Open Access
REVIEW
A perspective review on the biosynthesis of plant-based secondary metabolites and their application as potent drugs
1 Department of Food Technology, ITM University, Gwalior, India
2 Department of Food Technology, SRM University, Sonipat, India
3 Department of Food Technology, Uttaranchal University, Dehradun, India
4 Department of Nutrition and Dietetics, Manav Rachna International Institute of Research & Studies (MRIIRS), Faridabad, India
5 University Centre for Research and Development, UIBT, Chandigarh University, Mohali, India
6 Amity institute of Biotechnology, Amity University, Jharkhand, Ranchi, India
7 Department of Biotechnology, University Center for Research & Development (UCRD) Chandigarh University, Mohali, Punjab, India
8 Department of Science, Guru Nanak College of Pharmaceutical Sciences, Dehradun, India
9 Program of Biotechnology, Faculty of Science, Assam down town University, Guwahati, India
10 Department of Animal Morphology, Physiology and Genetics, Mendel University in Brno, Brno, Czech Republic
* Corresponding Authors: SUMIRA MALIK. Email: ; PETR SLAMA. Email:
# Represent equal contribution
BIOCELL 2024, 48(4), 541-557. https://doi.org/10.32604/biocell.2023.029031
Received 27 January 2023; Accepted 28 June 2023; Issue published 09 April 2024
Abstract
Many phytochemicals and their derived metabolites produced by plants are extensively employed in commercial goods, pharmaceutical products as well as in the environmental and medical fields. However, these secondary metabolites obtained from plants are in low amounts, and it is difficult to synthesize them at the industrial level. Despite these challenges, they may be utilized for a variety of medicinal products that are either available in the market or are being researched and tested. Secondary metabolites are complex compounds that exhibit chirality. Further, under controlled conditions with elicitors, desired secondary metabolites may be produced from plant cell cultures. This review emphasizes the various aspects of secondary metabolites including their types, synthesis, and applications as medicinal products. The article aims to promote the use of plant secondary metabolites in the management and treatment of various diseases.Graphical Abstract
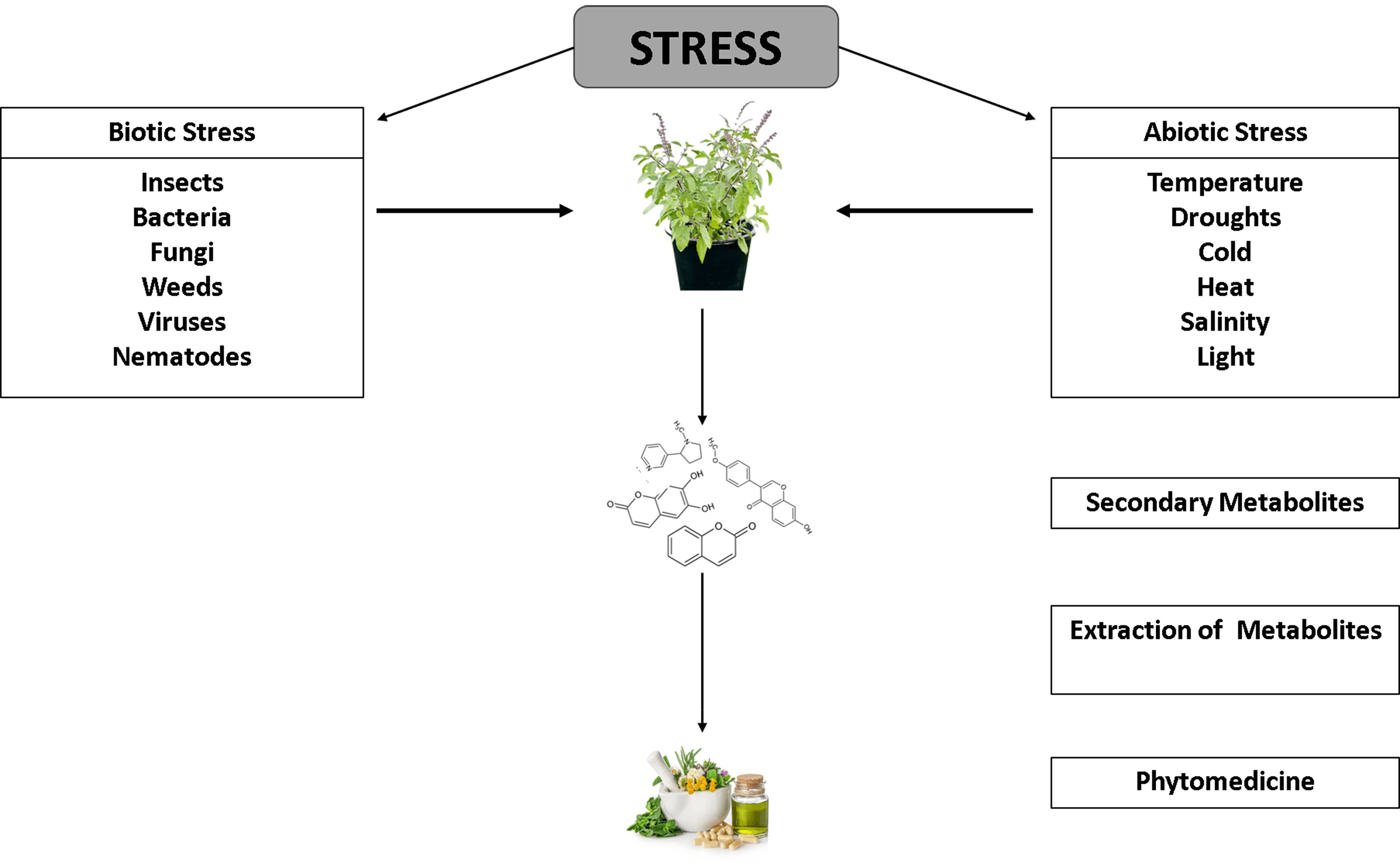
Keywords
Small chemical compounds known as secondary metabolites (SMs), which generally have molecular weights of less than 3000 Da, are produced during the stress developed in plants from primary metabolites [1]. Different plant species have different metabolite types and compositions. While secondary metabolites comprise most of the metabolites in natural plant products, there does not seem a significant difference between primary and secondary metabolites, making their definition difficult to understand. Secondary metabolites are intriguing for various reasons, including their structural variety, potential as therapeutic candidates, and efficacy as antioxidants. Plant metabolites are complexes that the industry cannot create since they have few examples of their chemical variety [1].
Since 2600 BC, plant metabolites have been utilized for various purposes, with most of these applications falling under the categories of food, medicine, and poison. Morphine was the first naturally occurring substance to be extracted from the opium poppy (Papaver somniferum), ushering in a new era in the study of secondary metabolites [2]. It was later determined that the action of plant extracts depends on the single chemical molecule with distinct identity and isolation ability [3]. As a result, these findings served as the foundation for natural product research. Bioactive substances have pharmacological or toxicological impacts on humans and other animals [4]. According to multiple pharmacological research, bioactive substances provide a wide range of health advantages and aid in the prevention of different diseases and metabolic disorders [5]. Plant secondary metabolites play a significant role in pharmaceutical development, as demonstrated by the studies where not less than 30% of drugs are derived directly or indirectly from natural sources [6,7]. The availability of cutting-edge research tools has led to a tremendous expansion in plant metabolite research during the last 100 years. The analytical techniques of mass spectrometry (MS) and nuclear magnetic resonance (NMR), and metabolomics are required for the screening of plant natural products, quality control, and drug discovery in the field of phytomedicine [8]. Due to the typically low quantities of metabolites in plants, their biological activities were mostly unknown earlier, and they were only understood to be metabolic waste or detoxifying products. However, over the last forty years, the knowledge of secondary metabolites has increased. Initially recognized for their harmful effects on animal cells, they are today recognized for their ecological significance and many additional advantages [9]. Various types of extraction strategies are used to extract the bioactive compounds of plants and these strategies depend on different properties such as structure, relative solubility, the nature of the source matrix, and chemical properties (bioactive compound and solvents), temperature, pressure, pH, etc. [10]. In addition to the standard laboratory-level Soxhlet extraction method, maceration, and decoction have both also been extensively employed in the extraction of essential oils and other bioactive substances at a home scale [11]. However, these techniques have certain drawbacks, including prolonged extraction durations and the use of bulk solvents. Further, maceration needs two to seven days for successful extraction, and in the case of decoction, the solvent-to-crude extract ratios might range from four to even fifty to one [12]. The extraction of thermally unstable chemicals is not suited for some techniques that need elevated temperatures. To get over these limitations, many techniques have been devised [13]. The yield and extraction speed have been increased by using aqueous two-phase systems, deep eutectic solvents, and ionic liquids [14]. With the main objective of cell wall rupture or degeneration, aided extraction employing pulsed electric fields, microwaves, pressurized liquids, and ultrasound is the fundamental premise of unconventional extraction methods [15]. This review emphasizes the various aspects of secondary metabolites, such as their types, synthesis, and applications as medicinal products.
