treated with M2 displayed severe reduction in height of the main stem (38.4% in 2016 and 38.5% in 2017), number of branches (33.3% in 2016 and 35.3% in 2017), length of the lateral stem (36.3% in 2016 and 34.4% in 2017), dry weight of the stems (37.4% in 2016 and 40.3% in 2017), and dry weight of the leaves (42.5% in 2016 and 42.9% in 2017) when compared with those in control.
3.2 Effect of Allelochemical Treatment on Peanut Root Morphological Characters
To characterize the role of exogenous allelochemicals on peanut root growth characters, we first concentrated on the phenotypic changes that occurred in the peanut seedlings. In line with the effect of allelochemicals on agronomic characteristics, seedlings treated with H, C, P, and M showed significantly reduced total root length, root surface area, and root dry weight compared with the water-treated control (Table 2). It appears that the high concentration of allelochemicals (H2, C2, and P2) had stronger effect than the low concentration treatments on the reduction of these characters. Additionally, the mixture of these allelochemicals (M1 and M2) showed strongest inhibition of peanut root growth. By contrast, exogenous application of allelochemicals significantly increased the average root diameter especially in M1 and M2 treatments as evident by the increment of 20.9% and 30.2% in 2016 and 20.8% and 29.2% in 2017, respectively compared with CK.
3.3 Effect of Allelochemical Treatment on Activities of Antioxidant Enzymes and Oxidative Damage of Peanut Root
To investigate the role of allelochemical in the response of antioxidant system in peanut roots, we assessed the activities of SOD, POD, and CAT. The exogenous application of allelochemicals significantly induced the activities of SOD (Figs. 1A and 1E), POD (Figs. 1B and 1F), and CAT (Figs. 1C and 1G) in both 2016 and 2017. Interestingly, different concentrations of allelochemicals induced further increases in the activities of these enzymes at various degrees. Allelochemical treatment at 720 μg L−1 (H2, C2, P2, and M2) concentration showed the better effect in this regard than those treatment of 360 μg L−1 (H1, C1, P1, and M1) compared with CK. Likewise, the increment of activities of SOD, POD, and CAT in M2 was significantly higher than other treatments. In line with the data of antioxidant enzymes, exogenous application of allelochemicals induced lipid peroxidation in peanut roots, as evidenced by the significantly increased malondialdehyde (MDA) content in both 2016 and 2017 (Figs. 1D and 1H). The higher concentration of allelochemicals exhibited higher MDA content than the lower concentration treatments. M1 and M2 caused the greatest aggravation of membrane peroxidation resulting in the induction of MDA content by 53.6/76.1% in 2016 (Figs. 1D) and 75.5/93.3% in 2017 (Figs. 1H), respectively compared with CK in peanut roots.
Figure 1: Effects of allelochemical treatment on activities of the antioxidant enzymes and lipid peroxidation in peanut roots. Peanut seedlings at the four-leaf stage were treated with allelochemicals. Activities of SOD (A, E), POD (B, F), CAT (C, G), and content of MDA (D, H) in peanut roots were determined at 75 d after emergence. Data are the means (±SD) of three biological replicates. Different letters indicate significant differences according to Tukey’s test (P < 0.05)
3.4 Effect of Allelochemical Treatment on Activities of NR, ATPase, and Root System of Peanut Root
To understand the effects of allelochemical treatment on the enzymes of NR, ATPase, and root system, the time course changes of NR, ATPase, and root system were determined under allelochemical treatment. Exogenous application of H, C, and P significantly decreased NR activity at 45 d after emergence in both 2016 and 2017 (Figs. 2A and 2B). Then the activities of NR were gradually decreased in all the treatments at 75 and 105 d after emergence. Particularly, the NR activity in M2 was 40.8% in 2016 and 47.1% in 2017, respectively lower than the untreated seedlings of CK at 45 d after emergence and remained at the lowest value during the remaining period of the experiments in both 2016 and 2017. Similarly, the activities of ATPase (Figs. 2C and 2D) and root system (Figs. 2E and 2F) showed similar changes to NR activity where application of allelochemicals significantly reduced the activities of ATPase and root system at 45 d after emergence and decreased at 75 and 105 d after emergence. Again, M2 treatment exhibited the lowest activities of ATPase and root system during the whole period of the experiment in both years.
