Open Access
REVIEW
Extracellular vesicles and angiotensin-converting enzyme 2 in COVID-19 disease
Department of Family and Community Medicine, Northwestern University Feinberg School of Medicine, Chicago, Illinois
* Corresponding Authors: YU LIU. Email: ; NATALIE J. S. CHOI. Email:
BIOCELL 2024, 48(1), 1-8. https://doi.org/10.32604/biocell.2023.031158
Received 18 May 2023; Accepted 06 November 2023; Issue published 30 January 2024
Abstract
Extracellular vesicles (EVs) are membranous vesicular structures released from almost all eukaryotic cell types under different physiological or pathological conditions. Growing evidence demonstrates that EVs can serve as mediators of intercellular communication between donor and recipient cells or microorganism-infected and noninfected cells. Coronavirus disease 2019 (COVID-19) disease is caused by infection of the severe acute respiratory syndrome coronavirus 2 (SARS-CoV-2) of host cells in the respiratory system and various extra-pulmonary tissue/organs, resulting in complications of multiple organ systems. As the cell surface receptor, angiotensin-converting enzyme 2 (ACE2) mediates cellular entry of SARS-CoV-2 into the host cells in patients with COVID-19. Recent studies have found that ACE2 can be released with EVs, which have been shown to interfere with the entry of the virus into host cells and thus may be involved in COVID-19 pathophysiology. In addition, ACE2, neprilysin (NEP), and thimet oligopeptidase (TOP) are the key enzymes that regulate angiotensin metabolism by converting angiotensin II or angiotensin I to angiotensin 1-7, the latter of which has protective effects in counterbalancing the harmful effects of angiotensin II in COVID-19 disease. This review summarizes the recent research progress regarding EV-associated ACE2, NEP, and TOP and the perspectives of their potential involvement in the pathophysiology of COVID-19 disease.Keywords
Cell membrane extracellular vesicles (EVs) are subcellular membrane structures which play physiological and pathological roles in human health and diseases [1,2]. EVs are classified into three groups based on their size, including exosomes (<100 nm), microvesicles (MVs) (<1 μm), and apoptotic bodies (1–5 μm) [1,3,4]. Exosomes are formed by the exocytosis of endosomal multivesicular bodies into the extracellular milieu [1,2]. MVs are membrane vesicles derived from the cell plasma membrane surface [1,2,5]. Apoptotic bodies are large membrane vesicles released in the late stages of apoptosis and can carry nuclear fragments and mitochondria [1,2]. EVs are generated during cell activation, senescence, or programmed cell death, i.e., apoptosis, necroptosis, pyroptosis, and NETosis (a unique neutrophil death) [1,2,4].
EVs are heterogeneous in size and composition [1,4–8]. Like their parental cells of origin, EVs have double-layer lipid membranes and may contain cellular components from the nucleus, cytoplasm, or cell membrane [1,4–8]. When EVs bud off of their parental cells, they harbor a vast array of bioactive molecules, including lipids, proteins, and nucleic acids (DNA, RNA, siRNA, microRNA, and lncRNA) [1,4–8]. EVs have been found in various biological fluids, including blood, urine, bronchoalveolar lavage fluid (BALF), and other body fluids, as well as in the tissues/organs of both humans and animals [1,2,4].
Over the past few years, severe acute respiratory syndrome coronavirus 2 (SARS-CoV-2) infection has caused a global pandemic of the coronavirus disease-2019 (COVID-19), resulting in over 771 million cases with over 6.96 million deaths worldwide according to statistical information from the World Health Organization [9]. The SARS-CoV-2 virus infects host cells by binding cell membrane surface angiotensin-converting enzyme 2 (ACE2), a viral entry receptor in the respiratory system and extra-pulmonary systemic tissue/organs in patients with COVID-19 disease [10,11]. Interestingly, membrane ACE2 can also be released with EVs (EV-ACE2) from SARS-CoV-2 infected cells (Table 1) [11,12], and elevated levels of EVs have been detected in patients with COVID-19 disease [13]. Classically, ACE2 is an enzyme with activities to convert angiotensin II to angiotensin 1-7 in the renal-angiotensin system (RAS) [5,14]. Importantly, several studies have reported elevated production of angiotensin II and decreased production of angiotensin 1-7 in COVID-19 patients [15,16]. In addition, several other enzymes, i.e., neprilysin (NEP) [17] and thimet oligopeptidase (TOP) [5,18], are also involved in angiotensin metabolism and production of angiotensin 1-7 (Fig. 1). Studies from our and other groups have found that both NEP [19] and TOP [18,20,21] can be released with EVs (Table 1). Here, we summarize recent research progress regarding EVs, their association with ACE2, NEP or TOP enzymes, and their relevant effect on angiotensin metabolism, as well as their potential involvement in SARS-CoV-2 infection and pathogenesis of COVID-19 disease.
Figure 1: Angiotensin metabolizing enzymes, EVs, and COVID-19 disease. Schematic illustration of angiotensin metabolism and angiotensin metabolizing enzymes, ACE2, NEP, and TOP, which can be released with EVs, as well as their roles in the metabolism of angiotensin I, and angiotensin II. This figure also highlights the potential involvements of angiotensin II, ACE2, and angiotensin 1-7 in COVID-19 disease. ACE2: angiotensin-converting enzyme 2; NEP: neprilysin; TOP: thimet oligopeptidase; EV: extracellular vesicles; COVID-19: coronavirus disease 2019; Mas receptor (MASR).
Extracellular vesicles and viral infection
Increasing evidence suggests that EVs play important roles in viral infections [1,4–6,8]. EVs can serve as vehicles for intercellular communication and the exchange of bioactive molecules between donor cells and recipient cells [1,4,8]. In addition, ligands or other cell surface molecules on EVs can bind to receptors of target cells, thus triggering intracellular signaling and resulting in inflammatory or immune responses [1,4,5,8].
EVs and viruses share similar physicochemical properties, such as small size and heterogenous size distribution [22]. Furthermore, viruses can utilize EV endocytic routes to enter noninfected cells and hijack the EV secretory pathway to exit infected cells. Thus, both share common cellular entry and biogenesis mechanisms [23,24]. Interestingly, a viral infection of cells can trigger the production of EVs through different mechanisms [25]. In turn, EVs from infected cells also play pathogenic roles in viral infectious diseases [26]. The EVs from infected cells may carry viral components or even entire virions and transfer viral components from infected cells to uninfected cells [23–25,27], thus promoting the spread of viral infection. In addition, Caobi et al. (2020) reported that EVs from virus-infected cells carry viral antigens that can be recognized by immune cells, leading to the activation of antiviral immune responses [28]. EVs from uninfected cells may contain antiviral interferons, inducing an antiviral state for restriction of viral replication [25,29].