Natural chemical compounds produced by plants and utilized for their growth and development include a wide range of substances. Primary metabolites provide the materials for activities like photosynthesis, translocation, and respiration. Secondary metabolites (SMs) are the byproducts of central metabolites that are not directly related to growth and development. They are synthesized by biosynthetic modifications such as methylation, glycosylation, and hydroxylation and are often the byproduct of primary metabolites. Compared to primary metabolites, secondary metabolites are unquestionably more complicated in terms of their side chains and structural makeup. Fig. 1 represents the different types of secondary metabolites.
Figure 1: Types of secondary metabolites.
Plant defense against parasites and pests depends on a class of SMs known as phenolic compounds, which are abundant in plants and foods made from plants [16]. They may be recognized by their structure, which includes at least one phenol ring. They include a variety of structural elements, such as polyphenols, and simple substances like vanillin, gallic acid, and caffeic acid. Depending on their chemical structures, phenolic compounds may be classified into subgroups (Fig. 2) and are often found in soluble or bound forms. Furthermore, bound phenolic substances are produced when soluble phenolic substances are transferred to the cell wall and bind with cell wall components via glycosidic and ester linkages [17]. Typically, soluble phenolic substances are formed in the endoplasmic reticulum (ER) and stored in vacuoles.
Figure 2: Phenolic compounds and their subgroups.
Coumarins (2H-benzopyran-2-one) are simple phenolic substances that are comprised of a substantial phenolic molecule synthesized by the combination of a benzene ring and α-pyrone ring. They are often found in vascular plants. They were found in the tonka bean (Dipteryx odorata) and more than 150 additional species from thirty distinct families [18]. Since then, early thirteen hundred coumarins have been discovered in plants as SMs. They are used by various species for a variety of functions, mostly in plant defense [19]. Coumarins have anti-bacterial activity and protective properties against fungi and herbivores due to a specific bioactive group of chemicals. While all plant parts contain them, the seeds contain the maximum level of these molecules. These can be classified into six groups based on their chemical makeup: simple, dihydrofurano, furano, phenyl, linear-type, and bicoumarins [20].
After cellulose, lignin is the second most prevalent biopolymer, comprising around 30% of the organic carbon in the biosphere. Lignin is one of the cell wall components. It is a high-molecular-weight complex racemic heteropolymer. Lignin is an important SM that stimulates tolerance against various factors, tissue and organ development, plant growth, and the ability to withstand biotic and abiotic stress. Lignification improves the physical hardness of plant tissues, rendering them indigestible to insects and other herbivores while also functioning as a plant defense mechanism against diseases and herbivores [21].
Furanocoumarins are known for their phytotoxicity and are typically found in Apiaceae and Rutaceae species. The phenylpropanoid and mevalonate pathways combine dimethylallyl pyrophosphate and 7-hydroxycoumarin to create furanocoumarins in part. When these materials are exposed to UV-A radiation, which activates a higher electron energy state, they often become toxic. Furanocoumarins penetrate the double helix of DNA, bind to pyrimidines, and induce transcriptional inhibition, resulting in cell death [22]. They also have mutagenic and carcinogenic effects. Furanocoumarins have comparable effects on the physiological growth and food preferences of several insects. They are primarily found in plant roots, leaves, and fruits and are preserved as essential oils. They may dramatically increase the cells sensitivity to ultraviolet, visible light, and solar light [23].
Flavonoids are phytonutrients that are abundantly present in plants. They have low molecular weights and are an important part of the human diet. They belong to one of the most well-known categories of secondary metabolites in higher plants. The chemical structure of flavonoids generally consists of a heterocyclic ring, a phenyl ring, and a 15-carbon skeleton. Flavonoids have a critical role in the pigmentation and defense mechanisms of plants. They also perform several health-promoting tasks and are a vital component of several cosmetic products, medicines, and pharmaceuticals. They may alter crucial cellular enzymatic processes due to their anticarcinogenic, antioxidative, anti-inflammatory, and antimutagenic properties [24].
Isoflavonoids, sometimes known as dietary antioxidants, belong to a group of phenolic compounds that include pterocarpans, isoflavones, and isoflavones. They are made up of a phenyl ring fused with a heterocyclic C-ring and another B-ring fused at the C3 position [25]. Isoflavonoids are usually found in leguminous species. They are naringenin derivatives that are essential for the growth of symbiotic rhizobia and nitrogen-fixing nodules [26]. When plants and microorganisms interact, isoflavonoids may help to produce phytoalexins [27]. It has also been hypothesized that certain isoflavonoids are found in microorganisms.
These are the third most important phenolic group that are common in oligos and polymers and may produce various compounds through various interactions with starch, cellulose, proteins, and minerals. There are two subgroups of tannins: condensed and hydrolyzable tannins, with molecular weights ranging from 600 to 3000. Tannins are often poisonous compounds that may affect the growth and survival of grazing species [22]. Two tannins crucial for plant disease resistance are chlorogenic acid and protocatechuic acid. The quantity of CO2 in the atmosphere, temperature, light, and nutrients are only a few environmental factors that affect the level of tannins that plants produce [28].
Terpenes or terpenoids (Fig. 3) are present in plants that are derived from glycolytic or acetyl-CoA intermediates. They can dissolve in lipids and have an enormous range of structural configurations [29]. Terpenes are the basic building blocks of several complex pigments, sterols, and phytohormones. The physiological functions and fragrances of these compounds are also based on terpenes. While certain terpenes have a pleasant aroma and attract pollinators, the majority of them serve as protective toxins and herbivore deterrents.
Figure 3: Terpenes and their subgroups.
A large class of terpenes known as monoterpenes is linked to the ten-carbon isoprenoids and is utilized extensively in culinary and pharmaceutical products. Natural monoterpenes and their derivatives have powerful anticancer, antiviral, anti-aggregating, antifungal, anti-inflammatory, anti-bacterial, antispasmodic, and local anesthetic effects [30]. Insects are harmed by monoterpene derivatives that collect in the resin ducts of twigs, needles, and trunks. They can also help with growth regulation and thermal tolerance. Monoterpenes give certain plants a distinctive presence in their surroundings. For example, citral gives lemons their unique aroma and thymol adds to the flavor of mandarin oranges. While certain monoterpenes are present in flower smells and may attract pollinators to plants, others are predator deterrents [31].
Sesquiterpenes are a significant additional family of terpenes, which may be found in cyclic and acyclic forms and include three units of isoprene. They occur in oxygenated and hydrocarbon forms, such as alcohols, lactones, aldehydes, and ketones. The plant kingdom has more than 5000 different sesquiterpene compounds, with the Asteraceae family having the greatest quantities of molecules like bisabolene and caryophyllen. Sesquiterpenes differ from costunolides by having a lactone ring with five members [32]. They are renowned for being able to defend themselves against herbivores [33]. Sesquiterpenes may function as transcriptional activators and have a regulatory role in developing and maintaining bud and seed dormancy [34].
Plants, animals, and fungi synthesize diterpenes through the isoprenoid pathway. Diterpenes are composed of two terpene units. They are classified as linear, bicyclic, tricyclic, tetracyclic, pentacyclic, and macrocyclic forms. These compounds are the precursors of various metabolites, including phytol, retinol, and retinal. These metabolites exhibit anti-inflammatory and anti-bacterial properties. Some of them like paclitaxel and ingenol-3-angelate have important biological impacts in a wide range including effective cancer treatments. Other diterpenes including carnosic acid, forskolin, triptolide, salvinorin A, and ginkgolide B exhibit antioxidant, cardioprotective, analgesic, and anti-inflammatory activities [35]. Gibberellins (diterpenes) play a crucial role in the germination of seeds, stomatal conductance, CO2 fixation, and biomass production [22].
Triterpenes are a group of terpenes made up of 6 isoprene units. Twenty thousand different terpenes have been identified so far [36]. Triterpenes contain thirty carbons and may be linear, dicyclic, tricyclic, tetracyclic, or pentacyclic. They are mostly made from squalene [37]. The main constituents of this group exhibit anti-inflammatory, anticancer, antioxidant, anti-bacterial, antiviral, and antifungal properties [38]. They are also found in food, cosmetic, and pharmaceutical products, and they are essential for managing diseases and pests as well as maintaining the quality of the food supplied by agricultural plants [39].
Tetraterpenes contain many isoprene units due to which they have a high molecular weight and belong to polyterpene groups. Multiple repeating isoprene units make up the polyterpene polymer known as rubber. They are found in laticifers, where they aid in wound healing and act as a defense mechanism against herbivores [40]. Like waxes, polyterpenes serve as co-tackifiers and viscosity diluents [41].
Secondary Metabolites Containing Sulfur
The very modest group of secondary metabolites from sulfur-containing plants consists of around 200 compounds. This category consists of the well-known glucosinolates (Fig. 4) and the byproducts of their breakdown, including oxazolidinethiones, epithionitriles, isothiocyanates, and thiocyanates [42]. Sulfur-containing secondary metabolites are essential for protein synthesis; hence, protein synthesis is hampered when sulfur levels are low. Only young leaves of plants are impacted by sulfur deficiency, and its symptoms are similar to those of nitrogen deficiency [43]. Sulfur-containing secondary metabolites are critical influencers of plant health because plants cannot properly use nitrogen without adequate sulfur [44]. According to research, the availability of sulfur and nitrogen affects sulfur- and nitrogen-containing secondary metabolites. Further, the proper balance of both, respectively, increases the plant’s ability to withstand various environmental conditions. Sulfur-containing SMs that are either directly or indirectly related to the plant defense system include defensins, allicin, phytoalexins, thionins, glycosphingolipid, and glutathione [22].