Figure 2: Time course changes of activities of NR (A, B), ATPase (C, D), and root system (E, F) post allelochemical treatment in peanut roots. Peanut seedlings at the four-leaf stage were treated with allelochemicals. Activities of NR, ATPase, and root system in peanut roots were determined at 45, 75, and 105 d after emergence. Data are the means (±SD) of three biological replicates
3.5 Effect of Allelochemical Treatment on Osmolytes Accumulation and Nutrient Content of Peanut Root
To determine the mechanism of the side effects of allelochemicals on peanut root, we analyzed the osmolytes accumulation involved in stress response. Treatment with H, C, P, and M significantly reduced the accumulation of osmolytes as evidenced by the decreased contents of total soluble sugar and total soluble protein in both 2016 and 2017 (Fig. 3). It is noteworthy that the magnitude of reduction for total soluble sugar and protein in low concentration of allelochemicals (H1, C2, P1, and M1) was augmented by high concentration of allelochemicals (H2, C2, P2, and M2). Allelochemical treatment led to evident reductions of total soluble sugar by 51.9, 50.8, 42.9, and 54.1% in 2016 (Fig. 3A) and 52.4, 51.4, 40.1, and 45.8% in 2017 (Fig. 3C), respectively in H2, C2, P2, and M2 seedlings compared with CK. Moreover, the allelochemical-induced reductions of total protein content were 49.1, 46.1, 49.8, and 50.2% in 2016 (Fig. 3B) and 50.8, 48.1 51.1 and 51.9% in 2017 (Fig. 3D), respectively in H2, C2, P2, and M2 seedlings compared with CK.
Figure 3: Effects of allelochemical treatment on osmolytes accumulation in peanut roots. Peanut seedlings at the four-leaf stage were treated with allelochemicals. Contents of total soluble sugar (A, C) and total soluble protein (B, D) in peanut roots were determined at 75 d after emergence. Data are the means (±SD) of three biological replicates. Different letters indicate significant differences according to Tukey’s test (P < 0.05)
Application of allelochemicals significantly inhibited the contents of nitrogen (N), phosphorus (P), and potassium (K) in peanut roots at 75 d after emergence (Fig. 4). The highest decline in N content was observed for M2 (28.7%), followed by M1 (23.6%), H2 (22.5%), and C2 (21.6%) compared with CK in 2016 (Fig. 4A). In 2017, the highest decline in N content was observed for M2 (22.8%), followed by C2 (22.6%), M1 (22.4%), and H2 (22.3%) compared with CK (Fig. 4B). In line with N content, similar results were obtained in the contents of P and K where the highest decline was observed in M2 by 33.9% (Fig. 4C) and 33.8% (Fig. 4E) in 2016 and 35.9% (Fig. 4D) and 34.0% in 2017 (Fig. 4F) of P content and K content, respectively compared with CK. Notably, the dosage effect of allelochemicals were also detected where application of 720 μg L−1 allelochemicals exhibited more reduction in the contents of N, P, and K than those of 360 μg L−1 compared with untreated control seedlings in both 2016 and 2017.
Figure 4: Effects of allelochemical treatment on the nutrient content in peanut roots. Peanut seedlings at the four-leaf stage were treated with allelochemicals. Contents of total nitrogen (A, B), total phosphorus (C, D), and total potassium (E, F) in peanut roots were determined at 75 d after emergence. Data are the means (±SD) of three biological replicates. Different letters indicate significant differences according to Tukey’s test (P < 0.05)
3.6 Effect of Allelochemical Treatment on Pod Yield and Yield Components of Peanut
The dry fruit weight per pot was significantly decreased by the 360 μg L−1 of allelochemical treatment in H1, C1, and P1. The reduction of dry fruit weight per pot was aggravated when the concentration was higher in H2, C2, and P2. Again, the reduction was more pronounced in M1 and M2 as evidenced by a decline of 42.4% and 49.6% in 2016, and 43.0% and 49.7% in 2017, respectively compared with CK (Table 3). Similar results were obtained in the yield components data where allelochemical treatment significantly decreased the pod number per plant, 100-pod weight, and full fruit rate while significantly increased the pod number per kilogram. Notably, the dosage effect still exists that high concentration of allelochemicals (H2, C2, and P2) showed more impact on the yield components than the low concentration treatments (H1, C1, and P1). Moreover, application of the mixture of allelochemicals (M1 and M2) have been shown to be the most severe treatments that impacted by allelochemicals where the pod number per plant was reduced by 46.1% and 50.7% in 2016, and 47.7% and 50.6% in 2017.The 100-pod weight was reduced by 10.3% and 11.4% in 2016, and 10.6% and 12.0% in 2017. The full fruit rate was reduced by 31.8% and 40.8% in 2016 and 31.5% and 41.7% in 2017 in M1 and M2, respectively compared with CK. However, the pod number per kilogram was induced by 38.7% and 53.7% in 2016 and 41.0% and 52.5% in 2017 in M1 and M2, respectively compared with CK.
This study elucidated some unique functions of allelochemicals in response to CC obstacle in peanut, an important oil seed crop worldwide. We noticed that plant growth inhibition could be induced by both high (720 μg L−1) and low (360 μg L−1) concentrations of allelochemicals (Table 1). Notably, exogenous application of allelochemicals H, C, P, and their mixture M significantly decreased the plant growth of both aboveground part and underground part as evidenced by the reduced plant height, number of branches, dry weight of stems and leaves, and root morphological characters (Tables 1 and 2). Meanwhile, allelochemicals also aggravated the membrane peroxidation even though the activities of antioxidant enzymes were induced (Fig. 1). Besides, allelochemicals restricted the uptake of root nutrition of N, P, K, and the accumulation of osmolytes such as soluble sugar and protein (Figs. 3 and 4). Ultimately, the application of allelochemicals reduced the pod yield of peanut plants (Table 3). We therefore propose that allelochemicals play a crucial role in CC obstacle induced peanut growth inhibition and yield reduction. The findings of the present study could provide novel information about the long-term monoculture from the perspective of allelochemicals.
