Open Access
ARTICLE
Effects of docosahexaenoic acid or arachidonic acid supplementation on the behavior of cardiomyocytes derived from human pluripotent stem cells
1 Graduate School of Science and Engineering, Yamagata University, Yonezawa, 9928510, Japan
2 Faculty of Health Sciences, Japan Healthcare University, Sapporo, 0620053, Japan
* Corresponding Author: ZHONGGANG FENG. Email: -u.ac.jp
BIOCELL 2023, 47(5), 1095-1106. https://doi.org/10.32604/biocell.2023.028186
Received 03 December 2022; Accepted 09 January 2023; Issue published 10 April 2023
Abstract
Background: Human heart changes its energetic substrates from lactate and glucose to fatty acids during the neonatal period. Noticing the lack of fatty acids in media for the culture of cardiomyocytes derived from human pluripotent stem cells (hiPS-CM), researchers have supplemented mixtures of fatty acids to hiPS-CM and reported the enhancement in the maturation of hiPS-CM. In our previous studies, we separately supplemented two polyunsaturated fatty acids (PUFAs), docosahexaenoic acid (DHA) or arachidonic acid (AA), to rat fetal cardiomyocytes and found that the supplementations upregulated the expressions of mRNAs for cardiomyocyte differentiation, fatty acid metabolism, and cellular adhesion. The enhancement in cellular contractility was attributed to the improvement in intercellular connection rather than a direct enhancement of the contractile force. Methods: This study reports the successive results of the effects of DHA or AA supplementation on hiPS-CM. In addition to the contractile force and mRNA measurements used in the previous study, we further investigated the effect of different cellular aggregations on the contractile force output by means of finite element analysis, measured glucose and fatty acids metabolites, and assessed cTNT and MLC2v expressions through immunofluorecsence evaluation. Results: It showed that the sole supplementation of albumin-conjugated DHA or AA can be taken up by hiPS-CM without other uptake-enhancing factors, and the supplementations may activate the CD36_ERRγ metabolic pathway. DHA or AA supplementation increased the cellular contractile ratio on collagen gels and AA supplementation stimulated hiPS-CM aggregation to form cellular clusters. The enhancement effect on the hiPS-CM contractile force was modest since the increase in contractile force was not significant. AA supplementation was more effective than DHA supplementation because it significantly upregulated mRNA expressions of P300 and CD36. However, finite element analysis showed that the formation of clusters on a collagen gel attenuated the contractile force exerted by the gel on its surroundings. Conclusion: DHA and AA, as having been supplemented in infant formulas, have no direct and significant enhancement effect on the performance of the hiPS-CM when they were supplemented individually, although they were able to enter the cellular metabolic system. The AA supplementation showed some auxiliary effect on the maturation of hiPS-CM, which is worthy of further investigation under the consideration of membrane composition alteration and remodeling of membrane molecules.Keywords
Heart failure is the leading cause of death worldwide; therefore, developing effective therapies to treat heart failure has always been an urgent demand in cardiovascular medicine. Regenerative medicine holds great promise as a potentially better alternative therapy to drug treatment and donor/artificial heart transplantation. For cardiac regenerative modality, cardiomyocytes derived from stem cells may be the only cell source because adult cardiomyocytes lack proliferative ability. Herein, human induced pluripotent stem (hiPS) cells possess the obvious advantages of unlimited renewal capability and avoiding immune responses in recipients, attracting enormous interest in cardiac regenerative medicine (Takahashi et al., 2007). Although highly effective cardiomyocyte differentiation from hiPS cells by controlling the Wnt pathway has been realized (Lian et al., 2013) and the first clinical trial in the world using hiPS-derived cardiomyocytes (hiPS-CM) was conducted in Japan (Mallapaty, 2022; Miyagawa et al., 2022), differentiating maturely functional cardiomyocytes from hiPS cells is still a big unmet challenge (Karbassi et al., 2020).
Till now, a number of strategies to differentiate mature hiPS-CM have been reported (Tu et al., 2018), including physical stimulations (Ronaldson-Bouchard et al., 2018), gene editing (Kuppusamy et al., 2015), biochemical factors (Yang et al., 2014), functional culture substrates and three-dimensional culture (Ribeiro et al., 2015; Fong et al., 2016), and co-culturing with other cell types (Pasquier et al., 2017). Although a putative optimal methodology for the maturation may be a sophisticated integration of multiple effective agents rather than any single one, independent investigation for each effective agent is a prerequisite to realizing such integration. Currently, the metabolic feature of cardiomyocytes and the role of fatty acids in the maturation of hiPS-CM are attracting research attention (Slaats et al., 2020; Tani et al., 2022), and this has been encouraged by great success in developing a method to purify hiPS-CM from a differentiated cell pool by exploiting metabolic features of cardiomyocytes (Tohyama et al., 2013).
Human hearts shift their energetic substrates from lactate and glucose to fatty acids during the neonatal period (Isu et al., 2019; Horikoshi et al., 2019). However, the fatty acids supplied to cultured cells as the composition of fetal bovine serum (FBS) supplemented with culture media are quite limited. Noticing this deviation, Yang et al. (2019) treated hiPS-CM with media containing both glucose and a mixture of fatty acids designed to match content levels found in newborns and reported the induced cardiomyocyte hypertrophy and a significant increase in cardiomyocyte contractile force. Most recently, Yoshida et al. (2022) compared the types of fatty acids metabolized between cardiomyocytes in culture and heart cells in vivo, supplemented a mixture of fatty acids to the hiPS-CM culture medium, and found that the supplementation increased oxygen consumption and contractile force of the hiPS-CM. In another report, Lopez et al. (2021) showed that a more mature metabolic phenotype of hiPS-CM can be induced within a 3D culture by stimulating fatty acid oxidation.
In this regard, we found considerable shortages of ω–3 and ω–6 polyunsaturated fatty acids (PUFAs) in cultured rat fetal cardiomyocytes by comparing the fatty acid composition in the cultured cells and in the cells in neonate myocardial tissue (Karimata et al., 2013). In particular, the contents of docosahexaenoic acid (DHA) and arachidonic acid (AA) were significantly lower than those in the neonatal tissue. Then, we supplemented DHA and AA, respectively, to Dulbecco’s modified Eagle’s medium/F12 culture medium and evaluated the effects of the supplementations on the behavior of rat fetal cardiomyocytes (Yano et al., 2021). The supplementations were found to upregulate the expressions of mRNAs for cardiomyocyte differentiation, fatty acid metabolism, and cellular adhesion. The enhancement of cellular contractility was attributed to the improvement of intercellular connection rather than a direct enhancement of the contractile force.
