Open Access
REVIEW
Do tensile and shear forces exerted on cells influence mechanotransduction through stored energy considerations?
1 Department of Pathology and Laboratory Medicine, Robert Wood Johnson Medical School, Rutgers, The State University of New Jersey, Piscataway, NJ 08854, USA
2 OptoVibronex, LLC., Ben Franklin Tech Partners, Bethlehem, PA 18015, USA
* Corresponding Author: FREDERICK H. SILVER. Email:
BIOCELL 2024, 48(4), 525-540. https://doi.org/10.32604/biocell.2024.047965
Received 23 November 2023; Accepted 26 February 2024; Issue published 09 April 2024
Abstract
All tissues in the body are subjected externally to gravity and internally by collagen fibril and cellular retractive forces that create stress and energy equilibrium required for homeostasis. Mechanotransduction involves mechanical work (force through a distance) and energy storage as kinetic and potential energy. This leads to changes in cell mitosis or apoptosis and the synthesis or loss of tissue components. It involves the application of energy directly to cells through integrin-mediated processes, cell-cell connections, stretching of the cell cytoplasm, and activation of the cell nucleus via yes-associated protein (YAP) and transcriptional coactivator with PDZ-motif (TAZ). These processes involve numerous complexes, intermediate molecules, and multiple pathways. Several pathways have been identified from research studies on vertebrate cell culture and from studies in invertebrates. These pathways involve mechanosensors and other molecules that activate the pathways. This review discusses the mitogen-activated protein kinase (MAPK) family, Hippo, Hedgehog, and Wingless-related integration site (WNT)/β catenin signaling pathways. The mediators covered include β catenin, ion channels, growth factors, hormone receptors, members of the Ras superfamily, and components of the linker of nucleoskeleton and cytoskeleton (LINC) complex. However, the interrelationship among the different pathways remains to be clarified. Integrin-mediated mechanotransduction involves direct tensile loading and energy applied to the cell membrane via collagen fibril stretching. This energy is transferred between cells by stretching the cell-cell connections involving cadherins and the WNT/β catenin pathway. These alterations induce changes in intracellular events in the cytoskeleton and nuclear skeleton caused by the release of YAP and TAZ. These coactivators then penetrate through the nuclear pores and influence nuclear cell function. Alteration in the balance of forces and energy applied to cells and tissues is hypothesized to shift the cell-extracellular matrix mechanical equilibrium by modifying mechanotransduction. The shift in equilibrium can lead to either tissue synthesis, genetic modifications, or promote fibrotic diseases, including epithelial cell-derived cancers, depending on the local metabolic conditions.Keywords
Substrate stiffness has been reported as one of the regulators of cellular behavior [1,2]. In addition, gravity and other forces affect mammalian cells and tissues, which lead to the activation of numerous pathways that alter the interactions between cells and extracellular matrices (ECMs) [3]. At equilibrium, all macromolecular components adopt the lowest free energy configurations to stabilize cells and tissues by balancing the forces applied to the interfaces [4,5]. This lowest free energy state is influenced by the exact nature of forces and applied energy influencing the cells and the ECM. Given that cells and ECM are normally tightly connected, any change in either external or internal forces that alter the kinetic energy (rate of movement) and potential energy (extent of stretching) of both alters this equilibrium (Fig. 1). Further, changes in cellular and ECM properties associated with aging and UV light exposure also affect this equilibrium. Mechanotransduction is a process by which mechanical forces and applied energy alter cellular and matrix composition and behavior. Recent work provides evidence that free energy considerations can provide important information on the stability of cancerous tissue [6]. Several mechanical parameters that are involved in mechanotransduction are listed in Table 1.
Figure 1: Diagram illustrating the external forces acting on the skin, including gravity, cellular tension in the epidermis and dermis, and collagen fiber tension along Langer’s lines. (A) The forces on the skin include gravity, tension in the epidermis, collagen fiber tension at angles of +/−33.5 degrees in the dermis with respect to Langer’s lines. (B) is an expanded view of the figure shown in (A). It shows the average tension in collagen fibers in the general direction of Langer’s lines and the cellular forces balancing the tension in the fibers. Energy stored due to the stretching of cell-cell connections, cell-collagen attachments, and collagen-collagen crosslinks is proportional to the net tension times the strain. The potential energy contained in the cells and collagen fibers is balanced during homeostasis. Alteration in this balance due to increased forces and deformations leads to changes in the mechanotransduction equilibrium.
Given that both cells and tissues are highly viscoelastic, their behavior is time-dependent [5,7,8]. Further, the energy storage ability of ECM is highly dependent on the tissue strain because the stress-strain curve for collagenous tissues is J-shaped, and the area under the stress-strain curve is proportional to the amount of energy stored [5,7,8].
Results of cell culture studies on substrates of different stiffnesses provide an incentive to examine further whether substrate stiffness alone dictates cell and tissue responses [1,2]. Since both cells and tissues are viscoelastic and their behavior is time and strain-dependent, the tissue kinetic and potential energy transferred at the interface between cells and ECM can emerge as important in understanding mechanotransduction [5,7].