Phenolic acids such as p-hydroxybenzoic acid, cinnamic acid, and phthalic acid used in this study have been reported to have a commercial value for human health due to their antiaging, antitumor, and antiinflammatory properties [48–52]. As major components of allelochemicals, phenolic acids always induce changes in soil microbial properties and enzymatic activity [53] and act as signaling molecules in plant-microorganism interactions [54], however, their functions in CC obstacle were less defined. As shown in Table 1, exogenous application of phenolic acids showed negative effects on peanut growth especially the underground part of the plants. In peanut production, CC obstacle has been considered as the major limitation of plant growth and yield formation. These results hinting a possibility that application of phenolic acids resembles that of CC obstacle, namely, CC obstacle induced allelochemicals especially phenolic acids play a dominant role in the inhibition of peanut root growth.
The induction of the activities of antioxidant enzymes such as SOD, POD, and CAT are demonstrated to be important for scavenging reactive oxygen species (ROS) [55–57]. In the present study, exposure of peanut seedlings to both low and high concentrations of allelochemicals induced substantial increases in activities of SOD, POD, and CAT (Fig. 1). However, such increase in activities of antioxidant enzymes was unable to ameliorate oxidative stress possibly due to insufficient ROS scavenging ability in parallel with allelochemical-induced ROS generation as characterized by the allelochemical-induced the membrane peroxidation (MDA accumulation) in peanut roots (Figs. 1D and 1H). We therefore speculate that the induction of antioxidant systems by allelochemicals may indicate an adaptive response by peanut as allelochemicals are well-recognized as signal molecules in response to plant environmental stress [58–60], which warrant further investigation. These observations confirmed that the antioxidant systems stimulated by exogenous allelochemicals were insufficient to confer CC obstacle induced peanut root inhibition.
The metabolisms of mineral nutrients such as N, P, and K, as well as osmolytes such as soluble sugar and soluble protein reveal the plant growing potential and root activity. Moreover, the composition of mineral nutrients and osmolytes may function as indicators for the assessment of the plant physiological parameters [61–63]. It is well accepted that the synthesis of soluble sugar and soluble protein could be partially attributed to the activities of root NR and ATPase for their functions in assimilation and transformation of N in plant roots [64–67]. NR catalyses the transfer of two electrons from NAD(P)H to nitrate to produce nitrite [68] while ATPase plays a key role in the maintenance of optimum photosynthetic rate and ensuring effective energy flow for crop growth [69]. In this paper, the reductions of NR activity and ATPase activity (Figs. 2A–2D) were observed under allelochemical treatments, which were consistent with the changes of total soluble sugar and protein content (Fig. 3), indicating that NR and ATPase play crucial roles in the uptake of mineral nutrients by peanut roots under CC obstacle. In line with the osmolytes data, the performed analysis exhibited a dramatic decline in dry weight of shoots and roots under allelochemical treatments (Table 1), suggesting that application of allelochemicals reduced the capacity of synthesis and transportation of carbohydrates through decreasing the content of total soluble sugar in peanut plants. The data of dried fruit weight per pot further pointing out that allelochemicals showed negative effects on peanut pod yield formation (Table 3). Consequently, the inhibited root physiological traits weakened the absorption of nitrogen and induced early senescence, which ultimately decreased the pod yield of peanut. A similar set of observations was made in the yield components where pod No. per plant, 100-pod weight, and full fruit rate were significantly reduced by the application of allelochemicals (Table 3). In line with the previous studies, the yield and yield components decreases for these treatments were possibly due to aggravating of CC obstacle as a result of positioned rotation [47,70].
In summary, our study has concentrated on addressing the allelochemical-induced peanut growth response and feedback to the pod yield under CC obstacle. Application of allelochemicals manifested remarkable inhibitions to peanut plants in terms of seedling growth, antioxidant system, osmolytes accumulation, and root activity. Moreover, the decreased nutrition absorption by roots might contribute to the peanut yield reduction (Fig. 5). The findings of this study provide new clues that could help to develop new strategies to combat CC obstacle in legume crops production through reducing the accumulation of allelochemicals. Further researches should be taken under field conditions to obtain practical conclusions in support of the involvement of allelochemicals in CC obstacle of legume crops.
Figure 5: The working model illustrating the mechanisms of allelochemicals in reducing peanut yield
Acknowledgement: We thank Dr. Dunwei Ci from Shandong Peanut Research Institute for the technical assistance.