Role of extracellular vesicles in COVID-19 disease
Like other viruses, SARS-CoV-2 can induce EV release from different cell types in infected patients [30,31]. In COVID-19 disease, the SARS-CoV-2 virus invades human host cells by infecting mucosal cells that express ACE2, the receptor for binding and entry of the SARS-CoV-2 virus into host cells [32–35]. The virus binds the host ACE2 receptor via two virus surface spike proteins [32]. As the host cell entry receptor, ACE2 likely plays a key role in determining host cell tropism and infectivity by the virus [32]. ACE2 is abundantly expressed not only in the respiratory system [10] but also in multiple extra-pulmonary tissues, directing the viral damage of host cells towards different organ systems [10,11,36]. Some studies have reported elevated ACE2 expression in infected organs of COVID-19 patients [37,38], while other studies suggest SARS-CoV-2 binding may result in downregulation of ACE2 expression [39,40]. There is, thus, significant controversy regarding the role of ACE2 in COVID-19 pathogenesis.
As discussed earlier, SARS-CoV-2 infection occurs through interaction between the viral spike protein and the host receptor ACE2 [29,41]. ACE2 has been detected in EVs from pulmonary cells in COVID-19 BALF [42,43], airway liquid secretions for pathological evaluation of various lung diseases [44,45], including COVID-19 lung diseases. It is noteworthy that EVs containing high levels of ACE2 in BALF from patients with severe COVID-19 are associated with reduced intensive care unit (ICU) and hospitalization times [43]. Furthermore, the level of ACE2 on the ACE2-EV surface is positively correlated with its ability to block SARS-CoV-2 [46]. El-Shennawy et al. (2022) reported that EV-associated ACE2 shows 135-fold higher potency in blocking the binding of SARS-CoV-2 viral spike protein to human host cells in vitro as compared to vesicle-free recombinant ACE2, thus blocking viral infection of host cells by acting as decoy sites of binding [11]. Importantly, studies using ACE2-expressing EVs generated from engineered cell lines, which were transfected with ACE2-expressing plasmids for stable ACE2 expression, have shown protective potential in the treatment of COVID-19 disease [11,43,47,48]. Therefore, engineered ACE2-EVs may serve as an alternative therapeutic strategy in addition to other treatment methods, while plasma levels of ACE2-EVs may serve as a clinical biomarker for the prognosis of COVID-19 disease severity.
EVs from viral infected host cells contain viral components, including viral RNA and proteins, which may contribute to viral replication and immune evasion [30,31]. In addition, EVs also carry host proteins, i.e., ACE2, CD9, transmembrane serine protease 2 (TMPRSS2), which can mediate the viral entry process and COVID-19 infection by aiding in viral incorporation into host cells [41,49–52]. ACE2-carrying EVs can compete with host cell surface ACE2, blocking SARS-CoV-2 virus binding and infection of host cells [11]. However, ACE2-carrying EVs may also be transferred to target receptor-null cells, thus making these previously naïve cells more susceptible to viral infection [53]. In addition, EV-associated TMPRSS2 can cleave the spike protein of the SARS-CoV-2 virus, which enables the virus to bind to the receptor, and subsequently enter host cells [49,52]. Previous studies have shown that CD9 may collaborate with TMPRSS2 in cleaving viral fusion glycoproteins and facilitate quick entry of coronavirus (e.g., MERS-CoV) into lung cells [54]. Studies also showed that membrane surface CD9 may also be involved in entry and exit mechanisms in respiratory viruses, including SARS-CoV-2 virus [48,55–57].
EVs have been implicated in the progression of cytokine storm, the main mechanism for severe illness and mortality in COVID-19 patients [50]. EVs derived from immune cells or lung epithelial cells in COVID-19 patients carry pro-inflammatory cytokines, chemokines, and damage-associated molecular patterns (DAMPs) [29,58], which all enhance inflammatory responses. An array of lung cells, such as epithelial cells, endothelial cells, and alveolar macrophages, can all release EVs [50,59], contributing to elevated levels of EVs in COVID-19 patients. Higher concentrations of EVs have been detected in the pulmonary edema fluid of patients with acute respiratory distress syndrome compared to controls [60]. In lipopolysaccharide (LPS)-induced cytokine storm models, EVs in BALF can initiate inflammatory responses in the lung via enhanced expression of tumor necrosis factor-α, interleukin-6, and junction proteins [61]. Administering natural sphingomyelinase inhibitor GW4869 in LPS-induced lung inflammation mouse models significantly decreased the level of pro-inflammatory cytokines and reduced lung elastance and alveolar collapse, thus providing protection against the effects of cytokine storm [62], probably due to the inhibitory effects of GW4869 on EV generation [63]. These studies support the involvement of EVs in initiating and aggravating cytokine storm.
It has long been known that EVs carry molecules, i.e., tissue factor (TF) and phosphatidylserine (PS), with pro-coagulant activities [1,64], which is also important in propagation of the pro-coagulant state in certain serious complications of COVID-19 disease [13,65]. EVs from COVID-19 patients have been shown to carry increased levels of biologically active TF [66–68], a key initiator of the extrinsic coagulation pathway, or membrane PS [69], an essential cofactor of coagulation, on EV surfaces. In fact, TF-EV levels were markedly higher when associated with COVID-19 and demonstrated increased fibrinolytic activities as compared to coagulation processes associated with septic shock in non-COVID-19 cases [66,70]. TF-EV activity has been associated with COVID-19 disease severity and mortality [67]. In addition, levels of TF-EV activity have been found to correlate with plasma D-dimer, which is known to be associated with thrombosis in COVID-19 [67]. Furthermore, SARS-CoV-2 infection causes systemic inflammation and over-activation of the immune system along with endothelial dysfunction and platelet activation, which are the known functions of EVs in immune system-related diseases [64,71]. All of the above lead to systemic pro-thrombotic states, which result in micro-thrombosis and multi-organ damage and failure in patients with COVID-19 [70,72].
