Open Access
REVIEW
Transcriptional factor RUNX1: A potential therapeutic target for fibrotic pulmonary disease
1 Department of Pulmonary and Critical Care Medicine, West China Hospital, Sichuan University, Chengdu, 610041, China
2 Laboratory of Pulmonary Immunology and Inflammation, Department of Respiratory and Critical Care Medicine, Frontiers Science Center for Disease-Related Molecular Network, West China Hospital, Sichuan University, Chengdu, 610041, China
3 International Medical Center, General Practice Unit, West China Hospital, Sichuan University, Chengdu, 610041, China
* Corresponding Author: FENGMING LUO. Email:
# These authors contributed equally to this article
(This article belongs to the Special Issue: Bioinformatics Study of Diseases)
BIOCELL 2023, 47(4), 697-705. https://doi.org/10.32604/biocell.2023.026148
Received 19 August 2022; Accepted 25 November 2022; Issue published 08 March 2023
Abstract
Runt-related transcription factor-1 (RUNX1), also known as the core-binding factor alpha 2 subunit, is closely related to human leukemia. The functions of RUNX1 in modulating cell proliferation, differentiation, and survival in multiple systems have been gradually discovered with the emergence of transgenic mice. RUNX1 is a powerful transcription factor implicated in diverse signaling pathways and cellular mechanisms that participate in lung development and pulmonary diseases. RUNX1 has recently been identified as a target regulator of fibrotic remodeling diseases, particularly in the kidney. However, the role of RUNX1 in pulmonary fibrosis is unclear. Pulmonary fibrosis is characterized by obscure nosogenesis, limited therapy, and poor prognosis. Moreover, the population of patients with pulmonary fibrosis is gradually increasing. Thus, there is an unmet need for therapeutic targets. In this review, we retrospectively discuss the alteration in RUNX1 mRNA expression in the RNA sequencing data of human fibrotic lungs and the protein levels in mouse pulmonary fibrosis. Subsequently, we focused on the interaction between RUNX1 and several signaling pathways involved in pulmonary fibrosis. Finally, this review highlights the therapeutic potential of RUNX1 as a target for slowing the progression of fibrotic lung disease.Keywords
Pulmonary fibrosis, a common lethal lung disease, is characterized by abnormal scarring of lung parenchyma. Pulmonary fibrosis has a poor prognosis, rising morbidity rates, and limited available therapeutic options. Ban et al. (2018) reported an increase from 12 to 532 (2000–2012) in the number of patients newly diagnosed with interstitial lung disease (ILD) in their study cohort. Another study revealed that in the United States, the prevalence of idiopathic pulmonary fibrosis (IPF) is twice as high as that reported ten years ago (Lederer and Martinez, 2018). Currently, only two inhibitors have been approved for the treatment of pulmonary fibrosis: (a) nintedanib, a tyrosine kinase inhibitor, and (b) pirfenidone, a cytokine suppressor (Karimi-Shah and Chowdhury, 2015). Large-scale clinical trials have shown that nintedanib and pirfenidone slow down the decline in the forced vital capacity of the lungs (Vancheri et al., 2018; Moor et al., 2020; Flaherty et al., 2018; Richeldi et al., 2011; Richeldi et al., 2014; King et al., 2014; Noble et al., 2011). Lung transplantation is the ultimate treatment option for pulmonary fibrosis. Regrettably, the median survival was barely 4.7 years for 9541 patients who received lung transplantation surgery for their fibrotic lungs (Yusen et al., 2014). Therefore, there is a long-term need for the identification of more therapeutic targets to promote a better prognosis for fibrosis.
The fibrotic process involves a complex series of alterations in protein and transcription levels, signaling pathways, and structural remodeling. Fibrosis is accompanied by extracellular matrix over-deposition, fibroblast proliferation, differentiation, impaired repair ability of alveolar epithelial cells, endothelial dysfunction, cell senescence, mitochondrial injury, and shortened telomerase (Wynn and Ramalingam, 2012). In fibrotic tissues, abnormal activation or inhibition of certain pathways’ activities have been observed; these pathways include TGF-β (Ikawa et al., 2008; Kunz et al., 2004), NF-κB (Wang et al., 2021; Peng et al., 2020; Pan et al., 2020), Wnt/β-catenin (Brack et al., 2007; Lee et al., 2020; Gay et al., 2020), and PI3K-Akt signaling (Hsu et al., 2017; Wu et al., 2017), as well as morphogenetic development-related pathways (Edeling et al., 2016; Chanda et al., 2019) such as Notch, Hippo, and Hedgehog signaling pathways. Runt-related transcription factor-1 (RUNX1) was discovered to be a novel and important regulator of the kidney (Zhou et al., 2018; Cheng et al., 2020), heart (Kuppe et al., 2020; Li et al., 2021b), and skin fibrosis (Abbasi et al., 2020). RUNX1 is essential for fibroblast proliferation and differentiation (Kim et al., 2014; Dubey et al., 2022). A recent study has suggested that RUNX1 is closely associated with pulmonary fibrosis (O’Hare et al., 2021). Accordingly, we speculated that RUNX1 might be a potential therapeutic target for pulmonary fibrosis.
Targeting transcriptional factors (TFs) to intervene in several aberrant signaling pathways in fibrotic tissue appears to be a powerful method for treating fibrosis (Bradner et al., 2017; Loft et al., 2021). TFs regulate gene expression, and one TF usually mediates the transcription of hundreds of target genes (Mevel et al., 2019). This feature enables them to modulate multiple signaling pathways and biological processes. Targeting promising TFs that influence multiple pathways involved in fibrosis is expected to slow the progression of over-deposition of the extracellular matrix, and structural remodeling. Hence, we reviewed and summarized the connection between the multifunctional TF RUNX1 and fibrotic pulmonary disease to provide a new perspective for clinical work and scientific research.
Structure and Function of RUNX1
RUNX1 belongs to the runt-related family that participates in proliferation, differentiation, and cell survival in various cell lineages (Hsu et al., 2020; Li et al., 2021a; Tang et al., 2021). RUNX1 encodes the alpha subunit of the core-binding transcription factor. The RUNX1 protein consists of three domains: the runt homology domain (RHD) within the N-terminal region, C-terminal transactivation domain (TAD), and repression domain (RD) (Tang et al., 2018). The RHD shoulders bind DNA, whereas the interaction of RUNX1 protein and nucleic acid requires the β subunit of the core binding transcription factor, which is encoded by CBFβ (Riddell et al., 2020). The RUNX1 protein partners with the β subunit to form a transcriptionally active heterodimer that can activate or repress target gene expression (Mevel et al., 2019).
In Fig. 1, we present the top 15 biological pathways regulated by RUNX1, based on the biological processes and molecular pathways involved in the downstream targets of RUNX1. The hTFtarget online database has curated comprehensive TF-targets regulation from large-scale ChIP-Seq data of human TFs. We obtained a large scale of 22,724 target genes regulated by RUNX1 for enrichment analysis at the biological process and signaling pathway levels. Pathway enrichment analysis of the target genes of RUNX1 has demonstrated that RUNX1 plays a constructive role in the cell cycle, autophagy, ubiquitin-mediated proteolysis, cellular senescence, lysosomes, and apoptosis (Fig. 1A). Gene Ontology (GO) enrichment analysis of the downstream target genes of RUNX1 showed that RUNX1 is an important part of biological processes, such as the proteasomal protein catabolic and RNA catabolic processes, regulation of mitotic cell cycle phase transition, and protein targeting (Yu et al., 2012) (Fig. 1B).
Figure 1: Top 15 items of KEGG and GO enrichment analyses for RUNX1 target genes. (A) Summary of pathway enrichment in KEGG. (B) Summary of GO enrichment at the level of biological process.