Authorship: The authors confirm contribution to the paper as follows: study conception and design: Zhaohui Tang, Shubo Wan; data collection: Zhaohui Tang, Feng Guo, Li Cui; analysis and interpretation of results: Zhaohui Tang, Qingkai Li, Jialei Zhang, Jianguo Wang; draft manuscript preparation: Zhaohui Tang, Sha Yang, Jingjing Meng, Xinguo Li, Ping Liu. All authors reviewed the results and approved the final version of the manuscript.
Funding Statement: This work was financially supported by the National Key R&D Program of China (2018YFD1000902) and the Natural Science Foundation of Shandong Province (ZR2021QC163).
Conflicts of Interest: The authors declare that they have no conflicts of interest to report regarding the present study.
References
1. Kakraliya, S. K., Singh, U., Bohra, A., Choudhary, K. K., Kumar, S. et al. (2018). Nitrogen and legumes: A meta-analysis. Singapore: Springer. DOI 10.1007/978-981-13-0253-4_9. [Google Scholar]
2. Stagnari, F., Maggio, A., Galieni, A., Pisante, M. (2017). Multiple benefits of legumes for agriculture sustainability: An overview. Chemical and Biological Technologies in Agriculture, 4(1), 2. DOI 10.1186/s40538-016-0085-1. [Google Scholar] [CrossRef]
3. Tian, S., Guo, R., Zou, X., Zhang, X., Yu, X. et al. (2019). Priming with the green leaf volatile (Z)-3-Hexeny-1-yl Acetate enhances salinity stress tolerance in peanut (Arachis hypogaea L.) seedlings. Frontiers in Plant Science, 10, 785. DOI 10.3389/fpls.2019.00785. [Google Scholar] [CrossRef]
4. Guo, R., Yan, H., Li, X., Zou, X., Zhang, X. et al. (2020). Green leaf volatile (Z)-3-hexeny-1-yl acetate reduces salt stress in peanut by affecting photosynthesis and cellular redox homeostasis. Physiologia Plantarum, 170(1), 75–92. DOI 10.1111/ppl.13110. [Google Scholar] [CrossRef]
5. Considine, M. J., Siddique, K. H. M., Foyer, C. H. (2017). Nature’s pulse power: Legumes, food security and climate change. Journal of Experimental Botany, 68(8), 1815–1818. DOI 10.1093/jxb/erx099. [Google Scholar] [CrossRef]
6. Zhang, X., Chen, N., Sheng, H., Ip, C., Yang, L. et al. (2019). Urban drought challenge to 2030 sustainable development goals. Science of the Total Environment, 693(2–4), 133536. DOI 10.1016/j.scitotenv.2019.07.342. [Google Scholar] [CrossRef]
7. Xie, X. G., Zhang, F. M., Wang, X. X., Li, X. G., Dai, C. C. (2019). Phomopsis liquidambari colonization promotes continuous cropping peanut growth by improving the rhizosphere microenvironment, nutrient uptake and disease incidence. Journal of the Science of Food and Agriculture, 99(4), 1898–1907. DOI 10.1002/jsfa.9385. [Google Scholar] [CrossRef]
8. Chen, S., Qi, G., Luo, T., Zhang, H., Jiang, Q. et al. (2018). Continuous-cropping tobacco caused variance of chemical properties and structure of bacterial network in soils. Land Degradation & Development, 29(11), 4106–4120. DOI 10.1002/ldr.3167. [Google Scholar] [CrossRef]
9. Jordan, D. L., Corbett, T., Bogle, C., Shew, B., Brandenburg, R. et al. (2017). Effect of previous rotation on plant parasitic nematode population in peanut and crop yield. Crop, Forage & Turfgrass Management, 3(1), 1–7. DOI 10.2134/cftm2016.12.0086. [Google Scholar] [CrossRef]
10. Chen, P., Wang, Y., Liu, Q., Zhang, Y., Li, X. et al. (2020). Phase changes of continuous cropping obstacles in strawberry (Fragaria × ananassa Duch.) production. Applied Soil Ecology, 155(1–3), 103626. DOI 10.1016/j.apsoil.2020.103626. [Google Scholar] [CrossRef]
11. Aparicio, V., Costa, J. L. (2007). Soil quality indicators under continuous cropping systems in the Argentinean Pampas. Soil and Tillage Research, 96(1), 155–165. DOI 10.1016/j.still.2007.05.006. [Google Scholar] [CrossRef]
12. Liu, Q., Wang, S., Li, K., Qiao, J., Guo, Y. et al. (2021). Responses of soil bacterial and fungal communities to the long-term monoculture of grapevine. Applied Microbiology and Biotechnology, 105(18), 7035–7050. DOI 10.1007/s00253-021-11542-1. [Google Scholar] [CrossRef]
13. Xiong, W., Zhao, Q., Zhao, J., Xun, W., Li, R. et al. (2015). Different continuous cropping spans significantly affect microbial community membership and structure in a vanilla-grown soil as revealed by deep pyrosequencing. Microbial Ecology, 70(1), 209–218. DOI 10.1007/s00248-014-0516-0. [Google Scholar] [CrossRef]