Figure 4: Secondary metabolites containing sulfur.
Secondary Metabolites Containing Nitrogen
The structures of plants often include nitrogen. Glucosinolates, cyanogenic glycosides, alkaloids, and nonprotein amino acids are the four main classes of secondary metabolites that contain nitrogen. These secondary metabolites are formed from common amino acids. The biological functions of SMs in diverse plant species are listed in Table 1.
Biosynthesis of Secondary Metabolites in Plants
Plants synthesize a variety of secondary metabolites. More than 100,000 secondary metabolites are synthesized by plants under unfavorable conditions through several metabolic pathways. The growth environment has a significant impact on both the quality and quantity of these chemicals [77].
Plants employ various metabolic pathways to synthesize a variety of secondary metabolites. For example, mevalonic acid and 2-C-methylerythritol 4-phosphate pathways are two important mechanisms to produce terpenes [78,79]. Malonic acid and shikimic acid pathways are also used by plants to synthesize phenolic chemicals [80]. Upon exposure to salinity and UV-B, plants synthesize the alkaloids via the action of transcription factors such as WRKY6 [81], and various enzymes like hyoscyamine 6-hydroxylase and tryptophan decarboxylase [82]. Fig. 5 represents the general pathway of some secondary metabolites.
Figure 5: Biosynthesis of secondary metabolites.
Precursor and Inducer-Assisted Induction of Plant Secondary Metabolism
There have been attempts to market the production of SMs for human benefit due to their medical and therapeutic properties [83]. Biochemical synthesis of SMs at a large commercial level is difficult due to complex metabolic pathways, the chirality of compounds, and the fact that most plants accumulate SMs in small amounts in specific tissues throughout their life cycles. Given these challenges, numerous methods are being used to increase the synthesis of such metabolites. The expression of secondary metabolites is primarily controlled by the transcriptional activities of gene cassettes encoding specific enzymes in the biosynthetic pathway for the desired products. As a result, the catalytic activities of the genes or enzymes are kept at very low levels, and the active genes are triggered using elicitors. Plant cells may accumulate secondary products due to exposure to elicitors or precursors [84]. The most often utilized and studied elicitors include tryptophan, phenylalanine, methyl jasmonate, jasmonic acid, and its methyl ester, as well as molecular derivatives of arachidonic acid [85]. Elicitors govern the biosynthesis of secondary metabolites as well as increase the synthesis rate through the upregulation of the biochemical pathways. It is important to understand how elicitors are identified by plants and how signals are changed in plant cells to activate and control the production of associated genes that are responsible for the synthesis of secondary metabolites. The secondary metabolite biosynthesis pathways should be investigated to answer these problems.
The remaining alternative, if chemical synthesis cannot be scaled up to make the metabolite, is to augment cell cultures and treat them with elicitors, precursors, and signaling substances to produce the metabolite. For example, when phenylalanine was added to cell suspension cultures of Salvia officinalis and Taxus (driven by the formation of taxol and rosmarinic acid), it acted as a precursor and intermediate to a secondary metabolite via metabolic pathways [86,87]. Elicitors improve the availability of SMs through signal triggering [88].
Enhanced Biomass and Secondary Metabolites in the Culture Environment: Applied Methods for Metabolite Isolation and Structure Elucidation
Obtaining highly concentrated secondary metabolites requires careful attention to physical factors (such as light, temperature, and pH), plant growth regulators, and culture media [89,90]. It is vital to choose source explants as inoculums to produce a high level of SMs. Even though it is widely utilized, there are more effective ways to induce secondary metabolites, given that medium standardization is known to have an impact on the biomass synthesis of secondary metabolites.
Several traditional and modern high-tech methods have been developed to extract metabolites. Conventional methods include the use of water and organic solvents like hexane, acetone, and methanol. The process is conducted at ambient temperature, which significantly permits the dissolution of the soluble metabolites into the solvent throughout the development stages. The effectiveness of this approach depends on the interaction between heat, mixing, and the solvent extraction capacity, which is required to differentiate active components by employing the right solvents. Several cutting-edge extraction techniques, including ultrasound-assisted extraction, supercritical fluid extraction, microwave-assisted extraction, and pressurized liquid extraction have been developed. These methods increase yield while decreasing solvent volume and extraction time [91].
Techniques for Identification and Characterization
A pure molecule is separated from the mixture and identified using chromatographic approaches and non-chromatographic techniques to study vital SMs. The active components in a mixture may be separated from one another or identified using chromatographic procedures. Chromatographic methods are used in various syntheses to separate and purify the products on an industrial scale or for academic reasons. There are many different chromatography methods, from basic thin-layer chromatography to sophisticated gas chromatography and liquid chromatography with mass spectroscopy [92]. Fig. 6 represents the scheme of extraction of bioactive phytochemicals and their applications [93]. Table 2 represents the various methods for the screening of secondary metabolites.
Figure 6: Extraction of bioactive phytochemicals and their applications.
Secondary Metabolites: Their Purpose and Uses
Plant extracts have historically been considered detoxifying or waste products [94]. Most studies were carried out when technology was used to investigate the uses of SMs for the biological tasks for which they were designed in nature. However, the great majority of secondary metabolites still have unclear biological roles. The most significant applications for secondary metabolites are as constituents in medications, food additives, and cosmetics.
Plants as Potential Sources of Medicinal Products
A plant product may be as basic as a skeleton or as complicated as compounds that are not even feasible to create in a lab. These metabolic products exhibit extremely specialized actions through distinct mechanisms. Further, human genome sequencing is used to investigate the potential application of secondary metabolites as therapeutic drugs [95]. Quinine was isolated and discovered, which marked a turning point in the field of pharmacognosy. After this report, numerous active components were isolated from plants and studied [96]. According to research done in a Japanese cohort, eating more fruits and vegetables is associated with a decreased chance of mortality [97]. For the maintenance of balanced gut microbiota, phytochemicals are crucial [98,99]. It has been well-documented how polyphenols like quercetin affect the gut microbiome. These polyphenols are metabolized by gut bacteria, which in turn alters the composition of the microbiome [100]. In a study, when Akkermansia muciniphila and quercetin were administered together, A. muciniphila colonized in the mouse stomach and modified the makeup of the gut microbiota, which decreased obesity and non-alcoholic fatty liver disease by changing bile acid metabolism [101]. Additionally, quercetin prevented fat in mice administered sodium glutamate. Another study demonstrated the interaction between quercetin and inulin, which reduced metabolic syndrome in a high-fat-fed mice model and resulted in significant weight reduction [102]. Administration of quercetin also reduces hyperlipidemia, metabolic syndrome [103], and nonalcoholic steatohepatitis [104].
In 2001, the FDA authorized the use of galantamine, an alkaloid derived from Galanthus woronowii, as a therapy for Alzheimer’s disease [105]. The different methodologies for the screening of secondary metabolites are mentioned in Table 2. Galantamine modifies the nicotinic acetylcholine receptor (nAChR) and inhibits acetylcholinesterase (AChE). Since at least 2000 years ago, artemisinin from Artemisia annua has been used as an antimalarial medication. Sesquiterpene lactone, found in artemisinin, possesses bioactivity that has been shown to cure Plasmodium falciparum-induced malignant cerebral malaria [96]. Due to the low bioavailability of artemisinin, a drug derivative called artemether was subsequently created that had a higher bioavailability [95,113]. Paclitaxel, a highly oxygenated tetracyclic diterpenoid derived from Taxus brevifolia, is an antimitotic drug that prevents tubulin from polymerizing to create microtubules. It was later authorized for many cancer therapies and is also used as a successful medicine against ovarian and breast malignancies [113]. Due to the enormous market demand for paclitaxel and the low natural availability of this drug, many methods have been developed to synthesize it from raw materials such as bacchatin III and 10-deacetylbacchatin III. It was also synthesized in large volumes by the second method utilizing cell cultures of Taxus plants in addition to chemical synthesis. The plant Calophyllum lanigerum was utilized to produce Calanolide A. This dipyranocoumarin inhibits zidovudine (AZT)-resistant strains of HIV and type-1 HIV by acting as a non-nucleoside reverse transcriptase inhibitor [95].