The functions of RUNX1 have been elucidated in knockout mice. Germline knockout of RUNX1 causes embryonic lethality and a lack of hematopoietic stem cells (Okuda et al., 1996; Wang et al., 1996; Theriault et al., 2004). These animal models initially revealed that RUNX1 is essential for definitive hematopoiesis. Furthermore, studies using stage- and tissue-specific conditional knockouts have uncovered a myriad of additional roles for RUNX1 in tissues. Conditional knockout of RUNX1 in the hematopoietic system can cause reduced hematopoietic stem and progenitor cell growth (Cai et al., 2015), hematopoietic stem cell expansion and subsequent exhaustion (Jacob et al., 2010), impaired megakaryocyte maturation, and platelet production (Ichikawa et al., 2004; Growney et al., 2005). RUNX1 knockout in the immune system can result in T-cell differentiation blockage and defective B lymphocyte development (Egawa et al., 2007; Seo et al., 2012). The depletion of RUNX1 in the nervous system results in abnormal pain perception (Chen et al., 2006). Additionally, RUNX1 deletion in the cardiac system prevents adverse cardiac remodeling following myocardial infarction and maintains contractile function (McCarroll et al., 2018). A mammary gland without RUNX1 reduces the number of mature luminal cells (van Bragt et al., 2014). Muscles lacking RUNX1 can lead to atrophy of denervated myofibers, disorganized myofibrils, and excessive autophagy (Wang et al., 2005; Umansky et al., 2015). However, the effects of RUNX1 knockout on the respiratory system are nearly absent, and therefore, the role of RUNX1 in the lung is largely unknown.
The Emerging Role of RUNX1 in Pulmonary Development and Diseases
Levanon et al. (2001) described RUNX1 expression in both the epithelium and mesenchyme of mouse lungs at E14.4–E16.5 (Levanon et al., 2001). An earlier study suggested that RUNX1 deletion from embryonic day (E) 6.5 in alveolar epithelial cells is not congenitally fatal and that the morphological features of fetal and adult lungs are similar to wild-type mouse lungs of the same age (Tang et al., 2017). In addition, Haley et al. (2011) reported that RUNX1 was highly expressed in human developing lungs, based on the assessment of RUNX1 expression in human developing lungs obtained from discarded surgical material. Our previous work showed that the RUNX1 protein was highly expressed (4.6-fold change) in adult lungs compared to E18.5 (Tang et al., 2017). Although the reason for the differential expression of RUNX1 in developing and postnatal lungs has not been explored, it may be related to the involvement of RUNX1 in response to stress from the external environment.
RUNX1 distribution in normal adult lung
Single-cell RNA sequencing technology exquisitely draws the transcriptome profile of the lungs. The human protein atlas is an open online database for mapping human proteins using the integration of various omics technologies, including antibody-based imaging, mass spectrometry-based proteomics, transcriptomics, and systems biology. Information from the entry of data source GSE130148 in the human protein atlas revealed that mRNA expression of RUNX1 was most enriched in blood and immune cells, followed by epithelial and mesenchymal cells, while endothelial cells had the lowest expression (Fig. 2) (The Human Protein Atlas, 2022; Karlsson et al., 2021). The fact that RUNX1 is widely expressed in diverse cells in the lung hints that it might play unknown roles in lung injury and repair.
Figure 2: Expression of RUNX1 in the lung. Sourced from the human protein atlas (https://www.proteinatlas.org/ENSG00000159216-RUNX1/single+cell+type).
RUNX1 plays a key role in the progression of pulmonary fibrosis. By comparing different stages of IPF lung tissue, an algorithmic model prediction revealed that RUNX1 serves as an essential factor affecting the development of fibrosis (McDonough et al., 2019). To date, RUNX1 mRNA expression in pulmonary fibrosis has been found to be upregulated or downregulated after pulmonary fibrosis. The alteration of RUNX1 protein, which primarily stems from the mouse model, is upregulated in bleomycin-induced pulmonary fibrosis.
For instance, RNA sequencing of human pulmonary fibrosis tissue showed that mRNA expression of RUNX1 was downregulated in IPF (Log2 fold change −2.99) (Konigsberg et al., 2021). With the analysis of GEO2R, a similar trend of RUNX1 mRNA expression was observed in other datasets, GSE110147 and GSE68239. Of the differentially expressed genes (DEGs) of GSE110147, we observed that RUNX1 mRNA expression was mildly downregulated in the IPF lungs compared with normal lungs (Cecchini et al., 2018). Paradoxically, other studies have demonstrated that the mRNA expression of RUNX1 is increased in pulmonary fibrotic lungs. RUNX1 was upregulated in a cohort of patients with transplant-stage IPF compared to normal lungs (Log2 fold change 0.661) (Sivakumar et al., 2019). Other studies on sequencing data generated from patients with IFP supported the increased expression of RUNX1 in fibrotic lungs hypothesis (DePianto et al., 2015; Horimasu et al., 2017). Thus, we can see that the alteration of RUNX1 mRNA expression in IPF and other pulmonary fibrosis types is debatable. Biological differences and dissimilar cell proportions in tissues may result in the contradictory expression of RUNX1 mRNA in human fibrotic lung; however, a large sample size and more advanced technologies are needed to explore the precise alteration of RUNX1 expression in pulmonary fibrosis.
Single-cell RNA sequencing technology is becoming a powerful tool to assist in exploring abnormal signaling at the cellular level. Alveolar epithelial cells and fibroblasts are crucial components of the hypothesized mechanism of fibrogenesis. Based on the finite research on human pulmonary fibrosis, RUNX1 mRNA seems to have a more conspicuous change in fibroblasts/myofibroblast clusters than in alveolar epithelial cells. A study collected lung tissues from patients with IPF, systemic scleroderma-related pulmonary fibrosis, and healthy individuals; the results showed an increased expression of RUNX1 mRNA mainly in mesenchymal clusters, not in epithelial clusters in fibrotic lung (Tsukui et al., 2020). The distinctly increased expression of RUNX1 in mesenchymal clusters characterized by fibroblasts/myofibroblasts is consistent with Adams’s work (Adams et al., 2020). Additionally, an obvious increase in RUNX1 mRNA in fibroblasts/myofibroblasts of the human fibrotic lung was observed; however, there was a clear decrease in RUNX1 expression in type 2 alveolar epithelial cells (Morse et al., 2019). Furthermore, a notable elevation in RUNX1 mRNA expression in fibroblasts/myofibroblast clusters was demonstrated (Habermann et al., 2020). Notably, in this study, RUNX1 mRNA expression in type 2 alveolar epithelial cells did not change significantly. Changes in RUNX1 expression in different cell types in lung fibrosis varied. The question of which cell type is the leading cluster regulated by RUNX1 in pulmonary fibrosis needs to be answered in the future to steer the precise intervention of RUNX1 in specific cell types.
RUNX1 protein expression has been evaluated in animal models of pulmonary fibrosis (especially bleomycin-induced pulmonary fibrosis). Few studies have reported RUNX1 protein level changes in human fibrotic lung tissue. Increased levels of RUNX1 proteins, including 55 kDa isoform 1 and 37 kDa isoform 2, were found in mouse lungs after bleomycin administration (Dubey et al., 2022; O’Hare et al., 2021; Lin et al., 2020; Ji et al., 2021). However, the molecular mechanism by which the RUNX1 protein exerts its function in pulmonary fibrosis could not be determined and remains unknown. Further research is needed to determine whether RUNX1 directly regulates extracellular matrix molecule-related gene expression or influences the activity of signaling pathways involved in pulmonary fibrosis; nevertheless, it is reasonable to speculate that RUNX1 plays a certain role in pulmonary fibrosis.