This study is a succession to the work by Yano et al. (2021), and here we report the newly obtained results of the effects of DHA or AA supplementation on the behavior of hiPS-CM.
hiPS cell culture and cardiomyocyte differentiation
The hiPS cell line (RBRC-HPS0002) was purchased from RIKEN Cell Bank. The hiPS cells were maintained and expanded by feeder-independent culture with mTeSR1 medium (Stemcell Technologies, BC, Canada) in 6-well plates (AS ONE, Osaka, Japan) coated with Matrigel (Corning, NY, USA). After expansion, the cells were transferred into tissue culture flasks (Iwaki, Shizuoka, Japan) coated with Matrigel, and the feeder-independent culture was continued up to 95%–98% cell confluency. Then, the cardiomyocyte differentiation was induced by CHIR-IWP2 treatment to control the Wnt signaling pathway, and the initiation time of the treatment was denoted as day 0. The differentiation protocol is described in detail earlier (Lian et al., 2013). The resultant hiPS-CM were cultured with serum-free Roswell Park Memorial Institute/B-27 medium (Thermo Fisher Scientific, MA, USA) plus 1% 4-(2-hydroxyethyl)-1-piperazineethanesulfo-nic acid (HEPES) and the medium was changed every other day. On day 15, the spontaneous beating of the hiPS-CM was already obvious under an inverted microscope (Olympus, Tokyo, Japan). Then, the hiPS-CM were harvested and a portion (ca. a half of the cell number) was seeded onto circular collagen gels to measure the contractile force (see next subsection), while the remaining hiPS-CM were purified by lactate selection (Tohyama et al., 2013) for 5 days. DHA or AA was bound to bovine serum albumin (Sigma-Aldrich, MO, USA) in a mole ratio of 2:1 and supplemented in the culture medium at a concentration of 20 µM for DHA or 50 µM for AA to the culture on collagen gels and the culture after the hiPS-CM purification. The culturing of hiPS-CM lasted for two weeks with the supplementations. Culture without supplementations was used as the study control. Culture for purified hiPS-CM was used to assess mRNA expression and metabolite measurements. HiPS-CM cultured on collagen gels were used for immunofluorescence observation and the contractile force measurement.
Measurement of the contractile force of hiPS-CM
The details of the measurement have been described earlier (Yano et al., 2021). Briefly, hiPS-CM were seeded on circular collagen gels at the beginning of the culture with DHA or AA supplementation. A lab-made vision-based measurement system was employed to measure the cardiomyocyte contractile force at the end of the culture (Fig. 1). In the system, the circular gel (denoted as hiPS-CM-gel) was regulated into an equilateral triangle by using two stainless hooks to fix two vertices and an L-shape cantilever made of a thin silver wire to pull the rest vertex, where the beating of the vertex was measured under an inverted microscope (Olympus, Tokyo, Japan) at different stretch strains of the hiPS-CM-gel. The cantilever was pulled to obtain stretch strains of 5%, 10%, 15%, and 20% to the initial side length. The force exerted onto the cantilever was obtained by the measured maximum beating displacement of the beating vertex multiplied by the cantilever deflection coefficient. The measured results for each experiment are presented as a relative contractile force to reduce the variation from the experiments at different times. Here, the relative contractile force was defined as the ratio of contractile force with PUFA supplementation to the contractile force without supplementation (i.e., the control sample in each experiment) at zero stretch strain.
Figure 1: A schematic drawing of the lab-made vision-based system for the measurement of cardiomyocyte contractile force (Yano et al., 2021), in which a circular hiPS-CM-gel is regulated into an equilateral triangle with its two vertices fixed on hooks and one vertex attached to a L-shape cantilever. The contractile force exerted from the hiPS-CM on the gel can be measured in terms of the cantilever deflection.
Measurement of hiPS-CM behavior on top of the collagen gels and finite element analysis (FEA) of the hiPS-CM-gels
Because the supplementation of AA significantly stimulated cellular aggregation on the hiPS-CM-gels (Fig. 2 and corresponding results), we modeled the hiPS-CM-gel as two cases: (a) cells uniformly distributed as a sheet on the top of the gels and (b) cell clusters formed on the top. We modeled the clusters as circles and their radii were calculated as the square root of the area of each cluster (measured through ImageJ) divided by the square root of π.
Figure 2: Observation of hiPS-CM beating on collagen gels under a microscope. (a) Uniform distribution of hiPS-CM of control; marks (1 and 2) were used to measure the contractile ratio as explained in the text. (b) Clusters (one of them is indicated by the white circle) manifested on the gel with arachidonic acid supplementation. Scale bar = 200 μm.
We further investigated the contractile ratios of the uniformly distributed hiPS-CM and the aggregated cell clusters by analyzing the beating movies through ImageJ. The contractile ratio was defined as the difference in length of a straight line (for uniform cell distribution) or of the radius (for cell cluster) between the relaxation and the maximal contraction divided by the length at relaxation. As shown in Fig. 2a, for the uniformly distributed hiPS-CM, two dark points appearing under the microscope were chosen as marks. With the gel beating, the differences in length for calculating contractile ratios in the longitudinal and the width directions were measured by tracing the displacements of the marks on the movie images through ImageJ. For the cellular clusters (Fig. 2b), the difference of a cluster area between relaxation and maximal contraction was measured by tracing the movie images and the contractile ratio of the cluster in its radius could be calculated. All the clusters in a movie scene were picked up and the average of the contractile ratio was calculated over these clusters.