Metabolic Engineering Approaches to Enhance Bioactive Compound Production
There are several metabolic pathways present in plants that are necessary for the synthesis of bioactive compounds. Numerous biotechnological approaches have been used to improve the synthesis of bioactive compounds including lipid based vascular drug delivery systems [114,115]. Given such findings, plant-based natural product derivatives are often used as medicinal treatments (Table 3). Gene editing methods are the latest development to enhance the production of bioactive compounds [119]. To fulfill the demand for medicinal plants, tissue culture techniques offer additional benefits over traditional methods of propagation [120]. Through the cell culture of plants, valuable metabolites can be produced [121]. The cell culture approach is used for the culturing of plant cells at a large scale for the mass production of bioactive compounds. For example, the mass production of geraniol was accomplished by the utilization of cell culture [122]. Cell culture has shown to be a manageable, economically feasible technology for the synthesis of natural products [123]. This method is independent of environmental or climatic factors and enables cells to proliferate at faster growth rates in a closed and controlled environment [124]. The most important stage in the commercialization of natural products is the expansion of the cell culture from a laboratory to an industrial scale. Therefore, it is crucial to choose a bioreactor that can meet the culture’s technical requirements as well as its biological requirements. The optimization of fundamental culture characteristics, such as nutrition availability, low-shear environment, proper mixing, and lowering mass transfer for enhanced oxygen, is necessary for the scaling-up phase from shaking flask cultures to bioreactors [125]. Air-life bioreactors (ALB), bubble column bioreactors (BCB), standard stirred-tank bioreactors (STR), hollow fiber bioreactors, wave bioreactors, membrane bioreactors, and rotating drum reactors can be used to cultivate plant cells [126]. Fig. 7 represents the flowchart for the process of metabolic engineering to enhance the production of bioactive compounds.
Figure 7: Flowchart for the process of metabolic engineering to enhance the production of bioactive compounds.
Nanotechnology-Based Drug Delivery Systems for Phytochemical Compounds
The literature states that 70% of the active components derived from plants are hydrophobic [127]. The bioavailability and bioactivity of phytochemical substances have been increased by the application of new technologies. For example, lipid-based and polymer-based delivery mechanisms boost the bioactivity of phytochemical substances [128,129]. The various nanocarriers for the delivery of phytochemicals were explained by the (Lipid-based carrier systems, which include vesicular systems, lipid particulates, and nanoemulsions have attracted interest in increasing the bioactivity, bioavailability, and stability of phytochemical compounds [130,127]. Vesicular drug delivery methods are characterized as highly organized assemblies made up of single or multiple concentric bilayers that self-assemble in the presence of water. The lipid cores of solid lipid nanoparticles (SLN) and nanostructured lipid carriers (NLC), two different types of nanoparticle systems, are made of solid lipids or mixes of liquid lipids [15]. The bioavailability of hydrophobic compounds was shown to be increased using nanoemulsions [131]. The most investigated nanocarriers are lipid-based nanosystems. When compared to polymer based-carriers, these carriers often have a reduced toxicity profile and a more affordable price [132]. The delivery of liposomes and phytosomes is regarded as one of the most successful strategies [133]. Liposomes provide the potential to enhance the solubility, effectiveness, and bioavailability of medications due to their excellent biocompatibility and biodegradability. Bioactive compounds that are both hydrophilic and lipophilic can be enclosed in liposomes [134,135].
Conclusion and Future Prospects
A remarkable library of bioactive molecules with a wide range of activities in the context of parasites, fungi, bacteria, and human cells can be found in plants. These secondary metabolites are sometimes lethal at high concentrations and very specialized, making them very crucial. Intense efforts have been made to investigate secondary metabolites for economic reasons, which have helped establish various branches of photochemistry. The primary objective of this review article was to outline the various secondary metabolite-related aspects, manufacturing syntheses, and plant-based sources for such medicinal metabolites. Therefore, this article will serve as a comprehensive resource for readers or researchers who are interested in secondary metabolites.
It is crucial that the Food and Drug Administration (FDA), World Health Organisation (WHO), European Medicines Agency (EMA), the pharmaceutical industry, biotech firms, and numerous other regulatory bodies worldwide coordinate and work together in order to provide clear guidelines for the research and development of herbal drugs and to make the most of the enormous potential of traditional medicines for the synthesis of drugs for various diseases. Only a portion of the medicinal plants and their phytochemical qualities have been studied, despite the fact that they serve as potential sources for new drug discoveries. With the use of databases and multidisciplinary team activities, the complete molecular characterization of the therapeutic substances must be explored and evaluated. The development of drugs that are effective and less toxic will ultimately help the entire global population.
Acknowledgement: The authors gratefully acknowledge Mrs. Tanbeer Kaur, Assistant Professor, Shoolini University, Solan, for the comments that have helped to greatly improve the manuscript.
Funding Statement: No funding is applied.
Author Contributions: Study conception and design: Jhilam Pramanik and Akash Kumar; data collection: Sumira Malik, Sarvesh Rustagi and Jutishna Bora; analysis and interpretation of results: Jhilam Pramanik, Akash Kumar, Sumira Malik, Anchal Trehan, Mehak Katyal, Nayan Talukdar, Sheetal Thakur; draft manuscript preparation: Sumira Malik and Petr Slama. All authors reviewed the results and approved the final version of the manuscript.
Availability of Data and Materials: Data sharing not applicable to this article as no datasets were generated or analyzed during the current study.
Ethics Approval: Not applicable.
Conflicts of Interest: The authors declare that they have no conflicts of interest to report regarding the present study.
References
1. Bourgaud F, Gravot A, Milesi S, Gontier E. Production of plant secondary metabolites: a historical perspective. Plant Sci. 2001;161:839–51. doi:10.1016/S0168-9452(01)00490-3. [Google Scholar] [CrossRef]
2. Sanchez S, Demain AL. Secondary metabolites. Compr Biotechnol. 2011;84(23):131–43. doi:10.1016/B978-0-444-64046-8.00012-4. [Google Scholar] [CrossRef]
3. Tsao R, Yang R, Xie S, Sockovie E, Khanizadeh S. Which polyphenolic compounds contribute to the total antioxidant activities of apple? J Agr Food Chem. 2005;53(12):4989–95. doi:10.1021/jf048289h. [Google Scholar] [PubMed] [CrossRef]
4. Câmara JS, Albuquerque BR, Aguiar J, Corréa RCG, Gonçalves JL, Granato D, et al. Food bioactive compounds and emerging techniques for their extraction: polyphenols as a case study. Foods. 2020;10(1):37. doi:10.3390/foods10010037. [Google Scholar] [PubMed] [CrossRef]
5. Chhikara N, Kushwaha K, Sharma P, Gat Y, Panghal A. Bioactive compounds of beetroot and utilization in food processing industry: a critical review. Food Chem. 2019;272(1):192–200. doi:10.1016/j.foodchem.2018.08.022. [Google Scholar] [PubMed] [CrossRef]