RUNX1 in other respiratory diseases
RUNX1 has been shown to play a role in respiratory diseases such as lung cancer, asthma, pulmonary inflammation, and pulmonary arterial hypertension. Our previous study showed that RUNX1 regulates LPS-induced acute lung injury via NF-κB signaling (Tang et al., 2017). RUNX1 was found to play a crucial role in the development and progression of lung neoplasm (Ramsey et al., 2018; He et al., 2019). The loss of RUNX1 has been reported to be associated with aggressive lung adenocarcinomas. Miyamoto et al. (2019) found that the absence of RUNX1 impaired the ability of group 2 innate lymphoid cells (ILC2s) to proliferate and produce effector TH2 cytokines and chemokines, and that RUNX1 contributes to airway allergic pathogenesis. In 2021, a study described a novel role for PRMT1 as a coactivator of RUNX1, which might be relevant to epithelial dysfunction in asthma (Zhai et al., 2021). The RUNX1 inhibitor Ro24-7429 exhibited anti-inflammatory and anti-fibrotic effects in a bleomycin-induced pulmonary fibrosis mouse model (O’Hare et al., 2021). A previous study suggested that the administration of RUNX1 inhibitor in vivo reduced the severity of pulmonary arterial damage and that targeting RUNX1 could be regarded as a novel therapy for pulmonary arterial hypertension (Jeong et al., 2022). Despite these advances, the role of RUNX1 in lung development and pulmonary disease is not sufficiently understood.
Signaling Crosstalk between RUNX1 and Pathways Involved in Pulmonary Fibrosis and Remodeling
Although the direct effects of RUNX1 in the lungs have not been elucidated, RUNX1 has been shown to interact with some signaling pathways in other tissues. These signaling pathways are also involved in pulmonary remodeling.
RUNX1 interacts with the TGF-β signaling pathway. RUNX1 is activated by TGF-β, and several biological effects of TGF-β stimulation have been shown to involve RUNX1, including myofibroblast differentiation and proliferation (Kim et al., 2014; Dubey et al., 2022; Lin et al., 2020; Ji et al., 2021). For example, inhibition of RUNX1 in fibroblasts decreased the expression of Ki67 and α-SMA, which are proliferation and fibroblast trans-differentiation markers, respectively. RUNX1 overexpression activates the TGF-β signaling pathway and promotes the expression of p15, resulting in cell cycle arrest (Sun et al., 2022). The interactive effect between RUNX1 and TGF-β may create a vicious circle and facilitate the differentiation of fibroblasts and collagen deposition during the development of lung fibrosis.
Cell senescence is an emerging pivotal driving mechanism for pulmonary fibrosis (Yao et al., 2021; Schafer et al., 2017; Waters et al., 2018). RUNX1 has also been associated with senescence (Wolyniec et al., 2009). Senescence-associated β-galactosidase (SA-β-gal) staining of RUNX1 overexpression in human primary foreskin fibroblasts revealed that RUNX1 facilitated G1 arrest and cellular senescence. In addition, Anderson et al. (2018) found that RUNX1 mediates growth arrest and senescence of primary cells. The accumulation of senescent cells is associated with age-related diseases, such as pulmonary fibrosis (Yao et al., 2021; Waters et al., 2018; Lin et al., 2020; Parimon et al., 2021; Lin and Xu, 2020). Senescent cells exhibit a senescence-associated secretory phenotype (SASP), which is characterized by an aberrant secretory spectrum of cytokines. Schafer et al. (2017) demonstrated an elevated abundance of senescence biomarkers in IPF lungs, where p16 expression increased with disease severity. Moreover, their work showed that the secretome of senescent fibroblasts was fibrogenic. The upregulation of RUNX1 in fibroblasts to mediate fibrogenesis via cell senescence may be a reasonable conjecture of pulmonary fibrosis pathophysiology.
RUNX1 has also been linked to Akt signaling, and the PI3k-Akt signaling pathway is also involved in fibrosis (Qin et al., 2021; Wang et al., 2022; Zhang et al., 2021b; Kiszałkiewicz et al., 2017). The PI3K-Akt pathway is an intracellular signal transduction pathway that promotes protein synthesis, glucose metabolism, cell survival, cell cycle progression, and angiogenesis in response to extracellular signals. Epithelial-mesenchymal transition (EMT) induced by TGF-β in renal epithelial cells requires RUNX1 and p110δ-mediated Akt activation (Zhou et al., 2018). A similar connection between RUNX1 and Akt signaling was established in renal fibroblasts, indicating that RUNX1 may play an important role in fibrotic lesions by regulating the PI3k-Akt signaling pathway (Cheng et al., 2020). This evidence for the connection between RUNX1 and the PI3k-Akt signaling pathway, suggests that RUNX1 potentially plays a role in pulmonary fibrosis with the assistance of the PI3k-Akt signaling pathway.
In addition, arising shreds of evidence have shown that sustained reactivation of the Wnt/β-catenin pathway is related to the pathogenesis of fibrotic disorders (Chanda et al., 2019; Guo et al., 2012; Hu et al., 2020; Tao et al., 2016; Distler et al., 2019). Previous studies have illustrated the correlation between RUNX1 and Wnt/β-catenin signaling pathways. Hair follicle stem cells originate from RUNX1-expressing embryonic cells, and RUNX1 causes activation and proliferation of mouse hair follicle stem cells to ensure adult skin integrity by regulating the Wnt signaling pathway (Osorio et al., 2011). The microarray database revealed that RUNX1 expression was positively related to multiple molecules participating in the Wnt/β-catenin signaling pathway, such as LEF1, CD44, WNT5A, and CTNNB1 (Li et al., 2019). These data indicate that RUNX1 mediates the activity of the Wnt/β-catenin signaling pathway and serves as a potential mechanism of fibrogenesis and progression.
RUNX1 is involved in the complex network of non-coding RNA (microRNAs [miRNAs] and long non-coding RNAs [lncRNAs]) and tissue fibrosis (Cheng et al., 2020; Lin et al., 2020; Zhang et al., 2021a). Noncoding RNAs are the focus and frontiers of fibrotic lesions. miRNAs are small endogenous RNAs that post-transcriptionally mediate gene expression by binding to the 3′UTR of target mRNAs. lncRNAs are defined as transcripts >200 nucleotides in length that lack protein-coding potential and play a vital role at transcriptional and translational levels. Specifically, miRNA-194 targets RUNX1 to promote renal fibrosis via the PI3K-Akt signaling pathway (Cheng et al., 2020). In addition, miRNA-30b ameliorates diabetic nephropathy by suppressing RUNX1 and negatively regulating the PI3K pathway (Zhang et al., 2021a). Furthermore, lncRNA Hoxaas3, which responds to the TGF-β/Smad pathway, promotes pulmonary fibrogenesis via the miR-450b-5p-Runx1 axis (Lin et al., 2020).
Further studies are warranted to determine whether RUNX1 is linked to pulmonary fibrosis by regulating these signaling pathways (Fig. 3).
Figure 3: A schematic representation of the possible pathways mediated by RUNX1 that are involved in the fibrotic lung.
RUNX1 as a Novel Target in Pulmonary Fibrosis
Pulmonary fibrosis is an irreversible pathological process that poses a huge global health and economic burden. There is a persistent unmet need for more therapeutic methods, as patients with pulmonary fibrosis have poor long-term outcomes and a low quality of life. Considering the close interactions between the TF RUNX1 and pathological processes involved in pulmonary fibrosis, novel therapies aimed at targeting RUNX1 may achieve a more efficacious therapeutic response by impacting multiple downstream signaling pathways to slow down fibrotic progression or reverse early-stage fibrosis and prevent the destruction of the alveolar structure.