We used the Computer Aided Engineering software Autodesk® Fusion360 to conduct FEA to the deformation and stress in the hiPS-CM-gel caused by hiPS-CM beating. As shown in Fig. 3, uniformly distributed cells or an aggregated cluster were modeled as a sheet or a disk, respectively. The FEA domain included one cluster for the hiPS-CM-gel with AA supplementation, while the FEA domain for the control and DHA supplementation (the cell sheet cases) was set to have the same size as the FEA domain for AA supplementation. Herein, although DHA supplementation also tended to stimulate cellular aggregation resulting in some dark regions with high cell density where the contractile ratio was measured (Table 2), the cell sheet model still fits for this case because a great many compacted clusters were not observed with DHA supplementation. The size of the FEA domain with AA supplementation was determined based on experimental measurements and explained as follows. First, the cluster numbers were counted along the gel circumference length (l) and width (w), denoted as N_cl and N_w, respectively. Second, average effective length (CL) and width (W), to which one cluster applied contractile force, were calculated by CL = l/N_cl and W = w/N_w, respectively; the CL and W were set to be the length and the width of the FEA domain. The thickness of the domain was identical to the gel thickness (H). FEA was then conducted for this domain (length × width × thickness = CL × W × H, see Fig. 3b) in terms of ~1.0 × 105 tetrahedron elements. As for the boundary conditions of the analysis, the boundary condition of the top surface for the case with the cellular sheet model (Fig. 3a) was set to contract at a contractile ratio; for the cluster case (Fig. 3b), the cluster disk contracting at a contractile ratio while the rest of the top surface was set to be the free surface condition. Boundary conditions on the lateral and bottom surfaces of the domain were set as free surfaces. Two ends at the length of the domain were fixed (no displacement). Thus, the tensile force in the cross-section of the ends was regarded as the contractile force of the domain due to the contraction of the hiPS-CM sheet or the cluster on the top. The total contractile force exerted by a hiPS-CM-gel to its surroundings was calculated as the tensile force in the domain times the number of the domains included in the whole gel, i.e., the number was equal to the gel volume divided by the domain volume.
Figure 3: FEA analysis of the contraction of hiPS-CM-gels with (a) uniformly distributed hiPS-CM and (b) hiPS-CM clusters on the top of collagen gels. As a contractile ratio is imposed on the cellular model, the deformation of the gel and tensile force transmitted at the two ends of the gel can be analyzed.
Measurement of gene expression
Table 1 presents information on genes investigated in this study. The expression of mRNAs of these genes was investigated by means of real-time polymerase chain reaction (RT-PCR). The genes were categorized into three groups related to “differentiation and maturity” (NKX2.5, SRF, P300, cTNT, ACTN2, MLC2v, MLC2a, HCN4, and HRT2), “cell adhesion” (CDH2 and CX43), and “fatty acid metabolism” (CD36, ERRγ, PPARα, PPARδ, PGC1α, and COX4). GAPDH was used for internal reference. mRNA was extracted through the conventional phenol-based method, followed by a cDNA synthesis via PrimeScript RT reagent Kit (Takara Bio, Shiga, Japan). The RT-PCR was conducted using a Thermal Cycler (Takara Bio, Shiga, Japan). The condition was set as: initial denaturation at 95°C for 30 s, 45 repetitive cycles of dissociation (95°C, 5 s), and annealing (60°C, 30 s). The ΔΔCt method was used to evaluate the relative expression of the genes.
Measurement of glucose and fatty acids metabolism
Glucose and fatty acids metabolism, respectively, were measured using a glucose-6-phosphate (G6P) assay kit (Sigma-Aldrich, MO, USA) and a β-hydroxybutyrate (BHB) assay kit (Sigma-Aldrich, MO, USA). The measurement was performed on days 4 and 15 with the supplementation following the protocols provided by the manufacturer.
Observation of immunofluorescence and evaluation of the expression by corrected total cell fluorescence (CTCF)
Cells were fixed with 4% paraformaldehyde and permeabilized with 2% Triton X-100, respectively, for 15 min at room temperature. Nonspecific binding was masked by incubation in 3% (V/V) goat albumin in phosphoric buffer saline for 1 h at 37°C. First antibodies rabbit IgG anti-myosin light chain 2 polyclonal (1:200, Proteintech, IL, USA) and mouse IgG1 anti-cardiac troponin T (1:200, Thermo Fisher Scientific, MA, USA) with respective secondary antibodies Alexa488 goat anti-rabbit IgG (1:1000, Thermo Fisher Scientific, MA, USA) and Alexa546 goat anti-mouse IgG1 (1:1000, Thermo Fisher Scientific, MA, USA) were used. After the immune staining, cells were incubated with 300 nM DAPI solution (Thermo Fisher Scientific, MA, USA) for 5 min.
To quantitatively evaluate the expressions of cTNT and MLC2v on the fluorescent images, we analyzed the images by ImageJ through the CTCF method (Jakic et al., 2017; McCloy et al., 2014) as follows: an area including ca. 30 cells was selected once a time through judging the DAPI staining; then, the area, integrated density, and mean gray value were measured; finally, the CTCF was calculated as CTCF = integrated density – (area of selected cells × mean fluorescence of background readings)/cell number, which was regarded as the fluorescence intensity of each cell.
Multiple comparison tests were performed using one-way ANOVA non-parameter analysis followed by the Dunnet test for multiple comparisons (BellCurve for Excel 3.20) to compare the data in the DHA and AA groups with respect to the control. p < 0.05 was regarded as significant.
As shown in Fig. 2, hiPS-CM cultured with AA supplementation formed cellular clusters on the gels. DHA supplementation also stimulated cell aggregation to render higher cell density to places scattered on the gels compared to their neighbors; however, these places were not as big and obvious as those dark clusters with AA supplementation (Fig. 2b). The samples with different media did not have significantly different beat rates, though the beating rate under DHA or AA supplementation showed a lower tendency compared with that of the control (Table 2). Conversely, for the supplementations, the contractile ratios tended to be greater; in particular, the contractile ratio of the clusters induced by AA supplementation was significantly greater than that of the control (Table 2).
Fig. 4 shows the relative contractile force obtained in this study; the contractile force with DHA or AA supplementation tended to increase, although it was not significant among the samples. The maximum contractile force of around 5% strain for AA supplementation or around 10% for DHA supplementation was more obvious than the maximum in the control data. The existence of maximum contractile force vs. construct strain at a few percent may be regarded as the Frank-Starling type behavior and an assessment for cardiomyocyte maturation. However, the maxima in Fig. 4 were not remarkable enough to make a sound assessment.
Figure 4: Relative contractile force measured in the experiments. The average contractile force of the controls at zero stretch strain of the gels was 44.9 ± 23.8 μN; n = 3, mean ± SE.
The FEA results are presented in Fig. 5. Under the same contractile ratio, the uniformly distributed beating cells induced greater stress in the gel beneath the cells than the cellular cluster does (compare Figs. 5a with 5b). The contractile force of a whole gel was converted (Fig. 5c), and the gel with cell clusters consequently generated a smaller contractile force than the gel with uniformly distributed cells. This can explain why the AA supplementation with a significantly larger contractile ratio (shown in Table 2) did not give rise to a larger contractile force compared with DHA supplementation (Fig. 4).