14. Tan, Y., Cui, Y., Li, H., Kuang, A., Li, X. et al. (2017). Rhizospheric soil and root endogenous fungal diversity and composition in response to continuous Panax notoginseng cropping practices. Microbiological Research, 194, 10–19. DOI 10.1016/j.micres.2016.09.009. [Google Scholar] [CrossRef]
15. Zeng, J., Liu, J., Lu, C., Ou, X., Luo, K. et al. (2020). Intercropping with turmeric or ginger reduce the continuous cropping obstacles that affect Pogostemon cablin (Patchouli). Frontiers in Microbiology, 11, 2526. DOI 10.3389/fmicb.2020.579719. [Google Scholar] [CrossRef]
16. Ma, H., Yang, B., Wang, H., Yang, Q., Dai, C. (2016). Application of Serratia marcescens RZ-21 significantly enhances peanut yield and remediates continuously cropped peanut soil. Journal of the Science of Food and Agriculture, 96(1), 245–253. DOI 10.1002/jsfa.7087. [Google Scholar] [CrossRef]
17. Lewis, K. L., DeLaune, P., Keeling, W. (2017). Securing our soil in intensive monoculture cropping systems. In: Global soil security, progress in soil science. Cham: Springer.DOI 10.1007/978-3-319-43394-3_13. [Google Scholar] [CrossRef]
18. Zhao, F., Zhang, Y., Dong, W., Zhang, Y., Zhang, G. et al. (2019). Vermicompost can suppress Fusarium oxysporum f. sp. lycopersici via generation of beneficial bacteria in a long-term tomato monoculture soil. Plant and Soil, 440(1), 491–505. DOI 10.1007/s11104-019-04104-y. [Google Scholar] [CrossRef]
19. Wang, H., Sun, K., Guan, Y., Qiu, M., Zhang, L. et al. (2019). Fungal endophyte Phomopsis liquidambari biodegrades soil resveratrol: A potential allelochemical in peanut monocropping systems. Journal of the Science of Food and Agriculture, 99(13), 5899–5909. DOI 10.1002/jsfa.9865. [Google Scholar] [CrossRef]
20. Li, Z., Xiong, F., Guo, W., Mo, C., Wu, H. et al. (2020). The root transcriptome analyses of peanut wild species Arachis correntina (Burkart) Krapov. & W.C. Gregory and cultivated variety Xiaobaisha in response to benzoic acid and p-cumaric acid stress. Genetic Resources and Crop Evolution, 67(1), 9–20. DOI 10.1007/s10722-019-00859-6. [Google Scholar] [CrossRef]
21. Shao, S., Chen, M., Liu, W., Hu, X., Wang, E. T. et al. (2020). Long-term monoculture reduces the symbiotic rhizobial biodiversity of peanut. Systematic and Applied Microbiology, 43(5), 126101. DOI 10.1016/j.syapm.2020.126101. [Google Scholar] [CrossRef]
22. Batish, D. R., Setia, N., Singh, H. P., Kohli, R. K. (2004). Phytotoxicity of lemon-scented eucalypt oil and its potential use as a bioherbicide. Crop Protection, 23(12), 1209–1214. DOI 10.1016/j.cropro.2004.05.009. [Google Scholar] [CrossRef]
23. Blaise, D., Manikandan, A., Verma, P., Nalayini, P., Chakraborty, M. et al. (2020). Allelopathic intercrops and its mulch as an integrated weed management strategy for rainfed Bt-transgenic cotton hybrids. Crop Protection, 135(2), 105214. DOI 10.1016/j.cropro.2020.105214. [Google Scholar] [CrossRef]
24. Głąb, L., Sowiński, J., Bough, R., Dayan, F. E. (2017). Chapter Two-allelopathic potential of Sorghum (Sorghum bicolor (L.) Moench) in weed control: A comprehensive review. Advances in Agronomy. DOI 10.1016/bs.agron.2017.05.001. [Google Scholar] [CrossRef]
25. Hoang Anh, L., van Quan, N., Tuan Nghia, L., Xuan, T. D. (2021). Phenolic allelochemicals: Achievements, limitations, and prospective approaches in weed management. Weed Biology and Management, 21(2), 37–67. DOI 10.1111/wbm.12230. [Google Scholar] [CrossRef]
26. Sitthinoi, P., Lertmongkol, S., Chanprasert, W., Vajrodaya, S. (2017). Allelopathic effects of jungle rice (Echinochloa colona (L.)Link) extract on seed germination and seedling growth of rice. Agriculture and Natural Resources, 51(2), 74–78. DOI 10.1016/j.anres.2016.09.004. [Google Scholar] [CrossRef]
27. Gulzar, A., Siddiqui, M. B. (2015). Root-mediated allelopathic interference of bhringraj (Eclipta alba L.) Hassk. on peanut (Arachis hypogaea) and mung bean (Vigna radiata). Applied Soil Ecology, 87, 72–80. DOI 10.1016/j.apsoil.2014.11.001. [Google Scholar] [CrossRef]