Extracellular vesicles, ACE2, angiotensin 1-7 axis and COVID-19 disease
In addition to being the viral entry receptor for SARS-CoV-2 infection [10], ACE2 classically functions as an enzyme in the renin–angiotensin system (RAS) to catalyze angiotensin metabolism and regulate the balance between angiotensin II and angiotensin 1-7 (Table 1), contributing to systemic complications of COVID-19 disease [51,73]. ACE2 is a transmembrane enzyme with a short cytoplasmic domain, a transmembrane domain, a catalytic ectodomain, and an amino-terminal signal peptide [73–75]. ACE2 can be detected as both membrane bound and solute forms [75]. Both membrane-bound and soluble forms of ACE2 can actively cleave angiotensin II [75]. Recent studies have found that ACE2 can be either released as full-length molecules with EVs (EV-ACE2) [11,12] or shed as ectodomain ACE2 exomeres (ecto-ACE2) through cleavage by ADAM10/17 [12,76]. Both forms of EV-associated ACE2 can bind to SARS-CoV-2 and inhibit its infection [12]. Levels of ACE2-positive EVs are associated with the severity of COVID-19 disease, indicating their defensive properties for maintaining homeostatic conditions in COVID-19 patients [11]. Studies have also reported the increased production of angiotensin II and decreased production of angiotensin 1-7 in COVID-19 patients [15,16,77], while the balance of angiotensin II in angiotensin 1-7 is regulated by ACE2 [5,14]. However, angiotensin 1-7 also has regulatory effects on ACE2 expression, and continuous infusion of angiotensin 1-7 has shown organ-specific downregulation effects on local ACE2 expression in vivo in rats [78]. Therefore, there may be a tightly regulated equilibrium between angiotensin I/II and angiotensin 1-7 as well as ACE/ACE2. In the context of COVID-19, a few studies have shown that SARS-CoV-2 binding can downregulate ACE2 expression [39,40].
As the major biologically active effector peptide of the RAS system [79–81], angiotensin II can activate AT1 (angiotensin II receptor type 1) receptor and trigger pro-inflammatory, prooxidative, pro-fibrotic, pro-thrombotic and vasoconstrictive effects, contributing to the severity of COVID-19 disease (Fig. 1) [73,79–81]. In contrast, angiotensin 1-7 has a range of anti-inflammatory, antioxidant, vasodilatory, and natriuretic protective effects through the G protein-coupled receptor (GPCR) MAS receptor (Fig. 1) [73,79,80]. Furthermore, angiotensin 1-7 also has a critical role in protecting against lung inflammation and fibrosis [79,80]. Recent studies have reported a potential link between angiotensin 1-7 and COVID-19 disease severity [82–86]. Decreased levels of blood angiotensin 1-7 have been reported in COVID-19 patients who were either severely ill or have died [83,84,86]. In contrast, higher levels of angiotensin 1-7 have been associated with reduced COVID-19 disease severity [83,84,86]. Furthermore, dysregulation of ACE2, angiotensin I/II, and angiotensin 1-7 are associated with mortality and end-organ damage in COVID-19 patients [87,88]. In fact, angiotensin 1-7 peptide replacement therapy has been proposed for the treatment of severe COVID-19 [79,85,89]. Both membrane-bound and soluble forms of ACE2 can actively cleave angiotensin II to angiotensin 1-7 [75], which has anti-inflammatory, antioxidant, and vasodilatory effects. Thus, EV-associated ACE2 may also be involved in COVID-19 by regulating the conversion of angiotensin II metabolism [75]. Although no relevant studies have yet been reported, it would be worthwhile to investigate the catalytic activities of circulating EV-associated ACE2 and its effects on the metabolism of angiotensin I/II and angiotensin 1-7, as well as their relevance to the severity of COVID-19 disease. Interestingly, several studies have reported that treatment with ACE inhibitors or angiotensin receptor blockers (ARBs) was associated with lower levels of inflammation and reduced risk of COVID-19 disease [90,91]. However, meta-analyses [92,93] and a randomized clinical trial [94] did not support the findings of these initial studies.
Extracellular vesicles, Neprilysin and Thimet Oligopeptidase, and COVID-19 diseases
In addition to ACE2, NEP and TOP are endopeptidases that can convert angiotensin I to angiotensin 1-7 by cleaving internal peptide bonds [5,17,18]. Interestingly, both NEP and TOP can be released with EVs based on recent studies from our and other publications [5,18,19]. Importantly, EV-associated NEP and TOP are enzymatically bioactive (Fig. 1) [5,18,19].
A recent study reported that NEP inhibitor sacubitril can inhibit further cleavage of angiotensin 1-7 [17], and therefore, may favor the preservation of angiotensin 1-7 [17]. Studies have shown the beneficial effects of sacubitril on the regulation of angiotensin 1-7 and the improvement of outcomes of COVID-19 disease [15,17,95–97]. Thus, several clinical trials using neprilysin inhibitor sacubitril have been proposed for the treatment of COVID-19 for a multi-targeted therapeutic approach [15,98]. In fact, sacubitril administration has shown the beneficial effects in COVID-19 patients with lung or cardiovascular complications [15,17,98]. NEP is abundantly expressed in many tissues/organs, including the lungs [99]. The above studies provide insights and also indicate the potential importance of NEP in COVID-19 pathogenesis. The roles of EV-associated NEP in the pathophysiology of COVID-19 disease, the plasma levels of NEP-positive EVs and their existence in BALF in COVID-19 patients, and their association with disease severity of COVID-19 have not yet been investigated. These are important questions to be explored in future studies.
TOP is primarily located in the cytosol, but it can also be associated with the cell membrane or secreted into the extracellular space (Table 1) [5,18]. About 20–25% of total TOP enzyme activity is associated with membrane fractions [100], and TOP has been visualized on the plasma membrane surface by confocal microscopy [5,18,101]. Interestingly, we have recently demonstrated that membrane-associated TOP can be released with EVs to the extracellular space [18,20,21]. Most importantly, EV-associated TOP exhibits considerable enzymatic activity [5,18,20,21]. Our findings suggest that EV-associated TOP might be a previously unrecognized, novel form of extracellular TOP [5,18,20,21], in addition to its soluble form in the extracellular milieu. The soluble form of TOP easily diffuses into the circulation and can be diluted by large volumes of systemic circulation. In contrast, EV-associated TOP may stay in the microenvironment in relatively higher concentrations [18,20,21]. Thus, the EV-associated format of TOP may enable the enzyme to work more potently in the local tissue/organs on its substrates, i.e., angiotensin I, and contribute to the progression of the relevant pathological conditions. Since TOP is known to convert angiotensin I in vitro to the biologically active peptide angiotensin 1-7 [102], one may expect that bioactive EV-associated TOP may also be able to convert angiotensin I to angiotensin 1-7. TOP converts angiotensin I to angiotensin 1-7, providing a path to side-step angiotensin II of the regular RAAS pathway, which has pro-inflammatory effects. TOP is expressed by various tissues/organs, including the lungs [103]. TOP has been proposed to be involved in COVID-19 disease [5,104,105] due to its role in converting angiotensin I to angiotensin 1-7, being able to offset the harmful effects of angiotensin II in favor of the anti-inflammatory and lung protective effects of angiotensin 1-7. Thus, the potential involvement of TOP and EV-associated TOP in pathological roles in COVID-19 disease would be worthwhile to investigate. A better understanding of TOP and EV-associated TOP in COVID-19 disease may provide insights into their role in clinical diagnosis, prognosis, and development of new therapeutic strategies.