Figure 5: Contraction analysis of hiPS-CM-gels is conducted by finite element analysis. Stress in the gels caused by contractile hiPS-CM on the top surfaces under a 6% contractile ratio with (a) uniform cellular distribution or (b) cellular cluster is illustrated via a heat map. (c) Integrated contractile force in the whole gel vs. a different contractile ratio of the hiPS-CM on the top surface.
Fig. 6 shows the mRNA expression of 17 genes in hiPS-CM cultured with the supplementations. The expressions of most of the genes did not significantly alter with the supplementations. Expression of transcriptional factor P300 was significantly upregulated with AA supplementation, which may correlate with the upregulation tendency in cTNT and MLC2a expressions with the supplementation. In contrast, DHA did not result in an obvious increase in the expression of genes associated with differentiation and maturation. Notably, MLC2v was downregulated with the supplementations; in particular, significantly downregulated with DHA supplementation.
Figure 6: mRNA expression of the hiPS-CM assessed by real-time PCR. 17 genes are categorized into three classifications associated with cardiomyocyte differentiation and maturation, cell adhesion, and fatty acid metabolism. n = 5, mean ± SE; *p < 0.05 vs. control; †p < 0.05 between docosahexaenoic acid (DHA) 20 and arachidonic acid (AA) 50 (by Bonferroni method). All data are presented by the dots.
Expression of CX43 with AA supplementation was upregulated, consistent with the phenomenon of cellular clustering with AA supplementation. However, in contrast, the important cellular adhesion protein CDH2 was significantly downregulated.
Genes coding for two critical factors with respect to fatty acid metabolism, the lipid transporter CD36 and the metabolic transcriptional factor ERRγ were upregulated (Fig. 6). Consistent with these upregulations, Fig. 7 shows that the precursor of fatty acid metabolism, BHB, was increased in the cytoplasm; meanwhile, the first intermediate of glucose metabolism, G6P, decreased. These alterations in the metabolites persisted with culture duration and the decrease in G6P on day 15 became significant.
Figure 7: Measurement of the metabolites within cytoplasm under different supplementations. The increase in β-hydroxybutyrate, one of the ketone bodies, is considered a biosynthesized product on the supplemented docosahexaenoic acid (DHA) or arachidonic acid (AA) and used as a metabolic intermediate due to the corresponding decrease in the expression of G6P, the first intermediate of glucose metabolism. N = 2–3, mean ± SE; *p < 0.05 vs. control. All data are presented by the dots.
The immunofluorescence images and the quantitative CTCF analysis are presented in Figs. 8a and 8b, respectively. MLC2v and cTNT were substantially expressed in the differentiated cells and the CTCF data may explain the enhanced tendency due to the DHA or AA supplementation.
Figure 8: (a) Immunofluorescence image of the hiPS-CM on collagen gels. (b) Corrected total cell fluorescence (CTCF) assessment of the images to quantify the expression of targeted proteins. n = 17–21, mean ± SE. All data are presented by the dots.
However, the above-discussed data had certain inconsistencies. The expressions of cTNT and MLC2v assessed by RT-PCR (Fig. 6) disagreed with those by CTCF via immunostaining (Fig. 8), and the downregulated expression of CDH2 (Fig. 6) with AA supplementation did not coincide with the cell clustering (Fig. 2b). We speculate that these inconsistencies may be caused by the purification process of hiPS-CM samples for assessing mRNA expression as further discussed in the next section.
Fatty acids are essential components for our lives. Mammals can biosynthesize saturated and monounsaturated fatty acids in vivo (Iso et al., 2006; Matsuzaka et al., 2007), but cannot effectively synthesize PUFAs because they do not have the desaturation enzyme necessary for catalyzing a double bond at n–3 or n–6 positions. Therefore, PUFAs have to be supplied with food (Schmitz and Ecker, 2008). Considering the lack of DHA and AA during infant development, these two PUFAs have been supplemented in formulas. These supplementations exert positive effects on cognition, brain connectivity, and allergy in early childhood (Carlson and Colombo, 2016; Clandinin et al., 2005) as well as their cardioprotective effects (Shysh et al., 2016).
Research interests in the effects of fatty acids on the differentiation and growth of cardiomyocytes derived from stem cells are based on the fact that during the neonatal period, mammalian hearts shift their energetic substrates from lactate and glucose to fatty acids (Horikoshi et al., 2019; Isu et al., 2019), which is obviously disparate in the cell culture with glucose-based media. Taking the metabolic environment in vivo as a blueprint for differentiation and maturation of hiPS-CM (Slaats et al., 2020), Yang et al. (2019) treated hiPS-CM with media containing both glucose and a cocktail of fatty acids including palmitate, oleate, and linoleate and reported induced cardiomyocyte hypertrophy and a significant increase in cardiomyocyte contractile force. Yoshida et al. (2022) supplemented a mixture of fatty acids including oleic acid, stearic acid, myristic acid, linoleic acid, linolenic acid, palmitic acid, palmitoleic acid, and arachidonic acid to the hiPS-CM culture medium and found that the supplementation increased oxygen consumption and contractile force of the hiPS-CM. Ramachandra et al. (2018) reported that supplementing hiPS-CM with palmitate and oleate significantly enhanced mitochondrial remodeling, oxygen consumption rates, and ATP production.
Our previous study also paid attention to the metabolism feature of cardiomyocytes and found that the DHA and AA content in primary-cultured cardiomyocytes was significantly lower than in the neonatal tissue (Karimata et al., 2013) and that the supplementation of DHA or AA to the primary culture upregulated the expressions of mRNAs for cardiomyocyte differentiation (Nkx2.5), fatty acid metabolism (Cd36, peroxisome proliferator-activated receptors α (Pparα), and Pparδ), and cellular adhesion (Cdh2 and Cx43). The enhancement of cellular contractility was attributed to the improvement in intercellular connection rather than the cellular contractile force itself (Yano et al., 2021). Continuing the thread in this study, we further investigated the individual effects of these two PUFAs on the hiPS-CM culture rather than the integrated effects of a fatty acid mixture.