Generally, there are two effective strategies for suppressing the expression of RUNX1. One is a small molecule inhibitor that interrupts the combination of RUNX1 and CBFB to form functional dimers, and the other is to silence RUNX1 RNA to stop DNA transcription. Two types of RUNX1 inhibitors that interfere with the binding of RUNX1 and the target sequence (Ro5-3335 and Ro24-7429, respectively), were used in vivo and in vitro, consequently blocking possible cellular signal transduction (Cunningham et al., 2012). Ro5-3335 served an anti-fibrotic role, reducing N-cadherin expression induced by TGF-β stimulation in the A549 cell lineage (O’Hare et al., 2021). Furthermore, Ro24-7429, a small molecule inhibitor, was initially invented for the human immunodeficiency virus type 1 (HIV-1) protein and was proven to be safe in a phase 2 trial of AIDS (Haubrich et al., 1995). Ro24-7429 robustly ameliorated lung fibrosis and inflammation in the bleomycin-induced acute fibrosis stage (O’Hare et al., 2021). With the intervention of RNA silencing of RUNX1, TGF-β failed to activate major components, such as fibronectin and alpha-SMA, in human lung fibroblasts (Dubey et al., 2022). A well-designed carrier with a surface carrying an antibody fragment to combine with mesenchymal cells in the lungs was administered in a bleomycin-induced pulmonary fibrosis model. The carrier managed to silence RUNX1 expression in the lung, leading to the reduction in collagen deposition and triumphing over the effects of pulmonary fibrosis (Ji et al., 2021). Accordingly, pharmacological targeting of RUNX1 could be a potential strategy to tackle uncontrolled fibrotic tissue remodeling.
Accumulating evidence has indicated that RUNX1 plays a role in pulmonary fibrosis. RUNX1 modification in the fibrotic lungs at the mRNA and protein levels is in its infancy, while inhibition or deletion of RUNX1 in vivo has an anti-fibrotic effect in mice. The therapeutic effect of the inhibition or deletion of RUNX1 in an animal model has limited value. For pulmonary fibrosis, implementing targeted therapy through the intervention of RUNX1 in primate or clinical trials would be more persuasive in the future. Considering the powerful function of RUNX1 in multiple tissues and organs, systemic administration may result in fatal or unexpected adverse effects, and the safety and efficacy of influencing RUNX1 expression should be considered. New signs of progress have helped us determine which delivery method is more effective. Administration of RUNX1 small interfering RNA by inhalation presented a promising effect of reducing collagen deposition in fibrotic mouse lungs, which indicated that inhaled administration directly delivered to the lung could be a better option than oral administration. Research on RUNX1 should extend to the possible molecular mechanisms and signaling pathways of RUNX1 in fibrosis. Research on the role of RUNX1 in pulmonary fibrosis is an urgent requirement with translational potential.
Funding Statement: This research was funded by 1.3.5 Project for Disciplines of Excellence, West China Hospital, Sichuan University, Grant No. ZYJC18021; Post-Doctoral Research Project, West China Hospital, Grant No. 2021HXBH074; the National Natural Science Foundation of China, Grant No. 82100075; Sichuan Science and Technology Program, Grant Nos. 2020YFH0073 and 2021YFG0329.
Author Contributions: Study conception and design: J.L; analysis and interpretation of results: J.L and B.Y; manuscript revise: F.P and F.L. All authors reviewed the results and approved the final version of the manuscript.
Ethics Approval: Not applicable.
Conflicts of Interest: The authors declare that they have no conflicts of interest to report regarding the present study.
References
Abbasi S, Sinha S, Labit E, Rosin NL, Yoon G et al. (2020). Distinct regulatory programs control the latent regenerative potential of dermal fibroblasts during wound healing. Cell Stem Cell 27: 396–412.E6. https://doi.org/10.1016/j.stem.2020.07.008 [Google Scholar] [PubMed] [CrossRef]
Adams TS, Schupp JC, Poli S, Ayaub EA, Neumark N et al. (2020). Single-cell RNA-seq reveals ectopic and aberrant lung-resident cell populations in idiopathic pulmonary fibrosis. Science Advances 6: 1437. https://doi.org/10.1126/sciadv.aba1983 [Google Scholar] [PubMed] [CrossRef]
Anderson G, Mackay N, Gilroy K, Hay J, Borland G et al. (2018). RUNX-mediated growth arrest and senescence are attenuated by diverse mechanisms in cells expressing RUNX1 fusion oncoproteins. Journal of Cellular Biochemistry 119: 2750–2762. https://doi.org/10.1002/jcb.26443 [Google Scholar] [PubMed] [CrossRef]
Ban C, Yan W, Xie B, Zhu M, Liu Y et al. (2018). Spectrum of interstitial lung disease in China from 2000 to 2012. The European Respiratory Journal 52: 1701554. https://doi.org/10.1183/13993003.01554-2017 [Google Scholar] [PubMed] [CrossRef]
Brack AS, Conboy MJ, Roy S, Lee M, Kuo CJ, Keller C, Rando TA (2007). Increased Wnt signaling during aging alters muscle stem cell fate and increases fibrosis. Science 317: 807–810. https://doi.org/10.1126/science.1144090 [Google Scholar] [PubMed] [CrossRef]
Bradner JE, Hnisz D, Young RA (2017). Transcriptional addiction in cancer. Cell 168: 629–643. https://doi.org/10.1016/j.cell.2016.12.013 [Google Scholar] [PubMed] [CrossRef]
Cai X, Gao L, Teng L, Ge J, Oo ZM, Kumar AR, Gilliland DG, Mason PJ, Tan K, Speck NA (2015). Runx1 deficiency decreases ribosome biogenesis and confers stress resistance to hematopoietic stem and progenitor cells. Cell Stem Cell 17: 165–177. https://doi.org/10.1016/j.stem.2015.06.002 [Google Scholar] [PubMed] [CrossRef]
Cecchini MJ, Hosein K, Howlett CJ, Joseph M, Mura M (2018). Comprehensive gene expression profiling identifies distinct and overlapping transcriptional profiles in non-specific interstitial pneumonia and idiopathic pulmonary fibrosis. Respiratory Research 19: 153. https://doi.org/10.1186/s12931-018-0857-1 [Google Scholar] [PubMed] [CrossRef]
Chanda D, Otoupalova E, Smith SR, Volckaert T, de Langhe SP, Thannickal VJ (2019). Developmental pathways in the pathogenesis of lung fibrosis. Molecular Aspects of Medicine 65: 6556–6569. https://doi.org/10.1016/j.mam.2018.08.004 [Google Scholar] [PubMed] [CrossRef]