In this viewpoint, we summarized the role of EVs in the pathogenesis of COVID-19 disease, including the role that EVs may play in propagating viral infection, promoting pro-thrombotic conditions, and enhancing the progression of cytokine storm in COVID-19 disease. The current paper has discussed recent progress regarding EV-associated ACE2 and its effects on interfering with viral entry into host cells and the therapeutic potential of engineered EV-associated ACE2 on COVID-19 disease based on its function of serving as the cell surface receptor for SARS-CoV-2. Based on the enzymatic activities of ACE2, which converts angiotensin II to angiotensin 1-7, and TOP and NEP which convert angiotensin I to angiotensin 1-7, we have discussed their EV-associated forms and the perspective insights regarding their potential involvements in COVID-19 disease through their functions in angiotensin metabolism. Through their enzymatic activity on the RAAS pathway, ACE2, NEP, and TOP may be able to offset the pro-inflammatory and harmful effects of angiotensin II in favor of the anti-inflammatory and lung protective effects of angiotensin 1-7. The roles of EVs and their associated ACE2, NEP, and TOP are complicated, and the potential roles of EV-associated ACE2, NEP, and TOP would thus be novel areas worthwhile of investigation and would provide insights into therapeutic strategy, diagnosis, and prognosis of COVID-19 disease.
Acknowledgement: The authors appreciate Dr. Adele J Wolfson for her previous guidance on the thimet oligopeptidase and extracellular vesicle research.
Funding Statement: The authors received no specific funding for this study.
Author Contributions: The authors confirm contribution to the paper as follows: study conception and design: YL; data collection: YL; analysis and interpretation of results: YL, NJSC; draft manuscript preparation: YL, NJSC, RJK. All authors reviewed the results and approved the final version of the manuscript.
Availability of Data and Materials: Data sharing not applicable to this article as no datasets were generated or analyzed during the current study.
Ethics Approval: Not applicable.
Conflicts of Interest: The authors declare that they have no conflicts of interest to report regarding the present study.
References
1. Wu X, Liu Y, Wei W, Liu ML. Extracellular vesicles in autoimmune vasculitis–Little dirts light the fire in blood vessels. Autoimmun Rev. 2019;18(6):593–606. [Google Scholar] [PubMed]
2. Zhao Y, Wei W, Liu ML. Extracellular vesicles and lupus nephritis–New insights into pathophysiology and clinical implications. J Autoimmun. 2020;115:102540. [Google Scholar] [PubMed]
3. Thery C, Witwer KW, Aikawa E, Alcaraz MJ, Anderson JD, Andriantsitohaina R, et al. Minimal information for studies of extracellular vesicles 2018 (MISEV2018a position statement of the international society for extracellular vesicles and update of the MISEV2014 guidelines. J Extracell Vesicles. 2018;7:1535750. [Google Scholar] [PubMed]
4. Buzas EI. The roles of extracellular vesicles in the immune system. Nat Rev Immunol. 2022;23(4):236–50. [Google Scholar] [PubMed]
5. Liu Y, Sigman JA, Bruce L, Wolfson A. Thimet oligopeptidase—A classical enzyme with new function and new form. Immuno. 2021;1:332–46. [Google Scholar]
6. Li M, Yu D, Williams KJ, Liu ML. Tobacco smoke induces the generation of procoagulant microvesicles from human monocytes/macrophages. Arterioscler Thromb Vasc Biol. 2010;30:1818–24. [Google Scholar] [PubMed]
7. Tkach M, Thery C. Communication by extracellular vesicles: where we are and where we need to go. Cell. 2016;164(6):1226–32. [Google Scholar] [PubMed]
8. van Niel G, D’Angelo G, Raposo G. Shedding light on the cell biology of extracellular vesicles. Nat Rev Mol Cell Biol. 2018;19:213–28. [Google Scholar] [PubMed]
9. WHO Coronavirus (COVID-19) Dashboard. https://covid19.who.int/. [Accessed 2022]. [Google Scholar]
10. Gupta A, Madhavan MV, Sehgal K, Nair N, Mahajan S, Sehrawat TS, et al. Extrapulmonary manifestations of COVID-19. Nat Med. 2020;26(7):1017–32. [Google Scholar] [PubMed]
11. El-Shennawy L, Hoffmann AD, Dashzeveg NK, McAndrews KM, Mehl PJ, Cornish D, et al. Circulating ACE2-expressing extracellular vesicles block broad strains of SARS-CoV-2. Nat Commun. 2022;13(1):405. [Google Scholar] [PubMed]
12. Zhang Q, Jeppesen DK, Higginbotham JN, Franklin JL, Crowe Jr JE, Coffey RJ. Angiotensin-converting enzyme 2-containing small extracellular vesicles and exomeres bind the severe acute respiratory syndrome Coronavirus 2 spike protein. Gastroenterology. 2021;160(3):958–61.e3. [Google Scholar] [PubMed]
13. Aharon A, Dangot A, Kinaani F, Zavaro M, Bannon L, Bar-Lev T, et al. Extracellular vesicles of COVID-19 patients reflect inflammation, thrombogenicity, and disease severity. Int J Mol Sci. 2023;24(6):5918. [Google Scholar] [PubMed]
14. Pereira MG, Souza LL, Becari C, Duarte DA, Camacho FR, Oliveira JA, et al. Angiotensin II-independent angiotensin-(1-7) formation in rat hippocampus: involvement of Thimet Oligopeptidase. Hypertension. 2013;62(5):879–85. [Google Scholar] [PubMed]