The cell-cell and cell-ECM interactions at equilibrium are affected by increased or decreased loading. In this paper, we attempt to analyze how energy storage and viscoelasticity influence mechanotransduction [5–7]. These properties are important in understanding how tissues perform their intended use and prevent premature mechanical failure. Applied forces on cells and tissues are converted into work (force moving through a distance) and lead to both cell and tissue elastic tensile stretching (reversible elastic behavior) and shear deformation (viscous behavior). This leads to changes in the tissue shape through shear and fluid flow. Tensile and shear loading are two loading conditions important in understanding the mechanisms by which mechanotransduction influences normal tissue maintenance and disease progression. Most soft tissues are highly viscoelastic, given that they are made of 70%–80% water [8]. During mechanical loading, soft tissues not only stretch and compress but also exude fluid like articular cartilage [9]. Fluid flow out of and into the ECM provides important mechanical stimuli affecting many tissues, including cartilage and vessel walls.
The force equilibrium and energy stored in cells and ECM are modified in reduced gravitational environments or under increased mechanical loading. Under these conditions, altered cell behavior and ECM composition change tissue and organ function in cardiovascular, ocular, dermal, musculoskeletal, immune, pulmonary, and other systems [10,11]. Exposure to microgravity is linked to changes in mechanotransduction; however, many of these changes are reversible. Therefore, changes associated with exposure to reduced gravitational forces must be only one variable influencing mechanotransduction. Mechanotransduction can be influenced by additional changes in macrophages [12], and other immune cells and eventually lead to cancerous tissue formation and fibrosis [13]. Given these observations, the relationship between mechanical loading, energy storage, cell and tissue aging, disease, and tissue mechanotransduction is complicated.
This review aims to relate information available in the literature on the consequences of tensile and shear loading on cells and ECM to mechanotransduction and energy storage in tissues. The ability of tissues and organs to store and dissipate energy efficiently is an important physiological function of tissues [14–16]. This ability decreases with age, leading to loss of tissue strength and compromised locomotion. The approach taken in this paper explores the role of energy storage in maintaining an equilibrium required for normal physiological functions of tissues and organs [14–16]. Alteration in this energy equilibrium between cells and ECM leads to the removal of unused tissue components (atrophy) or the creation of new tissue to support the new energy requirements [14–16]. In addition, new energy storage requirements alter mechanotransduction, which can lead to fibrosis and tissue pathology in diseases such as epithelial cell-derived cancers [17].
The balance between forces and energy applied by cells to a substrate is related to the boundary forces between the substrate and cells (Newton’s Third Law). Increased stiffness of implants over that of neighboring soft tissue leads to intimal hyperplasia in vascular grafts, capsular contraction around breast implants, and failure of hernia implants at the tissue interface [14]. These pathological conditions result from excess energy applied to the tissue due to the large cellular strains that occur when soft tissues are connected to stiffer implants. This results in increased energy applied at the interface, especially when the stiffness of the implant and tissue are not matched [18]. Large cellular and tissue strains increase the energy applied and stored in these components that must be dissipated within cells before a new cell-ECM equilibrium can occur.
How does altered cell and tissue free energy influence mechanotransduction?
Mechanotransduction involves mechanical work (force through a distance) and energy storage as kinetic and potential energy that leads to changes in cell mitosis or apoptosis and the synthesis or loss of tissue components [3,14–16]. Cells sense mechanical forces that do work (mechanical energy), such as providing tissue displacement required for locomotion, and convert them into changes in chemical energy (synthesis of new tissue) by upregulation of mechanotransduction [1,3]. In contrast, tissue disuse and decreased internal energy cause tissue atrophy and cellular loss through down-regulation of mechanotransduction.
For example, work done on tendons (force applied through a distance) by muscular forces results in mechanotransduction [19]. This is converted into new tissue deposition after repeated exercise [3]. The size of muscle cells increases (muscle cell hypertrophy) and the skin eventually expands through cellular hyperplasia and protein synthesis due to weightlifting. As a result, more skin is made eventually to cover the larger muscles [3,14–16]. Energy storage in tendons, followed by transmission to joints, eventually leads to excess energy dissipation as heat in muscle (Fig. 2) [20]. Energy storage, transmission, and eventual dissipation are important functions of all mammalian tissues that allow for locomotion and movement and protect against premature mechanical failure [3,5]. Energy dissipation in the skin, mineralized tissue, cartilage, blood vessels, cornea, and posterior segment of the eye protects these tissues from tearing, delamination, and excessive stiffening during mechanical loading [21–24].
Figure 2: Energy storage, transmission, and dissipation in tissues. (A) Diagram of how energy storage is related to the force times the deformation or strain. In the tendon, a muscle creates force, and the tendon stores work as force times displacement. (B) Energy transmission from the tendon is stored in the joint for driving locomotion. Excess energy in joints is then transferred back to the muscle through the tendon, where it is dissipated as heat. (C) Energy stored in tissue during stretching (area under the force-displacement curve during loading) is returned during unloading (area under the unloading force vs. displacement curve) and dissipated as heat (proportional to the difference between areas under loading and unloading curves). Excess energy is dissipated in muscle as heat or in other soft tissues by fluid flow.