Chen CL, Broom DC, Liu Y, de Nooij JC, Li Z, Cen C, Samad OA, Jessell TM, Woolf CJ, Ma Q (2006). Runx1 determines nociceptive sensory neuron phenotype and is required for thermal and neuropathic pain. Neuron 49: 365–377. https://doi.org/10.1016/j.neuron.2005.10.036 [Google Scholar] [PubMed] [CrossRef]
Cheng L, Tu C, Min Y, He D, Wan S, Xiong F (2020). MiR-194 targets Runx1/Akt pathway to reduce renal fibrosis in mice with unilateral ureteral obstruction. International Urology and Nephrology 52: 1801–1808. https://doi.org/10.1007/s11255-020-02544-5 [Google Scholar] [PubMed] [CrossRef]
Cunningham L, Finckbeiner S, Hyde RK, Southall N, Marugan J et al. (2012). Identification of benzodiazepine Ro5-3335 as an inhibitor of CBF leukemia through quantitative high throughput screen against RUNX1-CBFβ interaction. Proceedings of the National Academy of Sciences of the United States of America 109: 14592–14597. https://doi.org/10.1073/pnas.1200037109 [Google Scholar] [PubMed] [CrossRef]
DePianto DJ, Chandriani S, Abbas AR, Jia G, N’Diaye EN et al. (2015). Heterogeneous gene expression signatures correspond to distinct lung pathologies and biomarkers of disease severity in idiopathic pulmonary fibrosis. Thorax 70: 48–56. https://doi.org/10.1136/thoraxjnl-2013-204596 [Google Scholar] [PubMed] [CrossRef]
Distler JHW, Györfi AH, Ramanujam M, Whitfield ML, Königshoff M, Lafyatis R (2019). Shared and distinct mechanisms of fibrosis. Nature Reviews Rheumatology 15: 705–730. https://doi.org/10.1038/s41584-019-0322-7 [Google Scholar] [PubMed] [CrossRef]
Dubey S, Dubey PK, Umeshappa CS, Ghebre YT, Krishnamurthy P (2022). Inhibition of RUNX1 blocks the differentiation of lung fibroblasts to myofibroblasts. Journal of Cellular Physiology 237: 2169–2182. https://doi.org/10.1002/jcp.30684 [Google Scholar] [PubMed] [CrossRef]
Edeling M, Ragi G, Huang S, Pavenstädt H, Susztak K (2016). Developmental signalling pathways in renal fibrosis: The roles of Notch, Wnt and Hedgehog. Nature Reviews Nephrology 12: 426–439. https://doi.org/10.1038/nrneph.2016.54 [Google Scholar] [PubMed] [CrossRef]
Egawa T, Tillman RE, Naoe Y, Taniuchi I, Littman DR (2007). The role of the Runx transcription factors in thymocyte differentiation and in homeostasis of naive T cells. Journal of Experimental Medicine 204: 1945–1957. https://doi.org/10.1084/jem.20070133 [Google Scholar] [PubMed] [CrossRef]
Flaherty KR, Fell CD, Huggins JT, Nunes H, Sussman R et al. (2018). Safety of nintedanib added to pirfenidone treatment for idiopathic pulmonary fibrosis. European Respiratory Journal 52: 1800230. https://doi.org/10.1183/13993003.00230-2018 [Google Scholar] [PubMed] [CrossRef]
Gay D, Ghinatti G, Guerrero-Juarez CF, Ferrer RA, Ferri F et al. (2020). Phagocytosis of Wnt inhibitor SFRP4 by late wound macrophages drives chronic Wnt activity for fibrotic skin healing. Science Advances 6: 575. https://doi.org/10.1126/sciadv.aay3704 [Google Scholar] [PubMed] [CrossRef]
Growney JD, Shigematsu H, Li Z, Lee BH, Adelsperger J et al. (2005). Loss of Runx1 perturbs adult hematopoiesis and is associated with a myeloproliferative phenotype. Blood 106: 494–504. https://doi.org/10.1182/blood-2004-08-3280 [Google Scholar] [PubMed] [CrossRef]
Guo Y, Xiao L, Sun L, Liu F (2012). Wnt/β-catenin signaling: A promising new target for fibrosis diseases. Physiological Research 61: 337–346. https://doi.org/10.33549/physiolres.932289 [Google Scholar] [PubMed] [CrossRef]
Habermann AC, Gutierrez AJ, Bui LT, Yahn SL, Winters NI et al. (2020). Single-cell RNA sequencing reveals profibrotic roles of distinct epithelial and mesenchymal lineages in pulmonary fibrosis. Science Advances 6: 1691. https://doi.org/10.1126/sciadv.aba1972 [Google Scholar] [PubMed] [CrossRef]
Haley KJ, Lasky-Su J, Manoli SE, Smith LA, Shahsafaei A, Weiss ST, Tantisira K (2011). RUNX transcription factors: Association with pediatric asthma and modulated by maternal smoking. American Journal of Physiology-Lung Cellular and Molecular Physiology 301: L693–L701. https://doi.org/10.1152/ajplung.00348.2010 [Google Scholar] [PubMed] [CrossRef]
Haubrich RH, Flexner C, Lederman MM, Hirsch M, Pettinelli CP et al. (1995). A randomized trial of the activity and safety of Ro 24-7429 (Tat antagonist) versus nucleoside for human immunodeficiency virus infection. The AIDS Clinical Trials Group 213 Team. Journal of Infectious Diseases 172: 1246–1252. https://doi.org/10.1093/infdis/172.5.1246 [Google Scholar] [PubMed] [CrossRef]
He C, Bai X, Li Y, Sun H, Kong X, Fu B, Chen L, Zhu K, Li P, Xu S (2019). Runt-related transcription factor 1 contributes to lung cancer development by binding to tartrate-resistant acid phosphatase 5. Cell Cycle 18: 3404–3419. https://doi.org/10.1080/15384101.2019.1678966 [Google Scholar] [PubMed] [CrossRef]
Horimasu Y, Ishikawa N, Taniwaki M, Yamaguchi K, Hamai K et al. (2017). Gene expression profiling of idiopathic interstitial pneumonias (IIPsIdentification of potential diagnostic markers and therapeutic targets. BMC Medical Genetics 18: 88. https://doi.org/10.1186/s12881-017-0449-9 [Google Scholar] [PubMed] [CrossRef]
Hsu J, Huang HT, Lee CT, Choudhuri A, Wilson NK et al. (2020). CHD7 and Runx1 interaction provides a braking mechanism for hematopoietic differentiation. Proceedings of the National Academy of Sciences of the United States of America 117: 23626–23635. https://doi.org/10.1073/pnas.2003228117 [Google Scholar] [PubMed] [CrossRef]
Hsu HS, Liu CC, Lin JH, Hsu TW, Hsu JW, Su K, Hung SC (2017). Involvement of ER stress, PI3K/AKT activation, and lung fibroblast proliferation in bleomycin-induced pulmonary fibrosis. Scientific Reports 7: 1949. https://doi.org/10.1038/s41598-017-14612-5 [Google Scholar] [PubMed] [CrossRef]
Hu HH, Cao G, Wu XQ, Vaziri ND, Zhao YY (2020). Wnt signaling pathway in aging-related tissue fibrosis and therapies. Ageing Research Reviews 60: 101063. https://doi.org/10.1016/j.arr.2020.101063 [Google Scholar] [PubMed] [CrossRef]
Ichikawa M, Asai T, Saito T, Seo S, Yamazaki I et al. (2004). AML-1 is required for megakaryocytic maturation and lymphocytic differentiation, but not for maintenance of hematopoietic stem cells in adult hematopoiesis. Nature Medicine 10: 299–304. https://doi.org/10.1038/nm997 [Google Scholar] [PubMed] [CrossRef]