15. Acanfora D, Ciccone MM, Scicchitano P, Acanfora C, Casucci G. Neprilysin inhibitor-angiotensin II receptor blocker combination (sacubitril/valsartanrationale for adoption in SARS-CoV-2 patients. Eur Heart J Cardiovasc Pharmacother. 2020;6(3):135–6. [Google Scholar] [PubMed]
16. Bellis A, Mauro C, Barbato E, Trimarco B, Morisco C. The rationale for angiotensin receptor neprilysin inhibitors in a multi-targeted therapeutic approach to COVID-19. Int J Mol Sci. 2020;21(22):8612. [Google Scholar] [PubMed]
17. Esser N, Zraika S. Neprilysin inhibitors and angiotensin(1-7) in COVID-19. Br J Cardiol. 2020;27(4):109–11. [Google Scholar] [PubMed]
18. Liu Y, Bruce L, Wolfson A. Extracellular thimet oligopeptidase is released with extracellular vesicles from human prostate cancer cells. bioRxiv. 2020. doi:https://doi.org/10.1101/2020.05.10.087304. [Google Scholar] [CrossRef]
19. Gill M, Motta-Mejia C, Kandzija N, Cooke W, Zhang W, Cerdeira AS, et al. Placental syncytiotrophoblast-derived extracellular vesicles carry active NEP (Neprilysin) and are increased in preeclampsia. Hypertension. 2019;73(5):1112–9. [Google Scholar] [PubMed]
20. Liu Y, Bruce LA, Wolfson AJ. Extracellular Thimet Oligopeptidase is carried by cell membrane microvesicles of prostate cancer cells. The FASEB Journal. 2013;27:995.2. doi:https://doi.org/10.1096/fasebj.27.1_supplement.995.2. [Google Scholar] [CrossRef]
21. Liu Y. Extracellular Thimet Oligopeptidase is carried by cell membrane microvesicles of human prostate cancer cells. (Academic Theses). Wellesley College: USA; 2013. [Google Scholar]
22. Pocsfalvi G, Mammadova R, Ramos Juarez AP, Bokka R, Trepiccione F, Capasso G. COVID-19 and extracellular vesicles: an intriguing interplay. Kidney Blood Press Res. 2020;45(5):661–70. [Google Scholar] [PubMed]
23. Raab-Traub N, Dittmer DP. Viral effects on the content and function of extracellular vesicles. Nat Rev Microbiol. 2017;15:559–72. [Google Scholar] [PubMed]
24. Urbanelli L, Buratta S, Tancini B, Sagini K, Delo F, Porcellati S, et al. The role of extracellular vesicles in viral infection and transmission. Vaccines. 2019;7(3):102. [Google Scholar] [PubMed]
25. Martins ST, Alves LR. Extracellular vesicles in viral infections: two sides of the same coin? Front Cell Infect Microbiol. 2020;10:593170. [Google Scholar] [PubMed]
26. Yoshikawa FSY, Teixeira FME, Sato MN, Oliveira L. Delivery of microRNAs by extracellular vesicles in viral infections: could the news be packaged? Cells. 2019;8(6):611. [Google Scholar] [PubMed]
27. O’Brien K, Breyne K, Ughetto S, Laurent LC, Breakefield XO. RNA delivery by extracellular vesicles in mammalian cells and its applications. Nat Rev Mol Cell Biol. 2020;21(10):585–606. [Google Scholar] [PubMed]
28. Caobi A, Nair M, Raymond AD. Extracellular vesicles in the pathogenesis of viral infections in humans. Viruses. 2020;12(10):1200. [Google Scholar] [PubMed]
29. Tahyra ASC, Calado RT, Almeida F. The role of extracellular vesicles in COVID-19 pathology. Cells. 2022;11(16):2496. [Google Scholar] [PubMed]
30. Hernandez-Diazcouder A, Diaz-Godinez C, Carrero JC. Extracellular vesicles in COVID-19 prognosis, treatment, and vaccination: an update. Appl Microbiol Biotechnol. 2023;107(7–8):2131–41. [Google Scholar] [PubMed]
31. Xia B, Pan X, Luo RH, Shen X, Li S, Wang Y, et al. Extracellular vesicles mediate antibody-resistant transmission of SARS-CoV-2. Cell Discov. 2023;9(1):2. [Google Scholar] [PubMed]
32. Kuriakose J, Montezano AC, Touyz RM. ACE2/Ang-(1-7)/Mas1 axis and the vascular system: Vasoprotection to COVID-19-associated vascular disease. Clin Sci. 2021;135(2):387–407. [Google Scholar]
33. Wang Q, Zhang Y, Wu L, Niu S, Song C, Zhang Z, et al. Structural and functional basis of SARS-CoV-2 entry by using human ACE2. Cell. 2020;181(4):894–904. [Google Scholar] [PubMed]
34. Jha NK, Ojha S, Jha SK, Dureja H, Singh SK, Shukla SD, et al. Evidence of Coronavirus (CoV) pathogenesis and emerging pathogen SARS-CoV-2 in the nervous system: a review on neurological impairments and manifestations. J Mol Neurosci. 2021;71(11):2192–209. [Google Scholar] [PubMed]
35. Yan R, Zhang Y, Li Y, Xia L, Guo Y, Zhou Q. Structural basis for the recognition of SARS-CoV-2 by full-length human ACE2. Science. 2020;367(6485):1444–8. [Google Scholar] [PubMed]
36. Vaduganathan M, Vardeny O, Michel T, McMurray JJV, Pfeffer MA, Solomon SD. Renin-angiotensin-aldosterone system inhibitors in patients with COVID-19. N Engl J Med. 2020;382(17):1653–9. [Google Scholar] [PubMed]
37. van Lier D, Kox M, Santos K, van der Hoeven H, Pillay J, Pickkers P. Increased blood angiotensin converting enzyme 2 activity in critically ill COVID-19 patients. ERJ Open Res. 2021;7(1):00848-2020. [Google Scholar] [PubMed]
38. Gheware A, Ray A, Rana D, Bajpai P, Nambirajan A, Arulselvi S, et al. ACE2 protein expression in lung tissues of severe COVID-19 infection. Sci Rep. 2022;12(1):4058. [Google Scholar] [PubMed]
39. Chaudhry F, Lavandero S, Xie X, Sabharwal B, Zheng YY, Correa A, et al. Manipulation of ACE2 expression in COVID-19. Open Heart. 2020;7(2):e001424. [Google Scholar] [PubMed]