28. Li, X., Ding, C., Hua, K., Zhang, T., Zhang, Y. et al. (2014). Soil sickness of peanuts is attributable to modifications in soil microbes induced by peanut root exudates rather than to direct allelopathy. Soil Biology and Biochemistry, 78, 149–159. DOI 10.1016/j.soilbio.2014.07.019. [Google Scholar] [CrossRef]
29. Sánchez-Navarro, V., Zornoza, R., Faz, Á., Fernández, J. A. (2019). Comparing legumes for use in multiple cropping to enhance soil organic carbon, soil fertility, aggregates stability and vegetables yields under semi-arid conditions. Scientia Horticulturae, 246, 835–841. DOI 10.1016/j.scienta.2018.11.065. [Google Scholar] [CrossRef]
30. da Silva Rodrigues-Corrêa, K. C., Halmenschlager, G., Schwambach, J., de Costa, F., Mezzomo-Trevizan, E. et al. (2017). Dual allelopathic effects of subtropical slash pine (Pinus elliottii Engelm.) needles: Leads for using a large biomass reservoir. Industrial Crops and Products, 108(10), 113–120. DOI 10.1016/j.indcrop.2017.06.019. [Google Scholar] [CrossRef]
31. Liu, P., Zhao, H., Tang, Z., Zhang, Y., Lin, H. et al. (2015). Effects of continuous cropping on root exudates of different resistance peanut (Arachis hypogaea L.) varieties and allelochemicals content in soil. Chinese Journal of Oil Crop Sciences, 37(4), 467–474 (in Chinese). DOI 10.7505/j.issn.1007-9084.2015.04.006. [Google Scholar]
32. Qin, W., Yan, H., Zou, B., Guo, R., Ci, D. et al. (2021). Arbuscular mycorrhizal fungi alleviate salinity stress in peanut: Evidence from pot-grown and field experiments. Food and Energy Security, 10(4), e314. DOI 10.1002/fes3.314. [Google Scholar] [CrossRef]
33. Bradford, M. M. (1976). Rapid and sensitive method for quantitation of microgram quantities of protein utilizing principle of protein-dye binding. Analytical Biochemistry, 72(1–2), 248–254. DOI 10.1016/0003-2697(76)90527-3. [Google Scholar] [CrossRef]
34. Stewart, R. R. C., Bewley, J. D. (1980). Lipid peroxidation associated with accelerated aging of soybean axes. Plant Physiology, 65(2), 245–248. DOI 10.1104/pp.65.2.245. [Google Scholar] [CrossRef]
35. Cakmak, I., Marschner, H. (1992). Magnesium deficiency and high light intensity enhance activities of superoxide dismutase, ascorbate peroxidase, and glutathione reductase in bean leaves. Plant Physiology, 98(4), 1222–1227. DOI 10.1104/pp.98.4.1222. [Google Scholar] [CrossRef]
36. Patra, H. K., Kar, M., Mishra, D. (1978). Catalase activity in leaves and cotyledons during plant development and senescence. Biochemie und Physiologie der Pflanzen, 172(4), 385–390. DOI 10.1016/S0015-3796(17)30412-2. [Google Scholar] [CrossRef]
37. Hodges, D. M., DeLong, J. M., Forney, C. F., Prange, R. K. (1999). Improving the thiobarbituric acid-reactive-substances assay for estimating lipid peroxidation in plant tissues containing anthocyanin and other interfering compounds. Planta, 207(6), 604–611. DOI 10.1007/s00425-017-2699-3. [Google Scholar] [CrossRef]
38. Jiang, S., Sun, J., Tian, Z., Hu, H., Michel, E. J. S. et al. (2017). Root extension and nitrate transporter up-regulation induced by nitrogen deficiency improves nitrogen status and plant growth at the seedling stage of winter wheat (Triticum aestivum L.). Environmental and Experimental Botany, 141, 28–40. DOI 10.1016/j.envexpbot.2017.06.006. [Google Scholar] [CrossRef]
39. Ruf, M., Brunner, I. (2003). Vitality of tree fine roots: reevaluation of the tetrazolium test. Tree Physiology, 23(4), 257–263. DOI 10.1093/treephys/23.4.257. [Google Scholar] [CrossRef]
40. Begum, N., Ahanger, M. A., Zhang, L. (2020). AMF inoculation and phosphorus supplementation alleviates drought induced growth and photosynthetic decline in Nicotiana tabacum by up-regulating antioxidant metabolism and osmolyte accumulation. Environmental and Experimental Botany, 176(9), 104088. DOI 10.1016/j.envexpbot.2020.104088. [Google Scholar] [CrossRef]
41. Scheible, W. R., Lauerer, M., Schulze, E. D., Caboche, M., Stitt, M. (1997). Accumulation of nitrate in the shoot acts as a signal to regulate shoot-root allocation in tobacco. Plant Journal, 11(4), 671–691. DOI 10.1046/j.1365-313X.1997.11040671.x. [Google Scholar] [CrossRef]