6. Newman DJ, Cragg GM. Marine natural products and related compounds in clinical and advanced preclinical trials. J Nat Prod. 2004;67(8):1216–38. doi:10.1021/NP040031Y. [Google Scholar] [PubMed] [CrossRef]
7. Cragg GM, Newman DJ. Natural products: a continuing source of novel drug leads. Biochim Biophys Acta. 2013;1830(6):3670–95. doi:10.1016/j.bbagen.2013.02.008. [Google Scholar] [PubMed] [CrossRef]
8. Salem MA, Perez de Souza L, Serag A, Fernie AR, Farag MA, Ezzat SM, et al. Metabolomics in the context of plant natural products research: from sample preparation to metabolite analysis. Metab. 2020;10:37. doi:10.3390/metabo10010037. [Google Scholar] [PubMed] [CrossRef]
9. Weinberg ED. Trace-metal control of specific biosynthetic processes. Perspect Biol Med. 1962;5(4):432–45. doi:10.1353/pbm.1962.0017. [Google Scholar] [PubMed] [CrossRef]
10. Azmir J, Zaidul ISM, Rahman MM, Sharif KM, Mohamed A, Sahena F, et al. Techniques for extraction of bioactive compounds from plant materials: a review. J Food Eng. 2013;117(4):426–36. doi:10.1016/j.jfoodeng.2013.01.014. [Google Scholar] [CrossRef]
11. Muala WCB, Desobgo ZSC, Jong NE. Optimization of extraction conditions of phenolic compounds from Cymbopogon citratus and evaluation of phenolics and aroma profiles of extract. Heliyon. 2021;7(4):e06744. doi:10.1016/j.heliyon.2021.e06744. [Google Scholar] [PubMed] [CrossRef]
12. Li J, Niu D, Zhang Y, Zeng XA. Physicochemical properties, antioxidant and antiproliferative activities of polysaccharides from Morinda citrifolia L. (Noni) based on different extraction methods. Int J Biol Macromol. 2020;150:114–21. doi:10.1016/j.ijbiomac.2019.12.157. [Google Scholar] [PubMed] [CrossRef]
13. Pal CBT, Jadeja GC. Microwave-assisted extraction for recovery of polyphenolic antioxidants from ripe mango (Mangifera indica L.) peel using lactic acid/sodium acetate deep eutectic mixtures. Food Sci Technol Int. 2020;26(1):78–92. doi:10.1177/1082013219870010. [Google Scholar] [PubMed] [CrossRef]
14. Priyadarshi S, Balaraman M, Naidu MM. Ionic liquid-based microwave-assisted extraction of Heneicos-1-ene from coriander foliage and optimizing yield parameters by response surface methodology. Prep Biochem Biotechnol. 2019;50:246–51. doi:10.1080/10826068.2019.1687519. [Google Scholar] [PubMed] [CrossRef]
15. Lefebvre T, Destandau E, Lesellier E. Selective extraction of bioactive compounds from plants using recent extraction techniques: a review. J Chromatogr A. 2021;1635:461770. doi:10.1016/j.chroma.2020.461770. [Google Scholar] [PubMed] [CrossRef]
16. Wuyts N, de Waele D, Swennen R. Extraction and partial characterization of polyphenol oxidase from banana (Musa acuminata Grande naine) roots. Plant Physiol Biochem. 2006;44:308–14. doi:10.1016/j.plaphy.2006.06.005. [Google Scholar] [PubMed] [CrossRef]
17. Gan RY, Chan CL, Yang QQ, Bin Li H, Zhang D, Ge YY, et al. Bioactive compounds and beneficial functions of sprouted grains. Sprouted Grains. 2019;54(3):191–246. doi:10.1016/B978-0-12-811525-1.00009-9. [Google Scholar] [CrossRef]
18. Venugopala KN, Rashmi V, Odhav B. Review on natural coumarin lead compounds for their pharmacological activity. Biomed Res Int. 2013;2013(6):1–14. doi:10.1155/2013/963248. [Google Scholar] [PubMed] [CrossRef]
19. Iranshahi M, Askari M, Sahebkar A, Hadjipavlou-Litina D. Evaluation of antioxidant, anti-inflammatory and lipoxygenase inhibitory activities of the prenylated coumarin umbelliprenin. DARU J Pharm Sci. 2009;17:99–103. [Google Scholar]
20. Evans WC. Trease and Evans’ pharmacognosy. 16th ed. London: Elsevier Health Sciences; 2009. p. 1–603. [Google Scholar]
21. Cesarino I, Araújo P, Domingues AP, Mazzafera P. An overview of lignin metabolism and its effect on biomass recalcitrance. Braz J Bot. 2012;35(4):303–11. doi:10.1590/S0100-84042012000400003. [Google Scholar] [CrossRef]
22. Mazid M, Khan TA, Mohammad F. Role of secondary metabolites in defense mechanisms of plants. Biol Med. 2011;3:232–49. [Google Scholar]
23. Del Río JA, Díaz L, García-Bernal D, Blanquer M, Ortuño A, Correal E, et al. Furanocoumarins: biomolecules of therapeutic interest. Stud Nat Prod Chem. 2014;43:145–95. doi:10.1016/B978-0-444-63430-6.00005-9. [Google Scholar] [CrossRef]
24. Walker EH, Pacold ME, Perisic O, Stephens L, Hawkins PT, Wymann MP, et al. Structural determinants of phosphoinositide 3-kinase inhibition by wortmannin, LY294002, quercetin, myricetin, and staurosporine. Mol Cell. 2000;6:909–19. doi:10.1016/S1097-2765(05)00089-4. [Google Scholar] [PubMed] [CrossRef]
25. Han RM, Tian YX, Liu Y, Chen CH, Ai XC, Zhang JP, et al. Comparison of flavonoids and isoflavonoids as antioxidants. J Agric Food Chem. 2009;57(9):3780–5. doi:10.1021/JF803850P. [Google Scholar] [PubMed] [CrossRef]
26. Sreevidya VS, Srinivasa Rao C, Sullia SB, Ladha JK, Reddy PM. Metabolic engineering of rice with soybean isoflavone synthase for promoting nodulation gene expression in rhizobia. J Exp Bot. 2006;57(9):1957–69. doi:10.1093/jxb/erj143. [Google Scholar] [PubMed] [CrossRef]
27. Dixon RA, Ferreira D. Genistein. Phytochem. 2002;60(3):205–11. doi:10.1016/S0031-9422(02)00116-4. [Google Scholar] [PubMed] [CrossRef]
28. Fine PVA, Miller ZJ, Mesones I, Irazuzta S, Appel HM, Stevens MHH, et al. The growth-defense trade-off and habitat specialization by plants in amazonian forests. Ecol. 2006;87:150–62. doi:10.1890/0012-9658(2006)87. [Google Scholar] [CrossRef]
29. Vranová E, Coman D, Gruissem W. Structure and dynamics of the isoprenoid pathway network. Mol Plant. 2012;5(2):318–33. doi:10.1093/mp/sss015. [Google Scholar] [PubMed] [CrossRef]
30. Moniczewski A, Librowski T, Lochyński S, Strub D. Evaluation of the irritating influence of carane derivatives and their antioxidant properties in a deoxyribose degradation test. Pharmacol Rep. 2011;63(1):120–9. doi:10.1016/S1734-1140(11)70406-6. [Google Scholar] [PubMed] [CrossRef]
31. Boncan DAT, Tsang SSK, Li C, Lee IHT, Lam HM, Chan TF, et al. Terpenes and terpenoids in plants: interactions with environment and insects. Int J Mol Sci. 2020;21:7382. doi:10.3390/ijms21197382. [Google Scholar] [PubMed] [CrossRef]
32. Canales M, Hernández T, Caballero J, Romo de Vivar A, Avila G, Duran A, et al. Informant consensus factor and antibacterial activity of the medicinal plants used by the people of San Rafael Coxcatlán, Puebla, México. J Ethnopharmacol. 2005;97:429–39. doi:10.1016/j.jep.2004.11.013. [Google Scholar] [PubMed] [CrossRef]
33. Kessler A, Baldwin IT. Defensive function of herbivore- induced plant volatile emissions in nature. Sci. 2001;291(5511):2141–4. doi:10.1126/science.291.5511.2141. [Google Scholar] [PubMed] [CrossRef]
34. Schnall JA, Quatrano RS. Abscisic acid elicits the water-stress response in root hairs of Arabidopsis thaliana. Plant Physiol. 1992;100(1):216–8. doi:10.1104/pp.100.1.216. [Google Scholar] [PubMed] [CrossRef]
35. Mafu S, Zerbe P. Plant diterpenoid metabolism for manufacturing the biopharmaceuticals of tomorrow: prospects and challenges. Phytochem Rev. 2017;1(1):113–30. doi:10.1007/S11101-017-9513-5. [Google Scholar] [CrossRef]
36. Hill RA, Connolly JD. Triterpenoids. Nat Prod Rep. 2017;34(1):90–122. doi:10.1039/C6NP00094K. [Google Scholar] [PubMed] [CrossRef]
37. Breitmaier E. Terpenes: flavors, fragrances, pharmaca, pheromones. Wiley-VCH; 2006. doi:10.1002/aoc.1209. [Google Scholar] [CrossRef]
38. Chudzik M, Korzonek-Szlacheta I, Król W. Triterpenes as potentially cytotoxic compounds. Mol. 2015;20(1):1610–25. doi:10.3390/molecules20011610. [Google Scholar] [PubMed] [CrossRef]
39. Thimmappa R, Geisler K, Louveau T, O’Maille P, Osbourn A. Triterpene biosynthesis in plants. Annu Rev Plant Biol. 2014;65(1):225–57. doi:10.1146/annurev-arplant-050312-120229. [Google Scholar] [PubMed] [CrossRef]
40. Eisner T, Meinwald J. Chemical ecology. Proc Natl Acad Sci USA. 1995;92(1):1. doi:10.1073/pnas.92.1.1. [Google Scholar] [PubMed] [CrossRef]