40. Bourgonje AR, Abdulle AE, Timens W, Hillebrands JL, Navis GJ, Gordijn SJ, et al. Angiotensin-converting enzyme 2 (ACE2SARS-CoV-2 and the pathophysiology of coronavirus disease 2019 (COVID-19). J Pathol. 2020;251(3):228–48. [Google Scholar] [PubMed]
41. Hassanpour M, Rezaie J, Nouri M, Panahi Y. The role of extracellular vesicles in COVID-19 virus infection. Infect Genet Evol. 2020;85:104422. [Google Scholar] [PubMed]
42. Wang F, Fang B, Qiang X, Shao J, Zhou L. The efficacy of mesenchymal stromal cell-derived therapies for acute respiratory distress syndrome-a meta-analysis of preclinical trials. Respir Res. 2020;21(1):307. [Google Scholar] [PubMed]
43. Ching KL, de Vries M, Gago J, Dancel-Manning K, Sall J, Rice WJ, et al. ACE2-containing defensosomes serve as decoys to inhibit SARS-CoV-2 infection. PLoS Biol. 2022;20(9):e3001754. [Google Scholar] [PubMed]
44. Pastor L, Vera E, Marin JM, Sanz-Rubio D. Extracellular vesicles from airway secretions: new insights in lung diseases. Int J Mol Sci. 2021;22(2):583. [Google Scholar] [PubMed]
45. Liu Z, Yan J, Tong L, Liu S, Zhang Y. The role of exosomes from BALF in lung disease. J Cell Physiol. 2022;237(1):161–8. [Google Scholar] [PubMed]
46. Cocozza F, Nevo N, Piovesana E, Lahaye X, Buchrieser J, Schwartz O, et al. Extracellular vesicles containing ACE2 efficiently prevent infection by SARS-CoV-2 Spike protein-containing virus. J Extracell Vesicles. 2020;10:e12050. [Google Scholar] [PubMed]
47. Rao L, Xia S, Xu W, Tian R, Yu G, Gu C, et al. Decoy nanoparticles protect against COVID-19 by concurrently adsorbing viruses and inflammatory cytokines. Proc Natl Acad Sci. 2020;117(44):27141–7. [Google Scholar] [PubMed]
48. Kim HK, Cho J, Kim E, Kim J, Yang JS, Kim KC, et al. Engineered small extracellular vesicles displaying ACE2 variants on the surface protect against SARS-CoV-2 infection. J Extracell Vesicles. 2022;11(1):e12179. [Google Scholar] [PubMed]
49. Yan YY, Zhou WM, Wang YQ, Guo QR, Zhao FX, Zhu ZY, et al. The potential role of extracellular vesicles in COVID-19 treatment: opportunity and challenge. Front Mol Biosci. 2021;8:699929. [Google Scholar] [PubMed]
50. Xia X, Yuan P, Liu Y, Wang Y, Cao W, Zheng JC. Emerging roles of extracellular vesicles in COVID-19, a double-edged sword? Immunology. 2021;163:416–30. [Google Scholar] [PubMed]
51. Ni W, Yang X, Yang D, Bao J, Li R, Xiao Y, et al. Role of angiotensin-converting enzyme 2 (ACE2) in COVID-19. Crit Care. 2020;24(1):422. [Google Scholar] [PubMed]
52. Tey SK, Yam JWP. The importance of activated TMPRSS2 in the proviral role of small extracellular vesicles in SARS-CoV-2 infection. J Extracell Vesicles. 2022;11(12):e12296. [Google Scholar] [PubMed]
53. Wang J, Chen S, Bihl J. Exosome-mediated transfer of ACE2 (angiotensin-converting enzyme 2) from endothelial progenitor cells promotes survival and function of endothelial cell. Oxid Med Cell Longev. 2020;2020:4213541–11. [Google Scholar] [PubMed]
54. Earnest JT, Hantak MP, Li K, McCray PBJr., Perlman S, Gallagher T. The tetraspanin CD9 facilitates MERS-coronavirus entry by scaffolding host cell receptors and proteases. PLoS Pathog. 2017;13(7):e1006546. [Google Scholar] [PubMed]
55. Choi D, Khan N, Montermini L, Tawil N, Meehan B, Kim DK, et al. Quantitative proteomics and biological activity of extracellular vesicles engineered to express SARS-CoV-2 spike protein. J Extracell Biol. 2022;1(10):e58. [Google Scholar] [PubMed]
56. Healy EF. How tetraspanin-mediated cell entry of SARS-CoV-2 can dysregulate the shedding of the ACE2 receptor by ADAM17. Biochem Biophys Res Commun. 2022;593:52–6. [Google Scholar] [PubMed]
57. Malla R, Kamal MA. Tetraspanin-enriched microdomain containing CD151, CD9, and TSPAN 8-potential mediators of entry and exit mechanisms in respiratory viruses including SARS-CoV-2. Curr Pharm Des. 2022;28(46):3649–57. [Google Scholar] [PubMed]
58. Puhm F, Flamand L, Boilard E. Platelet extracellular vesicles in COVID-19: potential markers and makers. J Leukoc Biol. 2022;111(1):63–74. [Google Scholar] [PubMed]
59. Moon HG, Cao Y, Yang J, Lee JH, Choi HS, Jin Y. Lung epithelial cell-derived extracellular vesicles activate macrophage-mediated inflammatory responses via ROCK1 pathway. Cell Death Dis. 2015;6(12):e2016. [Google Scholar] [PubMed]
60. Bastarache JA, Fremont RD, Kropski JA, Bossert FR, Ware LB. Procoagulant alveolar microparticles in the lungs of patients with acute respiratory distress syndrome. Am J Physiol Lung Cell Mol Physiol. 2009;297(6):L1035–41. [Google Scholar] [PubMed]
61. Yuan C, Ai K, Xiang M, Xie C, Zhao M, Wu M, et al. Novel 1-hydroxy phenothiazinium-based derivative protects against bacterial sepsis by inhibiting AAK1-mediated LPS internalization and caspase-11 signaling. Cell Death Dis. 2022;13(8):722. [Google Scholar] [PubMed]
62. Okuro RT, Machado MN, Casquilho NV, Jardim-Neto A, Roncally-Carvalho A, Atella GC, et al. The role of sphingolipid metabolism disruption on lipopolysaccharide-induced lung injury in mice. Pulm Pharmacol Ther. 2018;50:100–10. [Google Scholar] [PubMed]