42. Requena, N., Breuninger, M., Franken, P., Ocón, A. (2003). Symbiotic status, phosphate, and sucrose regulate the expression of two plasma membrane H+-ATPase genes from the Mycorrhizal Fungus Glomus mosseae. Plant Physiology, 132(3), 1540–1549. DOI 10.1104/pp.102.019042. [Google Scholar] [CrossRef]
43. Galtier, N., Belver, A., Gibrat, R., Grouzis, J. P., Rigaud, J. et al. (1988). Preparation of corn root plasmalemma with low Mg-ATPase latency and high electrogenic H+ pumping activity after phase partitioning. Plant Physiology, 87(2), 491–497. DOI 10.1104/pp.87.2.491. [Google Scholar] [CrossRef]
44. Buysse, J. A. N., Merckx, R. (1993). An improved colorimetric method to quantify sugar content of plant tissue. Journal of Experimental Botany, 44(10), 1627–1629. DOI 10.1093/jxb/44.10.1627. [Google Scholar] [CrossRef]
45. Si, T., Wang, X., Zhao, C., Huang, M., Cai, J. et al. (2018). The role of hydrogen peroxide in mediating the mechanical wounding-induced freezing tolerance in wheat. Frontiers in Plant Science, 9, 327. DOI 10.3389/fpls.2018.00327. [Google Scholar] [CrossRef]
46. Aljazairi, S., Arias, C., Nogués, S. (2015). Carbon and nitrogen allocation and partitioning in traditional and modern wheat genotypes under pre-industrial and future CO2 conditions. Plant Biology, 17(3), 647–659. DOI 10.1111/plb.12280. [Google Scholar] [CrossRef]
47. Chi, B., Zhang, Y., Zhang, D., Zhang, X., Dai, J. et al. (2019). Wide-strip intercropping of cotton and peanut combined with strip rotation increases crop productivity and economic returns. Field Crops Research, 243(4954), 107617. DOI 10.1016/j.fcr.2019.107617. [Google Scholar] [CrossRef]
48. Yener, I., Kocakaya, S. O., Ertas, A., Erhan, B., Kaplaner, E. et al. (2020). Selective in vitro and in silico enzymes inhibitory activities of phenolic acids and flavonoids of food plants: Relations with oxidative stress. Food Chemistry, 327(1), 127045. DOI 10.1016/j.foodchem.2020.127045. [Google Scholar] [CrossRef]
49. Al Jitan, S., Alkhoori, S. A., Yousef, L. F. (2018). Phenolic acids from plants: Extraction and application to human health. Studies in Natural Products Chemistry, 58, 389–417. DOI 10.1016/B978-0-444-64056-7.00013-1. [Google Scholar] [CrossRef]
50. Proestos, C., Boziaris, I. S., Nychas, G. J. E., Komaitis, M. (2006). Analysis of flavonoids and phenolic acids in Greek aromatic plants: Investigation of their antioxidant capacity and antimicrobial activity. Food Chemistry, 95(4), 664–671. DOI 10.1016/j.foodchem.2005.01.049. [Google Scholar] [CrossRef]
51. Zhang, B., Zhang, Y., Li, H., Deng, Z., Tsao, R. (2020). A review on insoluble-bound phenolics in plant-based food matrix and their contribution to human health with future perspectives. Trends in Food Science & Technology, 105, 347–362. DOI 10.1016/j.tifs.2020.09.029. [Google Scholar] [CrossRef]
52. Zhang, X., Chen, N., Li, J., Chen, Z., Niyogi, D. (2017). Multi-sensor integrated framework and index for agricultural drought monitoring. Remote Sensing of Environment, 188, 141–163. DOI 10.1016/j.rse.2016.10.045. [Google Scholar] [CrossRef]
53. Qu, X. H., Wang, J. G. (2008). Effect of amendments with different phenolic acids on soil microbial biomass, activity, and community diversity. Applied Soil Ecology, 39(2), 172–179. DOI 10.1016/j.apsoil.2007.12.007. [Google Scholar] [CrossRef]
54. Stanek, M., Zubek, S., Stefanowicz, A. M. (2021). Differences in phenolics produced by invasive Quercus rubra and native plant communities induced changes in soil microbial properties and enzymatic activity. Forest Ecology and Management, 482, 118901. DOI 10.1016/j.foreco.2020.118901. [Google Scholar] [CrossRef]
55. Suzuki, N., Koussevitzky, S., Mittler, R., Miller, G. (2012). ROS and redox signalling in the response of plants to abiotic stress. Plant, Cell & Environment, 35(2), 259–270. DOI 10.1111/j.1365-3040.2011.02336.x. [Google Scholar] [CrossRef]
56. Miller, G., Shulaev, V., Mittler, R. (2008). Reactive oxygen signaling and abiotic stress. Physiologia Plantarum, 133(3), 481–489. DOI 10.1111/j.1399-3054.2008.01090.x. [Google Scholar] [CrossRef]