41. Benedek I. Pressure-sensitive adhesives and applications. Boca Raton: CRC Press; 2004, doi:10.1201/9780203021163. [Google Scholar] [CrossRef]
42. Venditti A, Bianco A. Sulfur-containing secondary metabolites as neuroprotective agents. Curr Med Chem. 2018;27(26):4421–36. doi:10.2174/0929867325666180912105036. [Google Scholar] [PubMed] [CrossRef]
43. Harz G. Sulphur deficiency symptoms in oilseed rape (Brassica napus L.)–The aesthetics of starvation. Phyton-Horn. 2010;45:79–95. [Google Scholar]
44. Bloem E, Haneklaus S, Salac I, Wickenhäuser P, Schnug E. Facts and fiction about sulfur metabolism in relation to plant-pathogen interactions. Plant Biol. 2007;9(5):596–607. doi:10.1055/s-2007-965420. [Google Scholar] [PubMed] [CrossRef]
45. Griffith M, Yaish MWF. Antifreeze proteins in overwintering plants: a tale of two activities. Trends Plant Sci. 2004;9(8):399–405. doi:10.1016/j.tplants.2004.06.007. [Google Scholar] [PubMed] [CrossRef]
46. Jain A, Singh A, Singh S, Singh HB. Phenols enhancement effect of microbial consortium in pea plants restrains Sclerotinia sclerotiorum. Biol Control. 2015;89(3):23–32. doi:10.1016/j.biocontrol.2015.04.013. [Google Scholar] [CrossRef]
47. Aldulaimi O. Screening of fruits of seven plants indicated for medicinal use in Iraq. Pharmacogn Mag. 2017;13(50):S189–95. doi:10.4103/0973-1296.210191. [Google Scholar] [CrossRef]
48. Lopes Andrade AW, Dias Ribeiro Figueiredo D, Torequl Islam M, Viana Nunes AM, da Conceição Machado K, da Conceição Machado K, et al. Toxicological evaluation of the biflavonoid, agathisflavone in albino Swiss mice. Biomed Pharmacother. 2019;110:68–73. doi:10.1016/j.biopha.2018.11.050. [Google Scholar] [PubMed] [CrossRef]
49. Hou DX, Fujii M, Terahara N, Yoshimoto M. Molecular mechanisms behind the chemopreventive effects of anthocyanidins. J Biomed Biotechnol. 2004;5(5):321–5. doi:10.1155/S1110724304403040. [Google Scholar] [PubMed] [CrossRef]
50. Brown GD. The biosynthesis of artemisinin (Qinghaosu) and the phytochemistry of Artemisia annua L (Qinghao). Mol. 2010;15:7603–98. doi:10.3390/molecules15117603. [Google Scholar] [PubMed] [CrossRef]
51. Abe I. Enzymatic synthesis of cyclic triterpenes. Nat Prod Rep. 2007;24:1311–31. doi:10.1039/b616857b. [Google Scholar] [PubMed] [CrossRef]
52. Abdalla MA, Mühling KH. Plant-derived sulfur containing natural products produced as a response to biotic and abiotic stresses: a review of their structural diversity and medicinal importance. J Appl Bot Food Qual. 2019;92:204–15. doi:10.5073/JABFQ.2019. [Google Scholar] [CrossRef]
53. Martínez‐Ballesta MDC, Muries B, Moreno DÁ, Dominguez‐Perles R, García‐Viguera C, Carvajal M. Involvement of a glucosinolate (sinigrin) in the regulation of water transport in Brassica oleracea grown under salt stress. Physiol Plant. 2014;150(2):145–60. doi:10.1111/PPL.12082. [Google Scholar] [PubMed] [CrossRef]
54. Li X, Lv X, Wang X, Wang L, Zhang M, Ren M. Effects of abiotic stress on anthocyanin accumulation and grain weight in purple wheat. CSIRO Publish. 2018;69(12):1208–14. doi:10.1071/CP18341. [Google Scholar] [CrossRef]
55. Moulin M, Deleu C, Larher F, Bouchereau A. The lysine- ketoglutarate reductase-saccharopine dehydrogenase is involved in the osmo-induced synthesis of pipecolic acid in rapeseed leaf tissues. Plant Physiol Biochem. 2006;44(7–9):474–82. doi:10.1016/j.plaphy.2006.08.005. [Google Scholar] [PubMed] [CrossRef]
56. Binder BYK, Peebles CAM, Shanks J, San KY. The effects of UV-B stress on the production of terpenoid indole alkaloids in Catharanthus roseus hairy roots. Biotechnol Prog. 2009;25(3):861–5. doi:10.1002/btpr.97. [Google Scholar] [PubMed] [CrossRef]
57. Ahmed E, Arshad M, Zakriyya Khan M, Shoaib Amjad M, Mehreen Sadaf H, et al. Secondary metabolites and their multidimensional prospective in plant life. J Pharmacogn Phytochem. 2017;6:205–14. [Google Scholar]
58. Yokota A, Kawasaki S, Iwano M, Nakamura C, Miyake C, Akashi K. Citrulline and DRIP-1 protein (ArgE homologue) in drought tolerance of wild watermelon. Ann Bot. 2002;89(7):825–32. doi:10.1093/aob/mcf074. [Google Scholar] [PubMed] [CrossRef]
59. Pang XY, Wang S, Jurczak MJ, Shulman GI, Moise AR. Retinol saturase modulates lipid metabolism and the production of reactive oxygen species. Arch Biochem Biophys. 2017;633:93–102. doi:10.1016/j.abb.2017.09.009. [Google Scholar] [PubMed] [CrossRef]
60. Masondo NA, Aremu AO, Finnie JF, van Staden J. Plant growth regulator induced phytochemical and antioxidant variations in micropropagated and acclimatized Eucomis autumnalis subspecies autumnalis (Asparagaceae). Acta Physiol Plant. 2014;36(9):2467–79. doi:10.1007/s11738-014-1619-4. [Google Scholar] [CrossRef]
61. Jung HY, Kang SM, Kang YM, Kang MJ, Yun DJ, Bahk JD, et al. Enhanced production of scopolamine by bacterial elicitors in adventitious hairy root cultures of Scopolia parviflora. Enzyme Microb Technol. 2003;33(7):987–90. doi:10.1016/S0141-0229(03)00253-9. [Google Scholar] [CrossRef]
62. Chi WC, Fu SF, Huang TL, Chen YA, Chen CC, Huang HJ. Identification of transcriptome profiles and signaling pathways for the allelochemical juglone in rice roots. Plant Mol Biol. 2011;77(6):591–607. doi:10.1007/s11103-011-9841-6. [Google Scholar] [PubMed] [CrossRef]
63. Lai D, Pičmanová M, Abou Hachem M, Motawia MS, Olsen CE, Møller BL, et al. Lotus japonicus flowers are defended by a cyanogenic β-glucosidase with highly restricted expression to essential reproductive organs. Plant Mol Biol. 2015;89(1–2):21–34. doi:10.1007/s11103-015-0348-4. [Google Scholar] [PubMed] [CrossRef]
64. Luo F, Lv Q, Zhao Y, Hu G, Huang G, Zhang J, et al. Quantification and purification of mangiferin from Chinese mango (Mangifera indica L.) cultivars and its protective effect on human umbilical vein endothelial cells under H2O2-induced stress. Int J Mol Sci. 2012;13:11260–74. doi:10.3390/ijms130911260. [Google Scholar] [PubMed] [CrossRef]
65. Lin Y, Qasim M, Hussain M, Akutse KS, Avery PB, Dash CK, et al. The herbivore-induced plant volatiles methyl salicylate and menthol positively affect growth and pathogenicity of entomopathogenic fungi. Sci Rep. 2017;7(1):40494. doi:10.1038/srep40494. [Google Scholar] [PubMed] [CrossRef]
66. Llorens-Molina JA, Vacas S. Effect of drought stress on essential oil composition of Thymus vulgaris L. (Chemotype 1, 8-cineole) from wild populations of Eastern Iberian Peninsula. J Essent Oil Res. 2017;29(2):145–55. doi:10.1080/10412905.2016.1211561. [Google Scholar] [CrossRef]
67. Petridis A, Therios I, Samouris G, Tananaki C. Salinity-induced changes in phenolic compounds in leaves and roots of four olive cultivars (Olea europaea L.) and their relationship to antioxidant activity. Environ Exp Bot. 2012;79:37–43. doi:10.1016/j.envexpbot.2012.01.007. [Google Scholar] [CrossRef]
68. Stringlis IA, de Jonge R, Pieterse CMJ. The age of coumarins in plant-microbe interactions. Plant Cell Physiol. 2019;60(7):1405–19. doi:10.1093/pcp/pcz076. [Google Scholar] [PubMed] [CrossRef]
69. Mishra RP, Singh RK, Jaiswal HK, Kumar V, Maurya S. Rhizobium-mediated induction of phenolics and plant growth promotion in rice (Oryza sativa L.). Curr Microbiol. 2006;52(5):383–9. doi:10.1007/s00284-005-0296-3. [Google Scholar] [PubMed] [CrossRef]
70. Fournier AR, Proctor JTA, Gauthier L, Khanizadeh S, Bélanger A, Gosselin A, et al. Understory light and root ginsenosides in forest-grown Panax quinquefolius. Phytochem. 2003;63:777–82. doi:10.1016/S0031-9422(03)00346-7. [Google Scholar] [PubMed] [CrossRef]
71. Hartmann T, Ober D. Biosynthesis and metabolism of pyrrolizidine alkaloids in plants and specialized insect herbivores. In: Topics in current chemistry. Berlin: Springer; 2000. p. 207–43. doi:10.1007/3-540-48146-X_5. [Google Scholar] [CrossRef]