63. Catalano M, O’Driscoll L. Inhibiting extracellular vesicles formation and release: a review of EV inhibitors. J Extracell Vesicles. 2020;9(1):1703244. [Google Scholar] [PubMed]
64. Liu ML, Williams KJ, Werth VP. Microvesicles in autoimmune diseases. Adv Clin Chem. 2016;77:125–75. [Google Scholar] [PubMed]
65. Setua S, Thangaraju K, Dzieciatkowska M, Wilkerson RB, Nemkov T, Lamb DR, et al. Coagulation potential and the integrated omics of extracellular vesicles from COVID-19 positive patient plasma. Sci Rep. 2022;12:22191. [Google Scholar] [PubMed]
66. Guervilly C, Bonifay A, Burtey S, Sabatier F, Cauchois R, Abdili E, et al. Dissemination of extreme levels of extracellular vesicles: tissue factor activity in patients with severe COVID-19. Blood Adv. 2021;5(3):628–34. [Google Scholar] [PubMed]
67. Rosell A, Havervall S, von Meijenfeldt F, Hisada Y, Aguilera K, Grover SP, et al. Patients with COVID-19 have elevated levels of circulating extracellular vesicle tissue factor activity that is associated with severity and mortality-brief report. Arterioscler Thromb Vasc Biol. 2021;41(2):878–82. [Google Scholar] [PubMed]
68. Balbi C, Burrello J, Bolis S, Lazzarini E, Biemmi V, Pianezzi E, et al. Circulating extracellular vesicles are endowed with enhanced procoagulant activity in SARS-CoV-2 infection. EBioMedicine. 2021;67:103369. [Google Scholar] [PubMed]
69. Zaid Y, Puhm F, Allaeys I, Naya A, Oudghiri M, Khalki L, et al. Platelets can associate with SARS-Cov-2 RNA and are hyperactivated in COVID-19. Circ Res. 2020;127(11):1404–18. [Google Scholar] [PubMed]
70. Iba T, Wada H, Levy JH. Platelet activation and thrombosis in COVID-19. Semin Thromb Hemost. 2023;49(1):55–61. [Google Scholar] [PubMed]
71. Liu ML, Williams KJ. Microvesicles: potential markers and mediators of endothelial dysfunction. Curr Opin Endocrinol Diabetes Obes. 2012;19(2):121–7. [Google Scholar] [PubMed]
72. Tan BK, Mainbourg S, Friggeri A, Bertoletti L, Douplat M, Dargaud Y, et al. Arterial and venous thromboembolism in COVID-19: a study-level meta-analysis. Thorax. 2021;76(10):970–9. [Google Scholar] [PubMed]
73. Sharma RK, Stevens BR, Obukhov AG, Grant MB, Oudit GY, Li Q, et al. ACE2 (angiotensin-converting enzyme 2) in cardiopulmonary diseases: ramifications for the control of SARS-CoV-2. Hypertension. 2020;76(3):651–61. [Google Scholar] [PubMed]
74. Daniell H, Nair SK, Shi Y, Wang P, Montone KT, Shaw PA, et al. Decrease in angiotensin-converting enzyme activity but not concentration in plasma/lungs in COVID-19 patients offers clues for diagnosis/treatment. Mol Ther Methods Clin Dev. 2022;26:266–78. [Google Scholar] [PubMed]
75. Yalcin HC, Sukumaran V, Al-Ruweidi M, Shurbaji S. Do changes in ACE-2 expression affect SARS-CoV-2 virulence and related complications: a closer look into membrane-bound and soluble forms. Int J Mol Sci. 2021;22(13):6703. [Google Scholar] [PubMed]
76. Niehues RV, Wozniak J, Wiersch F, Lilienthal E, Tacken N, Schumertl T, et al. The collectrin-like part of the SARS-CoV-1 and -2 receptor ACE2 is shed by the metalloproteinases ADAM10 and ADAM17. FASEB J. 2022;36(3):e22234. [Google Scholar] [PubMed]
77. Camargo RL, Bombassaro B, Monfort-Pires M, Mansour E, Palma AC, Ribeiro LC, et al. Plasma angiotensin II is increased in critical coronavirus disease 2019. Front Cardiovasc Med. 2022;9:847809. [Google Scholar] [PubMed]
78. Tan Z, Wu J, Ma H. Regulation of angiotensin-converting enzyme 2 and Mas receptor by Ang-(1-7) in heart and kidney of spontaneously hypertensive rats. J Renin Angiotensin Aldosterone Syst. 2011;12(4):413–9. [Google Scholar] [PubMed]
79. Peiro C, Moncada S. Substituting angiotensin-(1-7) to prevent lung damage in SARS-CoV-2 infection? Circulation. 2020;141:1665–6. [Google Scholar] [PubMed]
80. Brojakowska A, Narula J, Shimony R, Bander J. Clinical implications of SARS-CoV-2 interaction with renin angiotensin system: JACC review topic of the week. J Am Coll Cardiol. 2020;75(24):3085–95. [Google Scholar] [PubMed]
81. Verano-Braga T, Martins ALV, Motta-Santos D, Campagnole-Santos MJ, Santos RAS. ACE2 in the renin-angiotensin system. Clin Sci. 2020;134(23):3063–78. [Google Scholar]
82. Valle Martins AL, da Silva FA, Bolais-Ramos L, de Oliveira GC, Ribeiro RC, Pereira DAA, et al. Increased circulating levels of angiotensin-(1-7) in severely ill COVID-19 patients. ERJ Open Res. 2021;7(3):00114-2021. [Google Scholar] [PubMed]
83. Amezcua-Guerra LM, Del Valle L, Gonzalez-Pacheco H, Springall R, Marquez-Velasco R, Masso F, et al. The prognostic importance of the angiotensin II/angiotensin-(1-7) ratio in patients with SARS-CoV-2 infection. Ther Adv Respir Dis. 2022;16:17534666221122544. [Google Scholar] [PubMed]
84. Carpenter RM, Young MK, Petri WAO, Lyons GR, Gilchrist C, Carey RM, et al. Repressed Ang 1-7 in COVID-19 is inversely associated with inflammation and coagulation. mSphere. 2022;7(4):e0022022. [Google Scholar] [PubMed]
85. Onal H, Ergun NU, Arslan B, Topuz S, Semerci SY, Ugurel OM, et al. Angiotensin (1-7) peptide replacement therapy with plasma transfusion in COVID-19. Transfus Apher Sci. 2022;61(4):103418. [Google Scholar] [PubMed]