First, the uptake of DHA and AA into hiPS-CM was evidenced by the increase in CD36 expression (Fig. 6), the increase in BHB, and the decrease in G6P in the cytoplasm (Fig. 7). The alteration in the amount of the metabolites showed that substrates of the energy metabolism was replaced partially by the supplemented PUFAs. A putative activation of a metabolic pathway stimulated by AA supplementation in hiPS-CM, i.e., CD36_ERRγ_PGC1α_COX4 can be proposed (refer Fig. 6), which is different from our results of the rat cardiomyocyte primary culture where the activation of CD36_PPARα/PPARδ was suggested (Yano et al., 2021). ERRγ and PPARs are nuclear receptors actively involved with cardiomyocyte metabolism (Huss et al., 2015; Montaigne et al., 2021). Estrogen-related receptors (ERR) largely function as a transcriptional activator in the heart to drive oxidative metabolism, usually interacting with PGC1α, which co-expresses in the heart and increases myocardial mitochondrial capacity assisting the metabolism shift from reliance on glycolysis to fatty acid oxidation (Huss et al., 2015). The upregulation tendency of nuclear receptor ERRγ in hiPS-CM instead of PPARs in the primary culture of rat fetal cardiomyocytes may be because the hiPS-CM in this study were at their earlier development state than the fetal cardiomyocytes in the primary culture, rather than due to the difference in species because the metabolism pathways in mammalian cardiomyocytes are quite conserved and stable (Lopaschuk and Jaswal, 2010; Chung et al., 2007).
As for the contractile behavior, the measured contractile force did not increase significantly although the relative force to control showed an increasing tendency. Similar to the primary culture, cellular aggregation and the increase in contractile ratio were observed. We expected that upregulation in CX43 and CDH2 mRNA could be detected; however, the supplementations led to a significant decrease in CDH2. We speculate that this was a result of the purification process in the mRNA experiment because culture on the gels did not present such a decrease in CDH2 (data not shown here). Although the vital transcriptional factor P300 (Ghosh, 2020) was upregulated with the supplementations, the genes related to the cardiomyocyte constitution and maturation were not consistently upmodulated (Fig. 6). The expression of cTNT and MLC2a instead of MLC2v increased with the AA supplementation, whereas their expressions decreased with the DHA supplementation. Immunofluorescence investigation in terms of CTCF for each cell (Fig. 8b) did not show the decrease accordingly. These results may also implicate the influence of the purification process and suggest positive effects of co-culture with other cell types.
In summary, we investigated the effects of individual supplementation of DHA or AA rather than their mixture on the hiPS-CM behavior and observed that the sole supplementation of albumin-conjugated DHA or AA can be taken up by hiPS-CM without other uptake-enhancing factors. The enhancement effect on the hiPS-CM contractile force was modest since the increase in the contractile force was not significant. Supplementation of AA was more effective than that of DHA because it significantly upregulated mRNA expressions of P300, CD36, and COX4. However, the FEA showed that the formation of clusters on a collagen gel attenuated the contractile force exerted from the gel to its surroundings.
This study is a continuation of our previous study on the primary culture of rat neonatal cardiomyocytes and intended to assess the effect of the supplementation of DHA or AA on the hiPS-CM. This is quite interesting as cardiac differentiation/maturation from hiPS cells is an intensively attractive research topic in cardiac regeneration. The maturation of cardiomyocytes derived from stem cells is a complicated process that surely involves multiple biophysical and biochemical factors acting in a properly temporal manner and any single factor may not possibly dominate the process. We report here that these two PUFAs, having been supplemented in infant formulas, have no direct and significant enhancement effect on the performance of the hiPS-CM when they were supplemented individually, although they were able to enter the cellular metabolic system. The AA supplementation may have some auxiliary effect on the maturation of hiPS-CM, as observed by the cluster formation and mRNA expression. We speculate that these putatively positive effects may be attributed to the alteration of cardiomyocytes membrane composition and the remodeling of some membrane functional molecules, such as cardiolipin (Khairallah et al., 2012; Lamaziere et al., 2015; El-Hafidi et al., 2020) rather than the uptake of AA into the energy metabolism. Based on the results of this study, further work may be focused on 1) the mechanism of the cluster formation and its potential for the promotion of cardiomyocyte maturation, and 2) the combination with other biochemical factors, such as Tafazzin and acyl-transferases, in the consideration of the membrane/functional molecule remodeling due to the AA supplementation.
Funding Statement: The present study was supported financially in part by Grants-in-Aid for Scientific Research (C) (21K12661) from the Japan Society for the Promotion of Science and Grant 12-003-111 from Takahashi Industrial and Economic Research Foundation.
Author Contributions: Study conception and design: M. Yano, T. Nakamura, D. Sato, and Z. Feng. Data collection: M. Yano and K. Hiroi. Analysis and interpretation of results: M. Yano, K. Hiroi, T. Yuasa, K. Inoue, O. Yamamoto, D. Sato, and Z. Feng. Draft manuscript preparation: M. Yano, K. Hiroi, and Z. Feng. All authors reviewed the results and approved the final version of the manuscript.
Availability of Data and Materials: The dataset generated during and/or analyzed during the current study are available from the corresponding author on reasonable request.
Ethics Approval: Not applicable.
Conflicts of Interest: The authors declare that they have no conflicts of interest to report regarding the present study.