72. Gan RY, Kong KW, bin Li H, Wu K, Ge YY, Chan CL, et al. Separation, identification, and bioactivities of the main gallotannins of red sword bean (Canavalia gladiata) coats. Front Chem. 2018;6:39. doi:10.3389/FCHEM.2018.00039/FULL. [Google Scholar] [CrossRef]
73. Shi M, Kwok KW, Wu JY. Enhancement of tanshinone production in Salvia miltiorrhiza Bunge (red or Chinese sage) hairy-root culture by hyperosmotic stress and yeast elicitor. Biotechnol Appl Biochem. 2007;46(4):191–6. doi:10.1042/BA20060147. [Google Scholar] [PubMed] [CrossRef]
74. Munné-Bosch S, Mueller M, Schwarz K, Alegre L. Diterpenes and antioxidative protection in drought-stressed Salvia officinalis plants. J Plant Physiol. 2001;158(11):1431–7. doi:10.1078/0176-1617-00578. [Google Scholar] [CrossRef]
75. Hodaei M, Rahimmalek M, Arzani A, Talebi M. The effect of water stress on phytochemical accumulation, bioactive compounds and expression of key genes involved in flavonoid biosynthesis in Chrysanthemum morifolium L. Ind Crop Prod. 2018;120:295–304. doi:10.1016/J.INDCROP.2018.04.073. [Google Scholar] [CrossRef]
76. Pedras MS, Zheng QA, Schatte G, Adio AM. Photochemical dimerization of wasalexins in UV-irradiated Thellungiella halophila and in vitro generates unique cruciferous phytoalexins. Phytochem. 2009;70:2010–6. doi:10.1016/j.phytochem.2009.09.008. [Google Scholar] [PubMed] [CrossRef]
77. Meena KK, Sorty AM, Bitla UM, Choudhary K, Gupta P, Pareek A, et al. Abiotic stress responses and microbe-mediated mitigation in plants: the omics strategies. Front Plant Sci. 2017;8(868):172. doi:10.3389/fpls.2017.00172. [Google Scholar] [PubMed] [CrossRef]
78. Simpson K, Fuentes P, Quiroz-Iturra LF, Flores-Ortiz C, Contreras R, Handford M, et al. Unraveling the induction of phytoene synthase 2 expression by salt stress and abscisic acid in Daucus carota. J Exp Bot. 2018;69(16):4113–26. doi:10.1093/JXB/ERY207. [Google Scholar] [PubMed] [CrossRef]
79. Sankari M, Hridya H, Sneha P, Doss CG, Christopher JG, Mathew J, et al. Implication of salt stress induces changes in pigment production, antioxidant enzyme activity, and qRT-PCR expression of genes involved in the biosynthetic pathway of Bixa orellana L. Funct Integr Genom. 2019;19:565–74. doi:10.1007/s10142-019-00654-7. [Google Scholar] [PubMed] [CrossRef]
80. Ghasemzadeh A, Ghasemzadeh N. Flavonoids and phenolic acids: role and biochemical activity in plants and human. J Med Plants Res. 2011;5(31):6697–703. doi:10.5897/JMPR11.1404. [Google Scholar] [CrossRef]
81. Chen YF, Li LQ, Xu Q, Kong YH, Wang H, Wu WH. The WRKY6 transcription factor modulates PHOSPHATE1 expression in response to low Pi stress in Arabidopsis. Plant Cell. 2009;21(11):3554–66. doi:10.1105/tpc.108.064980. [Google Scholar] [PubMed] [CrossRef]
82. Mehrotra S, Mishra S, Srivastava V. Hairy root cultures for monoterpene indole alkaloid pathway: investigation and biotechnological production. In: Hairy roots: an effective tool of plant biotechnology. Singapore: Springer; 2018. p. 95–121. doi:10.1007/978-981-13-2562-5_5. [Google Scholar] [CrossRef]
83. Lewis WH, Elvin-Lewis MPF. Medical botany, plants affecting man’s health. London: Wiley; 1977. doi:10.3/JQUERY-UI.JS. [Google Scholar] [CrossRef]
84. Twaij BM, Hasan MN. Bioactive secondary metabolites from plant sources: types, synthesis, and their therapeutic uses. Int J Plant Biol. 2022;13:4–14. doi:10.3390/ijpb13010003. [Google Scholar] [CrossRef]
85. Gundlach H, Müller MJ, Kutchan TM, Zenk MH. Jasmonic acid is a signal transducer in elicitor-induced plant cell cultures. Proc Natl Acad Sci USA. 1992;89(6):2389–93. doi:10.1073/pnas.89.6.2389. [Google Scholar] [PubMed] [CrossRef]
86. Fett-Neto AG, Melanson SJ, Sakata K, DiCosmo F. Improved growth and taxol yield in developing calli of Taxus cuspidata by medium composition modification. Bio/Technol. 1993;11(6):731–4. doi:10.1038/nbt0693-731. [Google Scholar] [PubMed] [CrossRef]
87. Mulabagal V, Tsay HS. Plant cell cultures—An alternative and efficient source for the production of biologically important secondary metabolites. Int J Appl Sci Eng. 2004;2:29–48. doi:10.6703/IJASE.2004.2(1).29. [Google Scholar] [CrossRef]
88. Radman R, Saez T, Bucke C, Keshavarz T. Elicitation of plants and microbial cell systems. Biotechnol Appl Biochem. 2003;37(1):91–102. doi:10.1042/BA20020118. [Google Scholar] [PubMed] [CrossRef]
89. Dörnenburg H, Frickinger P, Seydel P. Plant cell-based processes for cyclotides production. J Biotechnol. 2008;135(1):123–6. doi:10.1016/j.jbiotec.2008.03.005. [Google Scholar] [PubMed] [CrossRef]
90. Res SK. A review on trends in production of secondary metabolites from higher plants by in vitro tissue, organ and cell cultures. J Med Plants Res. 2009;3:1222–39. [Google Scholar]
91. Veer V, Gopalakrishnan R. Herbal insecticides, repellents and biomedicines: effectiveness and commercialization. New Delhi: Springer; 2016. p. 1–258. doi:10.1007/978-81-322-2704-5. [Google Scholar] [CrossRef]
92. Sasidharan S, Chen Y, Saravanan D, Sundram KM, Yoga Latha L. Extraction, isolation and characterization of bioactive compounds from plants’ extracts. Afr J Tradit Complement Altern Med. 2010;8(1):1–10. doi:10.4314/ajtcam.v8i1.60483. [Google Scholar] [CrossRef]
93. Adeola HA, Bano A, Vats R, Vashishtha A, Verma D, Kaushik D, et al. Bioactive compounds and their libraries: an insight into prospective phytotherapeutics approach for oral mucocutaneous cancers. Biomed Pharmacother. 2021;141:111809. doi:10.1016/j.biopha.2021.111809. [Google Scholar] [PubMed] [CrossRef]
94. Jarvis MF, Williams M. Direct autoradiographic localization of adenosine A2 receptors in the rat brain using the A2-selective agonist, [3H]CGS 21680. Eur J Pharmacol. 1989;168:243–6. doi:10.1016/0014-2999(89)90571-2. [Google Scholar] [PubMed] [CrossRef]
95. Watson DJ, Laing L, Gibhard L, Wong HN, Haynes RK, Wiesner L. Toward new transmission-blocking combination therapies: pharmacokinetics of 10-amino-artemisinins and 11-aza-artemisinin and comparison with dihydroartemisinin and artemether. Antimicrob Agents Chemother. 2021;65(8):e0099021. doi:10.1128/AAC.00990-21. [Google Scholar] [PubMed] [CrossRef]
96. Phillipson JD. Phytochemistry and medicinal plants. Phytochem. 2001;56:237–43. doi:10.1016/S0031-9422(00)00456-8. [Google Scholar] [PubMed] [CrossRef]
97. Sahashi Y, Goto A, Takachi R, Ishihara J, Kito K, Kanehara R, et al. Inverse association between fruit and vegetable intake and all-cause mortality: Japan public health center-based prospective study. J Nutr. 2022;152:2245–54. doi:10.1093/jn/nxac136. [Google Scholar] [PubMed] [CrossRef]
98. Kikut J, Konecka N, Ziętek M, Kulpa D, Szczuko M. Diet supporting therapy for inflammatory bowel diseases. Eur J Nutr. 2021;60:2275–91. doi:10.1007/s00394-021-02489-0. [Google Scholar] [PubMed] [CrossRef]
99. Patel P, Butani K, Kumar A, Singh S, Prajapati BG. Effects of fermented food consumption on non-communicable diseases. Foods. 2023;12(4):687. doi:10.3390/foods12040687. [Google Scholar] [PubMed] [CrossRef]
100. Shabbir U, Rubab M, Daliri EBM, Chelliah R, Javed A, Oh DH. Curcumin, Quercetin, catechins and metabolic diseases: the role of gut microbiota. Nutr. 2021;13(1):206. doi:10.3390/NU13010206. [Google Scholar] [PubMed] [CrossRef]
101. Juárez-Fernández M, Porras D, Petrov P, Román-Sagüillo S, García-Mediavilla MV, Soluyanova P, et al. The synbiotic combination of Akkermansia muciniphila and quercetin ameliorates early obesity and NAFLD through gut microbiota reshaping and bile acid metabolism modulation. Antioxid. 2021;10:2001. doi:10.3390/ANTIOX10122001/S1. [Google Scholar] [CrossRef]
102. Tan S, Caparros-Martin JA, Matthews VB, Koch H, O’Gara F, Croft KD, et al. Isoquercetin and inulin synergistically modulate the gut microbiome to prevent development of the metabolic syndrome in mice fed a high fat diet. Sci Rep. 2018;8(1):1–13. doi:10.1038/s41598-018-28521-8. [Google Scholar] [PubMed] [CrossRef]