Ikawa Y, Ng PS, Endo K, Kondo M, Chujo S et al. (2008). Neutralizing monoclonal antibody to human connective tissue growth factor ameliorates transforming growth factor-β-induced mouse fibrosis. Journal of Cellular Physiology 216: 680–687. https://doi.org/10.1002/jcp.21449 [Google Scholar] [PubMed] [CrossRef]
Jacob B, Osato M, Yamashita N, Wang CQ, Taniuchi I, Littman DR, Asou N, Ito Y (2010). Stem cell exhaustion due to Runx1 deficiency is prevented by Evi5 activation in leukemogenesis. Blood 115: 1610–1620. https://doi.org/10.1182/blood-2009-07-232249 [Google Scholar] [PubMed] [CrossRef]
Jeong EM, Pereira M, So EY, Wu KQ, Del Tatto M et al. (2022). Targeting RUNX1 as a novel treatment modality for pulmonary arterial hypertension. Cardiovascular Research 351: cvac001. https://doi.org/10.1093/cvr/cvac001 [Google Scholar] [PubMed] [CrossRef]
Ji Q, Hou J, Yong X, Gong G, Muddassir M, Tang T, Xie J, Fan W, Chen X (2021). Targeted dual small interfering ribonucleic acid delivery via non-viral polymeric vectors for pulmonary fibrosis therapy. Advanced Materials 33: 2007798. https://doi.org/10.1002/adma.202007798 [Google Scholar] [PubMed] [CrossRef]
Karimi-Shah BA, Chowdhury BA (2015). Forced vital capacity in idiopathic pulmonary fibrosis—FDA review of pirfenidone and nintedanib. New England Journal of Medicine 372: 1189–1191. https://doi.org/10.1056/NEJMp1500526 [Google Scholar] [PubMed] [CrossRef]
Karlsson M, Zhang C, Méar L, Zhong W, Digre A et al. (2021). A single-cell type transcriptomics map of human tissues. Science Advances 7: 3620. https://doi.org/10.1126/sciadv.abh2169 [Google Scholar] [PubMed] [CrossRef]
Kim W, Barron DA, San Martin R, Chan KS, Tran LL, et al. (2014). RUNX1 is essential for mesenchymal stem cell proliferation and myofibroblast differentiation. Proceedings of the National Academy of Sciences of the United States of America 111: 16389–16394. https://doi.org/10.1073/pnas.1407097111 [Google Scholar] [PubMed] [CrossRef]
King TEJr, Bradford WZ, Castro-Bernardini S, Fagan EA, Glaspole I et al. (2014). A phase 3 trial of pirfenidone in patients with idiopathic pulmonary fibrosis. New England Journal of Medicine 370: 2083–2092. https://doi.org/10.1056/NEJMoa1402582 [Google Scholar] [PubMed] [CrossRef]
Kiszałkiewicz J, Piotrowski W, Brzeziańska-Lasota E (2017). Signaling pathways and their miRNA regulators involved in the etiopathology of idiopathic pulmonary fibrosis (IPF) and hypersensitivity pneumonitis (HP). Advances in Respiratory Medicine 85: 169–178. https://doi.org/10.5603/ARM.2017.0029 [Google Scholar] [PubMed] [CrossRef]
Konigsberg IR, Borie R, Walts AD, Cardwell J, Rojas M, Metzger F, Hauck SM, Fingerlin TE, Yang IV, Schwartz DA (2021). Molecular signatures of idiopathic pulmonary fibrosis. American Journal of Respiratory Cell and Molecular Biology 65: 430–441. https://doi.org/10.1165/rcmb.2020-0546OC [Google Scholar] [PubMed] [CrossRef]
Kunz CR, Jadus MR, Kukes GD, Kramer F, Nguyen VN, Sasse SA (2004). Intrapleural injection of transforming growth factor-β antibody inhibits pleural fibrosis in empyema. Chest 126: 1636–1644. https://doi.org/10.1378/chest.126.5.1636 [Google Scholar] [PubMed] [CrossRef]
Kuppe C, Ramirez Flores RO, Li Z, Hannani M, Tanevski J et al. (2020). Spatial multi-omic map of human myocardial infarction. bioRxiv 15: 83. https://doi.org/10.1101/2020.12.08.411686 [Google Scholar] [CrossRef]
Lederer DJ, Martinez FJ (2018). Idiopathic pulmonary fibrosis. New England Journal of Medicine 378: 1811–1823. https://doi.org/10.1056/NEJMra1705751 [Google Scholar] [PubMed] [CrossRef]
Lee DW, Lee WJ, Cho J, Yun CO, Roh H, Chang HP, Roh TS, Lee JH, Lew DH (2020). Inhibition of Wnt signaling pathway suppresses radiation-induced dermal fibrosis. Scientific Reports 10: 13594. https://doi.org/10.1038/s41598-020-70243-3 [Google Scholar] [PubMed] [CrossRef]
Levanon D, Brenner O, Negreanu V, Bettoun D, Woolf E et al. (2001). Spatial and temporal expression pattern of Runx3 (Aml2) and Runx1 (Aml1) indicates non-redundant functions during mouse embryogenesis. Mechanisms of Development 109: 413–417. https://doi.org/10.1016/S0925-4773(01)00537-8 [Google Scholar] [PubMed] [CrossRef]
Li M, Huang H, Li L, He C, Zhu L et al. (2021a). Core transcription regulatory circuitry orchestrates corneal epithelial homeostasis. Nature Communications 12: 420. https://doi.org/10.1038/s41467-020-20713-z [Google Scholar] [PubMed] [CrossRef]
Li Q, Lai Q, He C, Fang Y, Yan Q et al. (2019). RUNX1 promotes tumour metastasis by activating the Wnt/β-catenin signalling pathway and EMT in colorectal cancer. Journal of Experimental & Clinical Cancer Research 38: 7. https://doi.org/10.1186/s13046-019-1330-9 [Google Scholar] [PubMed] [CrossRef]
Li C, Sun J, Liu Q, Dodlapati S, Ming H et al. (2021b). The landscape of accessible chromatin in quiescent cardiac fibroblasts and cardiac fibroblasts activated after myocardial infarction. Epigenetics 17: 1–20. https://doi.org/10.1080/15592294.2021.1982158 [Google Scholar] [PubMed] [CrossRef]
Lin Y, Xu Z (2020). Fibroblast senescence in idiopathic pulmonary fibrosis. Frontiers in Cell and Developmental Biology 8: 593283. https://doi.org/10.3389/fcell.2020.593283 [Google Scholar] [PubMed] [CrossRef]
Lin S, Zhang R, Xu L, Ma R, Xu L, Zhu L, Hu J, An X (2020). LncRNA Hoxaas3 promotes lung fibroblast activation and fibrosis by targeting miR-450b-5p to regulate Runx1. Cell Death & Disease 11: 706. https://doi.org/10.1038/s41419-020-02889-w [Google Scholar] [PubMed] [CrossRef]
Loft A, Alfaro AJ, Schmidt SF, Pedersen FB, Terkelsen MK et al. (2021). Liver-fibrosis-activated transcriptional networks govern hepatocyte reprogramming and intra-hepatic communication. Cell Metabolism 33: 1685–1700.e1689. https://doi.org/10.1016/j.cmet.2021.06.005 [Google Scholar] [PubMed] [CrossRef]
McCarroll CS, He W, Foote K, Bradley A, McGlynn K et al. (2018). Runx1 deficiency protects against adverse cardiac remodeling after myocardial infarction. Circulation 137: 57–70. https://doi.org/10.1161/CIRCULATIONAHA.117.028911 [Google Scholar] [PubMed] [CrossRef]
McDonough JE, Ahangari F, Li Q, Jain S, Verleden SE et al. (2019). Transcriptional regulatory model of fibrosis progression in the human lung. JCI Insight 4: 67. https://doi.org/10.1172/jci.insight.131597 [Google Scholar] [PubMed] [CrossRef]
Mevel R, Draper JE, Lie ALM, Kouskoff V, Lacaud G (2019). RUNX transcription factors: Orchestrators of development. Development 146: 71 dev148296. https://doi.org/10.1242/dev.148296 [Google Scholar] [PubMed] [CrossRef]