86. Seyedmehdi SM, Imanparast F, Mohaghegh P, Mahmoudian S, Dehlaqi MK, Mehvari F, et al. Patients with severe COVID-19 have reduced circulating levels of angiotensin-(1-7a cohort study. Health Sci Rep. 2022;5(2):e564. [Google Scholar] [PubMed]
87. Osman IO, Melenotte C, Brouqui P, Million M, Lagier JC, Parola P, et al. Expression of ACE2, soluble ACE2, angiotensin I, angiotensin II and angiotensin-(1-7) Is modulated in COVID-19 patients. Front Immunol. 2021;12:625732. [Google Scholar] [PubMed]
88. Wang K, Gheblawi M, Nikhanj A, Munan M, MacIntyre E, O’Neil C, et al. Dysregulation of ACE (angiotensin-converting enzyme)-2 and renin-angiotensin peptides in SARS-CoV-2 mediated mortality and end-organ injuries. Hypertension. 2022;79:365–78. [Google Scholar] [PubMed]
89. Wagener G, Goldklang MP, Gerber A, Elisman K, Eiseman KA, Fonseca LD, et al. A randomized, placebo-controlled, double-blinded pilot study of angiotensin 1-7 (TXA-127) for the treatment of severe COVID-19. Crit Care. 2022;26(1):229. [Google Scholar] [PubMed]
90. Hippisley-Cox J, Tan PS, Coupland C. Risk of severe COVID-19 disease with ACE inhibitors and angiotensin receptor blockers: cohort study including 8.3 million people. Heart. 2020;106(19):1503–1511. [Google Scholar] [PubMed]
91. Pan M, Vasbinder A, Anderson E, Catalan T, Shadid HR, Berlin H, et al. Angiotensin-converting enzyme inhibitors, Angiotensin II receptor blockers, and outcomes in patients hospitalized for COVID-19. J Am Heart Assoc. 2021;10(24):e023535. [Google Scholar] [PubMed]
92. Flacco ME, Acuti Martellucci C, Bravi F, Parruti G, Cappadona R, Mascitelli A, et al. Treatment with ACE inhibitors or ARBs and risk of severe/lethal COVID-19: a meta-analysis. Heart. 2020;106:1519–24. [Google Scholar] [PubMed]
93. Patoulias D, Katsimardou A, Stavropoulos K, Imprialos K, Kalogirou MS, Doumas M. Renin-angiotensin system inhibitors and COVID-19: a systematic review and meta-analysis. evidence for significant geographical disparities. Curr Hypertens Rep. 2020;22(11):90. [Google Scholar] [PubMed]
94. Lawler PR, Derde LPG, van de Veerdonk FL, McVerry BJ, Huang DT, et al. Effect of angiotensin-converting enzyme inhibitor and angiotensin receptor blocker initiation on organ support-free days in patients hospitalized with COVID-19: a randomized clinical trial. JAMA. 2023;329(14):1183–96. [Google Scholar] [PubMed]
95. Zolfaghari Emameh R, Falak R, Bahreini E. Application of System biology to explore the association of Neprilysin, Angiotensin-converting enzyme 2 (ACE2and carbonic anhydrase (CA) in pathogenesis of SARS-CoV-2. Biol Proced Online. 2020;22:11. [Google Scholar] [PubMed]
96. Turner AJ, Nalivaeva NN. Angiotensin-converting enzyme 2 (ACE2two decades of revelations and re-evaluation. Peptides. 2022;151:170766. [Google Scholar] [PubMed]
97. Rex DAB, Arun Kumar ST, Modi PK, Keshava Prasad TS. Broadening COVID-19 interventions to drug innovation: neprilysin pathway as a friend, foe, or promising molecular target? OMICS. 2021;25(7):408–16. [Google Scholar] [PubMed]
98. Mann DL, Greene SJ, Givertz MM, Vader JM, Starling RC, Ambrosy AP, et al. Sacubitril/valsartan in advanced heart failure with reduced ejection fraction: rationale and design of the life trial. JACC Heart Fail. 2020;8(10):789–99. [Google Scholar] [PubMed]
99. Nalivaeva NN, Zhuravin IA, Turner AJ. Neprilysin expression and functions in development, ageing and disease. Mech Ageing Dev. 2020;192:111363. [Google Scholar] [PubMed]
100. Acker GR, Molineaux C, Orlowski M. Synaptosomal membrane-bound form of endopeptidase-24.15 generates Leu-enkephalin from dynorphin1-8, α- and β-neoendorphin, and Met-enkephalin from Met-enkephalin-Arg6-Gly7-Leu. J Neurochem. 1987;48(1):284–92. [Google Scholar] [PubMed]
101. Crack PJ, Wu TJ, Cummins PM, Ferro ES, Tullai JW, Glucksman MJ, et al. The association of metalloendopeptidase EC 3.4.24.15 at the extracellular surface of the AtT-20 cell plasma membrane. Brain Res. 1999;835(2):113–24. [Google Scholar] [PubMed]
102. Chappell MC, Allred AJ, Ferrario CM. Pathways of angiotensin-(1-7) metabolism in the kidney. Nephrol Dial Transplant. 2001;16(suppl_1):22–6. [Google Scholar] [PubMed]
103. Ferro ES, Gewehr MCF, Navon A. Thimet oligopeptidase biochemical and biological significances: past, present, and future directions. Biomolecules. 2020;10(9):1229. [Google Scholar] [PubMed]
104. Tragni V, Preziusi F, Laera L, Onofrio A, Mercurio I, Todisco S, et al. Modeling SARS-CoV-2 spike/ACE2 protein-protein interactions for predicting the binding affinity of new spike variants for ACE2, and novel ACE2 structurally related human protein targets, for COVID-19 handling in the 3PM context. EPMA J. 2022;13(1):149–75. [Google Scholar] [PubMed]
105. Ayass MA, Cao W, Zhang J, Dai J, Zhu K, Tripathi T, et al. Noninvasive nasopharyngeal proteomics of COVID-19 patient identify abnormalities related to complement and coagulation cascade and mucosal immune system. PLoS One. 2022;17(9):e0274228. [Google Scholar] [PubMed]
Cite This Article
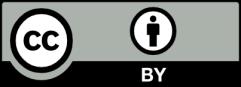