103. Arai Y, Watanabe S, Kimira M, Shimoi K, Mochizuki R, Kinae N. Dietary intakes of flavonols, flavones and isoflavones by Japanese women and the inverse correlation between quercetin intake and plasma LDL cholesterol concentration. J Nutr. 2000;130(9):2243–50. doi:10.1093/jn/130.9.2243. [Google Scholar] [PubMed] [CrossRef]
104. Cano-Martínez A, Bautista-Pérez R, Castrejón-Téllez V, Carreón-Torres E, Pérez-Torres I, Díaz-Díaz E, et al. Resveratrol and quercetin as regulators of inflammatory and purinergic receptors to attenuate liver damage associated to metabolic syndrome. Int J Mol Sci. 2021;22(16):8939. doi:10.3390/ijms22168939. [Google Scholar] [PubMed] [CrossRef]
105. Dewick PM. Medicinal natural products. In: Medicinal natural products: a biosynthetic approach. 3rd ed. New York: John Wiley & Sons; 2009. p. 1–539. doi:10.1002/9780470742761. [Google Scholar] [CrossRef]
106. Kumar G, Jayaveera K, Ashok Kumar C, Sanjay UP, Vrushabendra Swamy B, Kishore Kumar D, et al. Antimicrobial effects of Indian medicinal plants against acne-inducing bacteria. Trop J Pharm Res. 2007;6(2):717–23. doi:10.4314/tjpr.v6i2.14651. [Google Scholar] [CrossRef]
107. Parekh J, Karathia N, Chanda S. Evaluation of antibacterial activity and phytochemical analysis of Bauhinia variegata L. bark. Afr J Biomed Res. 2006;9:53–6. [Google Scholar]
108. Mallikharjuna PB, Rajanna LN, Seetharam YN, Sharanabasappa GK. Phytochemical studies of Strychnos potatorum L.f.–A medicinal plant. J Chem. 2007;4:510–8. doi:10.1155/2007/687859. [Google Scholar] [CrossRef]
109. Onwukaeme DN, Ikuegbvweha TB, Asonye CC. Evaluation of Phytochemical constituents, antibacterial activities and effect of exudate of Pycanthus Angolensis weld warb (Myristicaceae) on corneal ulcers in rabbits. Trop J Pharm Res. 2007;6:725–30. doi:10.4314/tjpr.v6i2.14652. [Google Scholar] [CrossRef]
110. Parekh J, Chanda S. In vitro antimicrobial activity and phytochemical analysis of some Indian medicinal plants. Turk J Biol. 2007;31:53–8. [Google Scholar]
111. Akinyemi KO, Oladapo O, Okwara CE, Ibe CC, Fasure KA. Screening of crude extracts of six medicinal plants used in South-West Nigerian unorthodox medicine for anti- methicillin resistant Staphylococcus aureus activity. BMC Complement Altern Med. 2005;5:6. doi:10.1186/1472-6882-5-6. [Google Scholar] [PubMed] [CrossRef]
112. Edeoga HO, Okwu DE, Mbaebie BO. Phytochemical constituents of some Nigerian medicinal plants. Afr J Biotechnol. 2005;4(7):685–8. doi:10.5897/AJB2005.000-3127. [Google Scholar] [CrossRef]
113. Atanasov AG, Waltenberger B, Pferschy-Wenzig EM, Linder T, Wawrosch C, Uhrin P, et al. Discovery and resupply of pharmacologically active plant-derived natural products: a review. Biotechnol Adv. 2015;33:1582–614. doi:10.1016/j.biotechadv.2015.08.001. [Google Scholar] [PubMed] [CrossRef]
114. Parsaeimehr A, Sargsyan E, Vardanyan A. Expression of secondary metabolites in plants and their useful perspective in animal health. ABAH Bioflux. 2011;3:115–24. [Google Scholar]
115. Jain S, Jain V, Mahajan SC. Lipid based vesicular drug delivery systems. Adv Pharm. 2014;2014:574673. doi:10.1155/2014/574673. [Google Scholar] [CrossRef]
116. Bassolé IHN, Lamien-Meda A, Bayala B, Tirogo S, Franz C, Novak J, et al. Composition and antimicrobial activities of lippia multiflora moldenke, Mentha× piperita L. and Ocimum basilicum L. essential oils and their major monoterpene alcohols alone and in combination. Mol. 2010;15:7825–39. doi:10.3390/molecules15117825. [Google Scholar] [PubMed] [CrossRef]
117. Schweiger R, Baier MC, Persicke M, Müller C. High specificity in plant leaf metabolic responses to arbuscular mycorrhiza. Nat Commun. 2014;5(1):3886. doi:10.1038/ncomms4886. [Google Scholar] [PubMed] [CrossRef]
118. Verma N, Shukla S. Impact of various factors responsible for fluctuation in plant secondary metabolites. J Appl Res Med Aromat Plants. 2015;2(4):105–13. doi:10.1016/j.jarmap.2015.09.002. [Google Scholar] [CrossRef]
119. Woo JW, Kim J, Kwon SII, Corvalán C, Cho SW, Kim H, et al. DNA-free genome editing in plants with preassembled CRISPR-Cas9 ribonucleoproteins. Nat Biotechnol. 2015;33(11):1162–4. doi:10.1038/nbt.3389. [Google Scholar] [PubMed] [CrossRef]
120. Rout GR, Samantaray S, Das P. In vitro manipulation and propagation of medicinal plants. Biotechnol Adv. 2000;18(2):91–120. doi:10.1016/S0734-9750(99)00026-9. [Google Scholar] [PubMed] [CrossRef]
121. Sharma M, Gupta RK, Khajuria R, Mallubhotla S, Ahuja A. Bacoside biosynthesis during in vitro shoot multiplication in Bacopa monnieri (L.) Wettst. grown in Growtek and air lift bioreactor. Indian J Biotechnol. 2015;14:547–51. [Google Scholar]
122. Chen W, Viljoen AM. Geraniol—A review of a commercially important fragrance material. S Afr J Bot. 2010;76(4):643–51. doi:10.1016/j.sajb.2010.05.008. [Google Scholar] [CrossRef]
123. Vasilev N, Schmitz C, Grömping U, Fischer R, Schillberg S. Assessment of cultivation factors that affect biomass and geraniol production in transgenic tobacco cell suspension cultures. PLoS One. 2014;9(8):e104620. doi:10.1371/journal.pone.0104620. [Google Scholar] [PubMed] [CrossRef]
124. Rahimi M, Farhadi R, Balashahri MS, Raeisi AS. Applications of new technologies in medicinal plant. Int J Agron Plant Prod. 2012;3:128–31. [Google Scholar]
125. Georgiev MI, Eibl R, Zhong JJ. Hosting the plant cells in vitro: recent trends in bioreactors. Appl Microbiol Biotechnol. 2013;97:3787–800. doi:10.1007/s00253-013-4817-x. [Google Scholar] [PubMed] [CrossRef]
126. Huang TK, McDonald KA. Bioreactor systems for in vitro production of foreign proteins using plant cell cultures. Biotechnol Adv. 2012;30:398–409. doi:10.1016/j.biotechadv.2011.07.016. [Google Scholar] [PubMed] [CrossRef]
127. Martins-Gomes C, Souto EB, Silva AM. Nanophytosomes: a novel approach for the delivery of herbal drugs. Syst Nanovesic Drug Deliv. 2022;103(10):239–57. doi:10.1016/B978-0-323-91864-0.00015-2. [Google Scholar] [CrossRef]
128. Souto EB, Dias-Ferreira J, Oliveira J, Sanchez-Lopez E, Lopez-Machado A, Espina M, et al. Trends in atopic dermatitis- from standard pharmacotherapy to novel drug delivery systems. Int J Mol Sci. 2019;20(22):5659. doi:10.3390/ijms20225659. [Google Scholar] [PubMed] [CrossRef]
129. Dewi MK, Chaerunisaa AY, Muhaimin M, Joni IM. Improved activity of herbal medicines through nanotechnology. Nanomater. 2022;12(22):4073. doi:10.3390/nano12224073. [Google Scholar] [PubMed] [CrossRef]
130. Subramanian AP, Jaganathan SK, Manikandan A, Pandiaraj KN, Gomathi N, Supriyanto E. Recent trends in nano-based drug delivery systems for efficient delivery of phytochemicals in chemotherapy. RSC Adv. 2016;6(54):48294–314. doi:10.1039/C6RA07802H. [Google Scholar] [CrossRef]
131. Kumar M, Bishnoi RS, Shukla AK, Jain CP. Techniques for formulation of nanoemulsion drug delivery system: a review. Prev Nutr Food Sci. 2019;24(3):225–34. doi:10.3746/pnf.2019.24.3.225. [Google Scholar] [PubMed] [CrossRef]
132. Németh Z, Pallagi E, Dobó DG, Kozma G, Kónya Z, Csóka I. An updated risk assessment as part of the QbD-based liposome design and development. Pharm. 2021;13(7):1071. doi:10.3390/PHARMACEUTICS13071071. [Google Scholar] [PubMed] [CrossRef]
133. Liu Y, Castro Bravo KM, Liu J. Targeted liposomal drug delivery: a nanoscience and biophysical perspective. Nanoscale Horiz. 2021;6:78–94. doi:10.1039/D0NH00605J. [Google Scholar] [PubMed] [CrossRef]
134. Kyriakoudi A, Spanidi E, Mourtzinos I, Gardikis K. Innovative delivery systems loaded with plant bioactive ingredients: formulation approaches and applications. Plants. 2021;10(6):1238. doi:10.3390/PLANTS10061238. [Google Scholar] [PubMed] [CrossRef]
135. Chavda VP, Vihol D, Mehta B, Shah D, Patel M, Vora LK, et al. Phytochemical-loaded liposomes for anticancer therapy: an updated review. Nanomed. 2022;17:547–68. doi:10.2217/nnm-2021-0463. [Google Scholar] [PubMed] [CrossRef]
Cite This Article
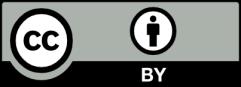