Miyamoto C, Kojo S, Yamashita M, Moro K, Lacaud G, Shiroguchi K, Taniuchi I, Ebihara T (2019). Runx/Cbfβ complexes protect group 2 innate lymphoid cells from exhausted-like hyporesponsiveness during allergic airway inflammation. Nature Communications 10: 447. https://doi.org/10.1038/s41467-019-08365-0 [Google Scholar] [PubMed] [CrossRef]
Moor CC, Mostard RLM, Grutters JC, Bresser P, Aerts JGJV, Dirksen CD, Kimman ML, Wijsenbeek MS (2020). Patient expectations, experiences and satisfaction with nintedanib and pirfenidone in idiopathic pulmonary fibrosis: A quantitative study. Respiratory Research 21: e44. https://doi.org/10.1186/s12931-020-01458-1 [Google Scholar] [PubMed] [CrossRef]
Morse C, Tabib T, Sembrat J, Buschur KL, Bittar HT et al. (2019). Proliferating SPP1/MERTK-expressing macrophages in idiopathic pulmonary fibrosis. European Respiratory Journal 54: 1802441. https://doi.org/10.1183/13993003.02441-2018 [Google Scholar] [PubMed] [CrossRef]
Noble PW, Albera C, Bradford WZ, Costabel U, Glassberg MK et al. (2011). Pirfenidone in patients with idiopathic pulmonary fibrosis (CAPACITYTwo randomised trials. Lancet 377: 1760–1769. https://doi.org/10.1016/S0140-6736(11)60405-4 [Google Scholar] [PubMed] [CrossRef]
Okuda T, van Deursen J, Hiebert SW, Grosveld G, Downing JR (1996). AML1, the target of multiple chromosomal translocations in human leukemia, is essential for normal fetal liver hematopoiesis. Cell 84: 321–330. https://doi.org/10.1016/S0092-8674(00)80986-1 [Google Scholar] [PubMed] [CrossRef]
Osorio KM, Lilja KC, Tumbar T (2011). Runx1 modulates adult hair follicle stem cell emergence and maintenance from distinct embryonic skin compartments. Journal of Cell Biology 193: 235–250. https://doi.org/10.1083/jcb.201006068 [Google Scholar] [PubMed] [CrossRef]
O’Hare M, Amarnani D, Whitmore HAB, An M, Marino C et al. (2021). Targeting runt-related transcription factor 1 prevents pulmonary fibrosis and reduces expression of severe acute respiratory syndrome coronavirus 2 host mediators. American Journal of Pathology 191: 1193–1208. https://doi.org/10.1016/j.ajpath.2021.04.006 [Google Scholar] [PubMed] [CrossRef]
Pan Z, Yang K, Wang H, Xiao Y, Zhang M, Yu X, Xu T, Bai T, Zhu H (2020). MFAP4 deficiency alleviates renal fibrosis through inhibition of NF-κB and TGF-β/Smad signaling pathways. The FASEB Journal 34: 14250–14263. https://doi.org/10.1096/fj.202001026R [Google Scholar] [PubMed] [CrossRef]
Parimon T, Hohmann MS, Yao C (2021). Cellular senescence: pathogenic mechanisms in lung fibrosis. International Journal of Molecular Sciences 22: 6214. https://doi.org/10.3390/ijms22126214 [Google Scholar] [PubMed] [CrossRef]
Peng L, Wen L, Shi QF, Gao F, Huang B, Meng J, Hu CP, Wang CM (2020). Scutellarin ameliorates pulmonary fibrosis through inhibiting NF-κB/NLRP3-mediated epithelial-mesenchymal transition and inflammation. Cell Death & Disease 11: 346. https://doi.org/10.1038/s41419-020-03178-2 [Google Scholar] [PubMed] [CrossRef]
Qin W, Cao L, Massey IY (2021). Role of PI3K/Akt signaling pathway in cardiac fibrosis. Molecular and Cellular Biochemistry 476: 4045–4059. https://doi.org/10.1007/s11010-021-04219-w [Google Scholar] [PubMed] [CrossRef]
Ramsey J, Butnor K, Peng Z, Leclair T, van der Velden J, Stein G, Lian J, Kinsey CM (2018). Loss of RUNX1 is associated with aggressive lung adenocarcinomas. Journal of Cellular Physiology 233: 3487–3497. https://doi.org/10.1002/jcp.26201 [Google Scholar] [PubMed] [CrossRef]
Richeldi L, Costabel U, Selman M, Kim DS, Hansell DM et al. (2011). Efficacy of a tyrosine kinase inhibitor in idiopathic pulmonary fibrosis. New England Journal of Medicine 365: 1079–1087. https://doi.org/10.1056/NEJMoa1103690 [Google Scholar] [PubMed] [CrossRef]
Richeldi L, du Bois RM, Raghu G, Azuma A, Brown KK et al. (2014). Efficacy and safety of nintedanib in idiopathic pulmonary fibrosis. New England Journal of Medicine 370: 2071–2082. https://doi.org/10.1056/NEJMoa1402584 [Google Scholar] [PubMed] [CrossRef]
Riddell A, McBride M, Braun T, Nicklin SA, Cameron E, Loughrey CM, Martin TP (2020). RUNX1: An emerging therapeutic target for cardiovascular disease. Cardiovascular Research 116: 1410–1423. https://doi.org/10.1093/cvr/cvaa034 [Google Scholar] [PubMed] [CrossRef]
Schafer MJ, White TA, Iijima K, Haak AJ, Ligresti G et al. (2017). Cellular senescence mediates fibrotic pulmonary disease. Nature Communications 8: 14532. https://doi.org/10.1038/ncomms14532 [Google Scholar] [PubMed] [CrossRef]
Seo W, Ikawa T, Kawamoto H, Taniuchi I (2012). Runx1-Cbfβ facilitates early B lymphocyte development by regulating expression of Ebf1. Journal of Experimental Medicine 209: 1255–1262. https://doi.org/10.1084/jem.20112745 [Google Scholar] [PubMed] [CrossRef]
Sivakumar P, Thompson JR, Ammar R, Porteous M, McCoubrey C et al. (2019). RNA sequencing of transplant-stage idiopathic pulmonary fibrosis lung reveals unique pathway regulation. ERJ Open Research 5: 00117–2019. https://doi.org/10.1183/23120541.00117-2019 [Google Scholar] [PubMed] [CrossRef]
Sun W, Yi D, Zhu L, Zeng J, Liu Y et al. (2022). RUNX1 overexpression triggers TGF-β signaling to upregulate p15 and thereby blocks early hematopoiesis by inducing cell cycle arrest. Stem Cell Research 60: 102694. https://doi.org/10.1016/j.scr.2022.102694 [Google Scholar] [PubMed] [CrossRef]
Tang X, Sun L, Jin X, Chen Y, Zhu H et al. (2017). Runt-related transcription factor 1 regulates LPS-induced acute lung injury via NF-κB signaling. American Journal of Respiratory Cell and Molecular Biology 57: 174–183. https://doi.org/10.1165/rcmb.2016-0319OC [Google Scholar] [PubMed] [CrossRef]
Tang X, Sun L, Wang G, Chen B, Luo F (2018). RUNX1: A regulator of NF-kB signaling in pulmonary diseases. Current Protein & Peptide Science 19: 172–178. https://doi.org/10.2174/1389203718666171009111835 [Google Scholar] [PubMed] [CrossRef]
Tang CY, Wu M, Zhao D, Edwards D, McVicar A et al. (2021). Runx1 is a central regulator of osteogenesis for bone homeostasis by orchestrating BMP and WNT signaling pathways. PLoS Genetics 17: e1009233. https://doi.org/10.1371/journal.pgen.1009233 [Google Scholar] [PubMed] [CrossRef]