References
Anderson PAW, Malouf NN, Oakeley AE, Pagani ED, Allen PD (1991). Troponin T isoform expression in humans. A comparison among normal and failing adult heart, fetal heart, and adult and fetal skeletal muscle. Circulation Research 69: 1226–1233. https://doi.org/10.1161/01.RES.69.5.1226 [Google Scholar] [PubMed] [CrossRef]
Backs J, Olson EN (2006). Control of cardiac growth by histone acetylation/deacetylation. Circulation Research 98: 15–24. https://doi.org/10.1161/01.RES.0000197782.21444.8f [Google Scholar] [PubMed] [CrossRef]
Balza RO, Misra RP (2005). Role of the serum response factor in regulating contractile apparatus gene expression and sarcomeric integrity in cardiomyocytes. Journal of Biological Chemistry 281: 6498–6510. https://doi.org/10.1074/jbc.M509487200 [Google Scholar] [PubMed] [CrossRef]
Barber RD, Harmer DW, Coleman RA, Clark BJ (2005). GAPDH as a housekeeping gene: analysis of GAPDH mRNA expression in a panel of 72 human tissues. Physiological Genomics 21: 389–395. https://doi.org/10.1152/physiolgenomics.00025.2005 [Google Scholar] [PubMed] [CrossRef]
Biel M, Schneider A, Wahl C (2002). Cardiac HCN channels: Structure, function, and modulation. Trends in Cardiovascular Medicine 12: 206–213. https://doi.org/10.1016/S1050-1738(02)00162-7 [Google Scholar] [PubMed] [CrossRef]
Bruneau BG (2002). Transcriptional regulation of vertebrate cardiac morphogenesis. Circulation Research 90: 509–519. https://doi.org/10.1161/01.RES.0000013072.51957.B7 [Google Scholar] [PubMed] [CrossRef]
Carlson S, Colombo J (2016). Docosahexaenoic acid and arachidonic acid nutrition in early development. Advances in Pediatrics 63: 453–471. https://doi.org/10.1016/j.yapd.2016.04.011 [Google Scholar] [PubMed] [CrossRef]
Chen J, Kubalak SW, Minamisawa S, Price RL, Becker KD, Hickey R, Ross J, Chien KR (1998). Selective requirement of myosin light chain 2v in embryonic heart function. Journal of Biological Chemistry 273: 1252–1256. https://doi.org/10.1074/jbc.273.2.1252 [Google Scholar] [PubMed] [CrossRef]
Chung S, Dzeja PP, Faustino RS, Perez-Terzic C, Behfar A, Terzic A (2007). Mitochondrial oxidative metabolism is required for the cardiac differentiation of stem cells. Nature Clinical Practice Cardiovascular Medicine 4: S60–S67. https://doi.org/10.1038/ncpcardio0766 [Google Scholar] [PubMed] [CrossRef]
Clandinin M, Van Aerde J, Merkel K, Harris C, Springer M, Hansen J, Diersen-Schade D (2005). Growth and development of preterm infants fed infant formulas containing docosahexaenoic acid and arachidonic acid. Journal of Pediatrics 146: 461–468. https://doi.org/10.1016/j.jpeds.2004.11.030 [Google Scholar] [PubMed] [CrossRef]
Ding Y, Yang KD, Yang Q (2014). The role of PPARd signaling in the cardiovascular system. Progress in Molecular Biology and Translational Science 121: 451–473. https://doi.org/10.1016/B978-0-12-800101-1.00014-4 [Google Scholar] [PubMed] [CrossRef]
El-Hafidi M, Correa F, Zazueta C (2020). Mitochondrial dysfunction in metabolic and cardiovascular diseases associated with cardiolipin remodeling. BBA—Molecular Basis of Disease 1866: 165744. https://doi.org/10.1016/j.bbadis.2020.165744 [Google Scholar] [PubMed] [CrossRef]
Fong AH, Romero-López M, Heylman CM, Keating M, Tran D et al. (2016). Three-dimensional adult cardiac extracellular matrix promotes maturation of human induced pluripotent stem cell-derived cardiomyocytes. Tissue Engineering Part A 22: 1016–1025. https://doi.org/10.1089/ten.tea.2016.0027 [Google Scholar] [PubMed] [CrossRef]
Gentillon C, Li D, Duan M, Yu WM, Preininger MK et al. (2019). Targeting HIF-1α in combination with PPARα activation and postnatal factors promotes the metabolic maturation of human induced pluripotent stem cell-derived cardiomyocytes. Journal of Molecular and Cellular Cardiology 132: 120–135. https://doi.org/10.1016/j.yjmcc.2019.05.003 [Google Scholar] [PubMed] [CrossRef]
Ghosh AK (2020). p300 in cardiac development and accelerated cardiac aging. Aging and disease 11: 916–926. https://doi.org/10.14336/AD.2020.0401 [Google Scholar] [PubMed] [CrossRef]
Guo Y, Cao Y, Jardin BD, Sethi I, Ma Q et al. (2021). Sarcomeres regulate murine cardiomyocyte maturation through MRTF-SRF signaling. Proceedings of the National Academy of Sciences of the United States of America 118: 1–11. https://doi.org/10.1073/pnas.2008861118 [Google Scholar] [PubMed] [CrossRef]
Horikoshi Y, Yan Y, Terashvili M, Wells C, Horikoshi H, Fujita S, Bosnjak ZJ, Bai X (2019). Fatty acid-treated induced pluripotent stem cell-derived human cardiomyocytes exhibit adult cardiomyocyte-like energy metabolism phenotypes. Cells 8: 1095. https://doi.org/10.3390/cells8091095 [Google Scholar] [PubMed] [CrossRef]
Huss JM, Garbacz WG, Xie W (2015). Constitutive activities of estrogen-related receptors: Transcriptional regulation of metabolism by the ERR pathways in health and disease. Biochimica et Biophysica Acta—Molecular Cell Research 1852: 1912–1927. https://doi.org/10.1016/j.bbadis.2015.06.016 [Google Scholar] [PubMed] [CrossRef]
Iso H, Kobayashi M, Ishihara J, Sasaki S, Okada K, Kita Y, Kokubo Y, Tsugane S (2006). Intake of fish and n-3 fatty acids and risk of coronary heart disease among Japanese. Circulation 133: 195–202. https://doi.org/10.1161/CIRCULATIONAHA.105.581355 [Google Scholar] [PubMed] [CrossRef]
Isu G, Diaz DR, Grussenmeyer T, Gaudiello E, Eckstein F, Brink M, Marsano A (2019). Fatty acid-based monolayer culture to promote in vitro neonatal rat cardiomyocyte maturation. Biochimica et Biophysica Acta—Molecular Cell Research 1867: 118561. https://doi.org/10.1016/j.bbamcr.2019.118561 [Google Scholar] [PubMed] [CrossRef]