57. Choudhury, F. K., Rivero, R. M., Blumwald, E., Mittler, R. (2017). Reactive oxygen species, abiotic stress and stress combination. Plant Journal, 90(5), 856–867. DOI 10.1111/tpj.13299. [Google Scholar] [CrossRef]
58. Yoneyama, K., Natsume, M. (2013). Allelochemicals for plant-plant and plant-microbe interactions. Reference Module in Chemistry, Molecular Sciences and Chemical Engineering, Elsevier, 4, 539–561. DOI 10.1016/B978-0-12-409547-2.02802-X. [Google Scholar] [CrossRef]
59. Zhang, Y., Wang, J., Tan, L. (2019). Characterization of allelochemicals of the diatom Chaetoceros curvisetus and the effects on the growth of Skeletonema costatum. Science of the Total Environment, 660(1798), 269–276. DOI 10.1016/j.scitotenv.2019.01.056. [Google Scholar] [CrossRef]
60. Inderjit, Weiner, J. (2001). Plant allelochemical interference or soil chemical ecology? Perspectives in Plant Ecology, Evolution and Systematics, 4(1), 3–12. DOI 10.1078/1433-8319-00011. [Google Scholar] [CrossRef]
61. Liang, X., Guo, F., Feng, Y., Zhang, J., Yang, S. et al. (2020). Single-seed sowing increased pod yield at a reduced seeding rate by improving root physiological state of Arachis hypogaea. Journal of Integrative Agriculture, 19(4), 1019–1032. DOI 10.1016/S2095-3119(19)62712-7. [Google Scholar] [CrossRef]
62. Noguchi, A., Kageyama, M., Shinmachi, F., Schmidhalter, U., Hasegawa, I. (2005). Potential for using plant xylem sap to evaluate inorganic nutrient availability in soil. Soil Science and Plant Nutrition, 51(3), 333–341. DOI 10.1111/j.1747-0765.2005.tb00038.x. [Google Scholar] [CrossRef]
63. Li, R., Chen, N., Zhang, X., Zeng, L., Wang, X. et al. (2020). Quantitative analysis of agricultural drought propagation process in the Yangtze River Basin by using cross wavelet analysis and spatial autocorrelation. Agricultural and Forest Meteorology, 280(2), 107809. DOI 10.1016/j.agrformet.2019.107809. [Google Scholar] [CrossRef]
64. Torabian, S., Farhangi-Abriz, S., Rathjen, J. (2018). Biochar and lignite affect H+-ATPase and H+-PPase activities in root tonoplast and nutrient contents of mung bean under salt stress. Plant Physiology and Biochemistry, 129, 141–149. DOI 10.1016/j.plaphy.2018.05.030. [Google Scholar] [CrossRef]
65. Carr, N. F., Boaretto, R. M., Mattos, D. (2020). Coffee seedlings growth under varied NO3−: NH4+ ratio: Consequences for nitrogen metabolism, amino acids profile, and regulation of plasma membrane H+-ATPase. Plant Physiology and Biochemistry, 154(5), 11–20. DOI 10.1016/j.plaphy.2020.04.042. [Google Scholar] [CrossRef]
66. Sperandio, M. V. L., Santos, L. A., Bucher, C. A., Fernandes, M. S., de Souza, S. R. (2011). Isoforms of plasma membrane H+-ATPase in rice root and shoot are differentially induced by starvation and resupply of NO3− or NH4+. Plant Science, 180(2), 251–258. DOI 10.1016/j.plantsci.2010.08.018. [Google Scholar] [CrossRef]
67. Cabañero, F. J., Carvajal, M. (2007). Different cation stresses affect specifically osmotic root hydraulic conductance, involving aquaporins, ATPase and xylem loading of ions in Capsicum annuum L. plants. Journal of Plant Physiology, 164(10), 1300–1310. DOI 10.1016/j.jplph.2006.08.010. [Google Scholar] [CrossRef]
68. Kaiser, W. M., Weiner, H., Huber, S. C. (1999). Nitrate reductase in higher plants: A case study for transduction of environmental stimuli into control of catalytic activity. Physiologia Plantarum, 105(2), 384–389. DOI 10.1034/j.1399-3054.1999.105225.x. [Google Scholar] [CrossRef]
69. Ye, Z. P., Robakowski, P., Suggett, D. J. (2013). A mechanistic model for the light response of photosynthetic electron transport rate based on light harvesting properties of photosynthetic pigment molecules. Planta, 237(3), 837–847. DOI 10.1007/s00425-012-1790-z. [Google Scholar] [CrossRef]
70. Johnson, A. W., Dowler, C. C., Handoo, Z. A. (2000). Population dynamics of Meloidogyne incognita, M. arenaria, and other nematodes and crop yields in rotations of cotton, peanut, and wheat under ninimum tillage. Journal of Nematology, 32(1), 52–61. DOI 10.1063/1.3117032. [Google Scholar] [CrossRef]
Cite This Article
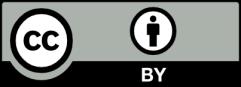