Tao H, Yang JJ, Shi KH, Li J (2016). Wnt signaling pathway in cardiac fibrosis: New insights and directions. Metabolism: Clinical and Experimental 65: 30–40. https://doi.org/10.1016/j.metabol.2015.10.013 [Google Scholar] [PubMed] [CrossRef]
The Human Protein Atlas (2022). Expression of Runx1 in lung. https://www.proteinatlas.org/ENSG00000159216-RUNX1/single+cell+type. [Google Scholar]
Theriault FM, Roy P, Stifani S (2004). AML1/Runx1 is important for the development of hindbrain cholinergic branchiovisceral motor neurons and selected cranial sensory neurons. Proceedings of the National Academy of Sciences of the United States of America 101: 10343–10348. https://doi.org/10.1073/pnas.0400768101 [Google Scholar] [PubMed] [CrossRef]
Tsukui T, Sun KH, Wetter JB, Wilson-Kanamori JR, Hazelwood LA et al. (2020). Collagen-producing lung cell atlas identifies multiple subsets with distinct localization and relevance to fibrosis. Nature Communications 11: 1920. https://doi.org/10.1038/s41467-020-15647-5 [Google Scholar] [PubMed] [CrossRef]
Umansky KB, Gruenbaum-Cohen Y, Tsoory M, Feldmesser E, Goldenberg D, Brenner al, Groner Y, Cox GA (2015). Runx1 transcription factor is required for myoblasts proliferation during muscle regeneration. PLoS Genetics 11: e1005457. https://doi.org/10.1371/journal.pgen.1005457 [Google Scholar] [PubMed] [CrossRef]
van Bragt MP, Hu X, Xie Y, Li Z (2014). RUNX1, a transcription factor mutated in breast cancer, controls the fate of ER-positive mammary luminal cells. Elife 3: e03881. https://doi.org/10.7554/eLife.03881.022 [Google Scholar] [CrossRef]
Vancheri C, Kreuter M, Richeldi L, Ryerson CJ, Valeyre D et al. (2018). Nintedanib with add-on pirfenidone in idiopathic pulmonary fibrosis. Results of the INJOURNEY trial. American Journal of Respiratory and Critical Care Medicine 197: 356–363. https://doi.org/10.1164/rccm.201706-1301OC [Google Scholar] [PubMed] [CrossRef]
Wang X, Blagden C, Fan J, Nowak SJ, Taniuchi I, et al. (2005). Runx1 prevents wasting, myofibrillar disorganization, and autophagy of skeletal muscle. Genes & Development 19: 1715–1722. https://doi.org/10.1101/gad.1318305 [Google Scholar] [PubMed] [CrossRef]
Wang J, Hu K, Cai X, Yang B, He Q, Wang J, Weng Q (2022). Targeting PI3K/AKT signaling for treatment of idiopathic pulmonary fibrosis. Acta Pharmaceutica Sinica B 12: 18–32. https://doi.org/10.1016/j.apsb.2021.07.023 [Google Scholar] [PubMed] [CrossRef]
Wang Z, Li X, Chen H, Han L, Ji X et al. (2021). Resveratrol alleviates bleomycin-induced pulmonary fibrosis via suppressing HIF-1α and NF-κB expression. Aging 13: 4605–4616. https://doi.org/10.18632/aging.202420 [Google Scholar] [PubMed] [CrossRef]
Wang Q, Stacy T, Binder M, Marin-Padilla M, Sharpe AH, Speck NA (1996). Disruption of the Cbfa2 gene causes necrosis and hemorrhaging in the central nervous system and blocks definitive hematopoiesis. Proceedings of the National Academy of Sciences of the United States of America 93: 3444–3449. https://doi.org/10.1073/pnas.93.8.3444 [Google Scholar] [PubMed] [CrossRef]
Waters DW, Blokland KEC, Pathinayake PS, Burgess JK, Mutsaers SE, Prele CM, Schuliga M, Grainge CL, Knight DA (2018). Fibroblast senescence in the pathology of idiopathic pulmonary fibrosis. American Journal of Physiology-Lung Cellular and Molecular Physiology 315: L162–L172. https://doi.org/10.1152/ajplung.00037.2018 [Google Scholar] [PubMed] [CrossRef]
Wolyniec K, Wotton S, Kilbey A, Jenkins A, Terry A, Peters G, Stocking C, Cameron E, Neil JC (2009). RUNX1 and its fusion oncoprotein derivative, RUNX1-ETO, induce senescence-like growth arrest independently of replicative stress. Oncogene 28: 2502–2512. https://doi.org/10.1038/onc.2009.101 [Google Scholar] [PubMed] [CrossRef]
Wu L, Zhang Q, Mo W, Feng J, Li S et al. (2017). Quercetin prevents hepatic fibrosis by inhibiting hepatic stellate cell activation and reducing autophagy via the TGF-β1/Smads and PI3K/Akt pathways. Scientific Reports 7: 9289. https://doi.org/10.1038/s41598-017-09673-5 [Google Scholar] [PubMed] [CrossRef]
Wynn TA, Ramalingam TR (2012). Mechanisms of fibrosis: Therapeutic translation for fibrotic disease. Nature Medicine 18: 1028–1040. https://doi.org/10.1038/nm.2807 [Google Scholar] [PubMed] [CrossRef]
Yao C, Guan X, Carraro G, Parimon T, Liu X et al. (2021). Senescence of alveolar type 2 cells drives progressive pulmonary fibrosis. American Journal of Respiratory and Critical Care Medicine 203: 707–717. https://doi.org/10.1164/rccm.202004-1274OC [Google Scholar] [PubMed] [CrossRef]
Yu G, Wang LG, Han Y, He QY (2012). clusterProfiler: An R package for comparing biological themes among gene clusters. Omics: A Journal of Integrative Biology 16: 284–287. https://doi.org/10.1089/omi.2011.0118 [Google Scholar] [PubMed] [CrossRef]
Yusen RD, Edwards LB, Kucheryavaya AY, Benden C, Dipchand AI et al. (2014). The registry of the international society for heart and lung transplantation: Thirty-first adult lung and heart-lung transplant report—2014; Focus theme: Retransplantation. Journal of Heart and Lung Transplantation 33: 1009–1024. https://doi.org/10.1016/j.healun.2014.08.004 [Google Scholar] [PubMed] [CrossRef]
Zhai W, Sun H, Li Z, Li L, Jin A et al. (2021). PRMT1 modulates processing of asthma-related primary microRNAs (Pri-miRNAs) into mature miRNAs in lung epithelial cells. Journal of Immunology 206: 11–22. https://doi.org/10.4049/jimmunol.2000887 [Google Scholar] [PubMed] [CrossRef]
Zhang Y, Cai Y, Zhang H, Zhang J, Zeng Y et al. (2021a). Brown adipose tissue transplantation ameliorates diabetic nephropathy through the miR-30b pathway by targeting Runx1. Metabolism-Clinical and Experimental 12: 154916. https://doi.org/10.1016/j.metabol.2021.154916 [Google Scholar] [PubMed] [CrossRef]
Zhang Y, Jin D, Kang X, Zhou R, Sun Y, Lian F, Tong X (2021b). Signaling pathways involved in diabetic renal fibrosis. Frontiers in Cell and Developmental Biology 9: 696542. https://doi.org/10.3389/fcell.2021.696542 [Google Scholar] [PubMed] [CrossRef]
Zhou T, Luo M, Cai W, Zhou S, Feng D, Xu C, Wang H (2018). Runt-related transcription factor 1 (RUNX1) promotes TGF-β-induced renal tubular epithelial-to-mesenchymal transition (EMT) and renal fibrosis through the PI3K subunit p110δ. eBioMedicine 31: 217–225. https://doi.org/10.1016/j.ebiom.2018.04.023 [Google Scholar] [PubMed] [CrossRef]
Cite This Article
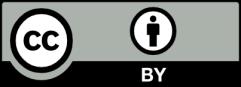