Jakic B, Buszko M, Cappellano G, Wick G (2017). Elevated sodium leads to the increased expression of HSP60 and induces apoptosis in HUVECs. PLoS One 12: e0179383. https://doi.org/10.1371/journal.pone.0179383 [Google Scholar] [PubMed] [CrossRef]
Karbassi E, Fenix A, Marchiano S, Muraoka N, Nakamura K, Yang X, Murry CE (2020). Cardiomyocyte maturation: Advances in knowledge and implications for regenerative medicine. Nature Reviews Cardiology 17: 341–359. https://doi.org/10.1038/s41569-019-0331-x [Google Scholar] [PubMed] [CrossRef]
Karimata T, Sato D, Seya D, Sato D, Wakatsuki T, Feng Z, Nishina A, Kusunoki M, Nakamura T (2013). Fatty acid composition in fetal, neonatal, and cultured cardiomyocytes in rats. In Vitro Cellular & Developmental Biology—Animal 49: 798–804. https://doi.org/10.1007/s11626-013-9668-3 [Google Scholar] [PubMed] [CrossRef]
Khairallah R, Kim J, O’Shea K, O’Connell K, Brown B et al. (2012). Improved mitochondrial function with diet-induced increase in either docosahexaenoic acid or arachidonic acid in membrane phospholipids. PLoS One 7: e34402. https://doi.org/10.1371/journal.pone.0034402 [Google Scholar] [PubMed] [CrossRef]
Kuppusamy KT, Jones DC, Sperber H, Madan A, Fischer KA et al. (2015). Let-7 family of microRNA is required for maturation and adult-like metabolism in stem cell-derived cardiomyocytes. Proceedings of the National Academy of Sciences of the United States of America 112: E2785–E2794. https://doi.org/10.1073/pnas.1424042112 [Google Scholar] [PubMed] [CrossRef]
Lamaziere A, Richard D, Bausero P, Barbe U, Kefi K, Wolf C, Visioli F (2015). Comparison of docosahexaenoic acid uptake in murine cardiomyocyte culture and tissue: Significance to physiologically relevant studies. Prostaglandins, Leukotrienes and Essential Fatty Acids 94: 49–54. https://doi.org/10.1016/j.plefa.2014.11.004 [Google Scholar] [PubMed] [CrossRef]
Lian X, Zhang J, Azarin SM, Zhu K, Hazeltine LB, Bao X, Hsiao C, Kamp TJ, Palecek SP (2013). Directed cardiomyocyte differentiation from human pluripotent stem cells by modulating Wnt/β-catenin signaling under fully defined conditions. Nature Protocols 8: 162–175. https://doi.org/10.1038/nprot.2012.150 [Google Scholar] [PubMed] [CrossRef]
Lopaschuk GD, Jaswal JS (2010). Energy metabolic phenotype of the cardiomyocyte during development, differentiation, and postnatal maturation. Journal of Cardiovascular Pharmacology 56: 130–140. https://doi.org/10.1097/FJC.0b013e3181e74a14 [Google Scholar] [PubMed] [CrossRef]
Lopez CA, Al-Siddiqi HHAA, Purnama U, Iftekhar S, Bruyneel AAN et al. (2021). Physiological and pharmacological stimulation for in vitro maturation of substrate metabolism in human induced pluripotent stem cell-derived cardiomyocytes. Scientific Reports 11: 7802. https://doi.org/10.1038/s41598-021-87186-y [Google Scholar] [PubMed] [CrossRef]
Luo XL, Zhang P, Liu X, Huang S, Rao SL, Ding Q, Yang HT (2021). Myosin light chain 2 marks differentiating ventricular cardiomyocytes derived from human embryonic stem cells. Pflügers Archiv—European Journal of Physiology 473: 991–1007. https://doi.org/10.1007/s00424-021-02578-3 [Google Scholar] [PubMed] [CrossRef]
Mallapaty S (2022). Pioneering stem-cell trials in Japan report promising results. Nature 609: 235. https://doi.org/10.1038/d41586-022-02232-7 [Google Scholar] [PubMed] [CrossRef]
Matsuzaka T, Shimano H, Yahagi N, Kato T, Atsumi A et al. (2007). Crucial role of a long chain fatty acid elongase, Elovl6, in obesity-induced insulin resistance. Nature Medicine 13: 1193–1202. https://doi.org/10.1038/nm1662 [Google Scholar] [PubMed] [CrossRef]
McCloy RA, Rogers S, Caldon CE, Lorca T, Castro A, Burgess A (2014). Partial inhibition of Cdk1 in G2 phase overrides the SAC and decouples mitotic events. Cell Cycle 13: 1400–1412. https://doi.org/10.4161/cc.28401 [Google Scholar] [PubMed] [CrossRef]
Miyagawa S, Kainuma S, Kawamura T, Suzuki K, Ito Y et al. (2022). Case report: Transplantation of human induced pluripotent stem cell-derived cardiomyocyte patches for ischemic cardiomyopathy. Frontiers in Cardiovascular Medicine 9: 950829. https://doi.org/10.3389/fcvm.2022.950829 [Google Scholar] [PubMed] [CrossRef]
Montaigne D, Butruille L, Staels B (2021). PPAR control of metabolism and cardiovascular functions. Nature Reviews Cardiology 18: 809–823. https://doi.org/10.1038/s41569-021-00569-6 [Google Scholar] [PubMed] [CrossRef]
Nakagawa O, Nakagawa M, Richardson JA, Olson EN, Srivastava D (1999). HRT1, HRT2, and HRT3: A new subclass of bHLH transcription factors marking specific cardiac, somitic, and pharyngeal arch segments. Developmental Biology 216: 72–84. https://doi.org/10.1006/dbio.1999.9454 [Google Scholar] [PubMed] [CrossRef]
Pasquier J, Gupta R, Rioult D, Hoarau-Véchot J, Courjaret R et al. (2017). Coculturing with endothelial cells promotes in vitro maturation and electrical coupling of human embryonic stem cell-derived cardiomyocytes. Journal of Heart and Lung Transplantation 36: 684–693. https://doi.org/10.1016/j.healun.2017.01.001 [Google Scholar] [PubMed] [CrossRef]
Ramachandra C, Mehta A, Wong P, Myu Mai Ja KP, Fritsche-Danielson R, Bhat R, Hausenloy D, Kovalik J, Shim W (2018). Fatty acid metabolism driven mitochondrial bioenergetics promotes. International Journal of Cardiology 272: 288–297. https://doi.org/10.1016/j.ijcard.2018.08.069 [Google Scholar] [PubMed] [CrossRef]
Reguera DP, Cunátová K, Vrbacktfytf M, Pecinová A, Houštek J, Mrácek T, Pecina P (2020). Cytochrome c oxidase subunit 4 isoform exchange results in modulation of oxygen affinity. Cells 9: 1–20. https://doi.org/10.3390/cells9020443 [Google Scholar] [PubMed] [CrossRef]
Ribeiro AJ, Ang YS, Fu JD, Rivas RN, Mohamed TM, Higgs GC, Srivastava D, Pruitt BL (2015). Contractility of single cardiomyocytes differentiated from pluripotent stem cells depends on physiological shape and substrate stiffness. Proceedings of the National Academy of Sciences of the United States of America 112: 12705–12710. https://doi.org/10.1073/pnas.1508073112 [Google Scholar] [PubMed] [CrossRef]
Ronaldson-Bouchard K, Ma SP, Yeager K, Chen T, Song L, Sirabella D, Morikawa K, Teles D, Yazawa M, Vunjak-Novakovic G (2018). Advanced maturation of human cardiac tissue grown from pluripotent stem cells. Nature 556: 239–243. https://doi.org/10.1038/s41586-018-0016-3 [Google Scholar] [PubMed] [CrossRef]
Rowe GC, Jiang A, Arany Z (2010). PGC-1 coactivators in cardiac development and disease. Circulation Research 107: 825–838. https://doi.org/10.1161/CIRCRESAHA.110.223818 [Google Scholar] [PubMed] [CrossRef]
Salameh A, Schneider P, Mqhlberg K, Hagendorff A, Dhein S, Pfeiffer D (2004). Chronic regulation of the expression of gap junction proteins connexin40, connexin43, and connexin45 in neonatal rat cardiomyocytes. European Journal of Pharmacology 503: 9–16. https://doi.org/10.1016/j.ejphar.2004.09.024 [Google Scholar] [PubMed] [CrossRef]
Schmitz G, Ecker J (2008). The opposing effects of n-3 and n-6 fatty acids. Progress in Lipid Research 47: 147–155. https://doi.org/10.1016/j.plipres.2007.12.004 [Google Scholar] [PubMed] [CrossRef]
Shysh AM, Nagibin VS, Kaplinskii SP, Dosenko VE (2016). N-3 long chain polyunsaturated fatty acids increase the expression of PPARγ-target genes and resistance of isolated heart and cultured cardiomyocytes to ischemic injury. Pharmacological Reports 68: 1133–1139. https://doi.org/10.1016/j.pharep.2016.06.013 [Google Scholar] [PubMed] [CrossRef]
Slaats RH, Schwach V, Passier R (2020). Metabolic environment in vivo as a blueprint for differentiation and maturation of human stem cell-derived cardiomyocytes. Biochimica et Biophysica Acta—Molecular Basis of Disease 1866: 165881. https://doi.org/10.1016/j.bbadis.2020.165881 [Google Scholar] [PubMed] [CrossRef]
Steinbusch LKM, Schwenk RW, Ouwens DM, Diamant M, Glatz JFC, Luiken JJFP (2011). Subcellular trafficking of the substrate transporters GLUT4 and CD36 in cardiomyocytes. Cellular and Molecular Life Sciences 68: 2525–2538. https://doi.org/10.1007/s00018-011-0690-x [Google Scholar] [PubMed] [CrossRef]
Takahashi K, Tanabe K, Ohnuki M, Narita M, Ichisaka T, Tomoda K, Yamanaka S (2007). Induction of pluripotent stem cells from adult human fibroblasts by defined factors. Cell 131: 861–872. https://doi.org/10.1016/j.cell.2007.11.019 [Google Scholar] [PubMed] [CrossRef]
Tani H, Tohyama S, Kishino Y, Kanazawa H, Fukuda K (2022). Production of functional cardiomyocytes and cardiac tissue from human induced pluripotent stem cells for regenerative therapy. Journal of Molecular and Cellular Cardiology 164: 83–91. https://doi.org/10.1016/j.yjmcc.2021.11.008 [Google Scholar] [PubMed] [CrossRef]
Tohyama S, Hattori F, Sano M, Hishiki T, Nagahata Y et al. (2013). Distinct metabolic flow enables large-scale purification of mouse and human pluripotent stem cell-derived cardiomyocytes. Cell Stem Cell 12: 127–137. https://doi.org/10.1016/j.stem.2012.09.013 [Google Scholar] [PubMed] [CrossRef]
Tu C, Chao B, Wu J (2018). Strategies for improving the maturity of human induced pluripotent stem cell-derived cardiomyocytes. Circulation Research 123: 512–514. https://doi.org/10.1161/CIRCRESAHA.118.313472 [Google Scholar] [PubMed] [CrossRef]
Wang T, McDonald C, Petrenko NB, Leblanc M, Wang T, Giguere V, Evans RM, Patel VV, Pei L (2015). Estrogen-related receptor α (ERRα) and ERRγ are essential coordinators of cardiac metabolism and function. Molecular and Cellular Biology 35: 1281–1298. https://doi.org/10.1128/MCB.01156-14 [Google Scholar] [PubMed] [CrossRef]
Yang X, Rodriguez M, Pabon L, Fischer KA, Reinecke H, Regnier M, Sniadecki NJ, Ruohola-Baker H, Murry CE (2014). Tri-iodo-l-thyronine promotes the maturation of human cardiomyocytes-derived from induced pluripotent stem cells. Journal of Molecular and Cellular Cardiology 72: 296–304. https://doi.org/10.1016/j.yjmcc.2014.04.005 [Google Scholar] [PubMed] [CrossRef]
Yang X, Rodriguez ML, Leonard A, Sun L, Fischer KA et al. (2019). Fatty acids enhance the maturation of cardiomyocytes derived from human pluripotent stem cells. Stem Cell Reports 13: 657–668. https://doi.org/10.1016/j.stemcr.2019.08.013 [Google Scholar] [PubMed] [CrossRef]
Yano M, Umehara Y, Kudo T, Nakamura T, Kosawada T, Nishina A, Sazuka M, Sato D, Feng Z (2021). Effects of docosahexaenoic acid or arachidonic acid supplementation on gene expression and contractile force of rat cardiomyocytes in primary culture. BIOCELL 45: 1213–1229. https://doi.org/10.32604/biocell.2021.016281 [Google Scholar] [CrossRef]
Yoshida A, Sekine W, Homma J, Sekine H, Itoyama YY, Sasaki D, Matsuura K, Kobayashi E, Shimizu T (2022). Development of appropriate fatty acid formulations to raise the contractility of constructed myocardial tissues. Regenerative Therapy 21: 413–423. https://doi.org/10.1016/j.reth.2022.09.006 [Google Scholar] [PubMed] [CrossRef]
Zuppinger C, Eppenberger-Eberhardt M, Eppenberger HM (2000). N-cadherin: Structure, function and importance in the formation of new intercalated disc-like cell contacts in cardiomyocytes. Heart Failure Reviews 5: 251–257. https://doi.org/10.1023/A:1009809520194 [Google Scholar] [PubMed] [CrossRef]
Cite This Article
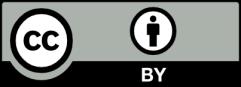