The tensile and shear loading of cells and tissues increases their kinetic and potential energy and leads to conformational changes in the macromolecular components [3,5,22]. When macromolecules are stretched in tension, potential energy is stored in individual macromolecules by decreasing the available rotational angles and extending the chain structure in space [3,22]. The energy stored on stretching macromolecules and tissues is available to modify mechanotransduction [3,22].
Shear forces in tissues are a result of fluid flow, either due to tensile or compressible loading that leads to energy transfer through fluid viscosity. Viscosity is a measure of kinetic energy losses due to frictional forces that occur between molecules and surfaces [3]. Kinetic energy losses can be converted into potential energy in cells and tissues like how energy is transferred from flowing blood to the vessel walls [24]. This transferred energy has the potential to alter mechanotransduction and composition in many tissues.
Molecules, complexes and pathways involved in mechanotransduction
Several molecules, complexes, and pathways involved in mechanotransduction have been studied in mammals and invertebrates. Some of the important molecules, pathways, and complexes associated with mechanotransduction are listed in Table 2. These are only a few of the potential elements involved in mechanotransduction that have been documented, it is evident that many other molecules and pathways may be involved that are not covered in this review. Given that the interrelationships between these elements are not yet clear at this time, only an overview will be presented here.
Efficient cellular mechanotransduction requires mechanical connections between the extracellular matrix elements, including collagen fibrils [25] and fibronectin [26], the surrounding cells, and tissue components. Integrin binding to ECM stimulates mechanotransduction as well as growth factor and hormone receptors activation, focal adhesion formation, ion channel stimulation, activation of mitogen-activated protein kinase (MAPK) [27–29], extracellular regulated kinase (Erk) 1/2 [29], p38, c-Jun N-terminal kinase (JNK) [30], ERK 5, Hippo [28], Hedgehog [31], WNT/β catenin [32–34], and integrin-mediated pathways [35–38]. Each of these pathways and molecules is described below, along with the possible interrelationships. Tensile forces appear to cause stretching and energy storage in cells and tissues that stimulate integrin and other pathways mediating mechanotransduction. This may also result in generating shear forces and fluid flow, affecting ion channels and hormone receptor activation.
Pathways and mediators involved in mechanotransduction
Numerous pathways have been identified from research on vertebrate cell culture and from studies in invertebrates. These pathways include mechanosensors and other molecules that activate pathways. The pathways discussed in this review include MAPK Family, Hippo, Hedgehog, and WNT/β catenin [27–29]. Several mediators are also covered [30], including ion channels, growth factors, hormones, growth factor, and hormone receptors, members of the Ras superfamily, and components of the LINC (linker of nucleoskeleton and cytoskeleton complex) [31–33]. Each of the pathways is discussed with examples of how pathway dysregulation in the liver, lung, pancreas, vessel, epithelial-derived cancers, and microgravity occurs [34–37]. Finally, an attempt is made to relate the complex roles of cells and ECM in energy balancing during homeostasis and the unbalancing during disease progression and cancer.
Forces and mechanical energy applied to cells externally affect integrin-based adhesion sites that involve collagen, fibronectin, and other extracellular matrix molecules [38]. At equilibrium, the forces applied to the cell surface are balanced by the forces generated by the cell through the cell cytoskeleton and nuclear skeleton, as shown in Figs. 3 and 4. In addition, the energy required to be borne by the cell and transmitted away from the cell to prevent cell death may depend on the cell-cell connections. Tissue homeostasis entails mechanical equilibrium among external tissue forces, applied energy, and cellular resistance. This would suggest that failure to maintain this equilibrium may either up-regulate or down-regulate mechanotransduction. Applied forces acting at the cell-ECM interface may stimulate new tissue growth or tissue catabolism, depending on the pathway activated. Further, reduced forces at the cell-ECM interface may down-regulate mechanotransduction, leading to tissue atrophy and cellular apoptosis [3]. In this way, the balance of forces and applied energy at the cell-ECM interface may regulate the effects of mechanotransduction in homeostasis and tissue pathology by stimulating different pathways depending on the exact tissue loading and genetic conditions.
Figure 3: Diagrammatic representation of the force balance at the extracellular matrix (ECM)–cell junction. The collagen fibrils are attached to the cell membrane through integrins (top). When collagen fibrils are stretched, the cell shape changes, and energy is stored in the cell as strain energy (bottom). Note that tensile forces cause the stretching of collagen fibrils that lead to the stretching of attached cells through cell–cell junctions. At equilibrium, all net stresses applied to the collagen fibrils and cells must be zero.
Figure 4: Connections between collagen fibrils, integrins, and the cell membrane. Collagen fibrils comprise alternating flexible (white lines on top) and rigid (black lines on top) regions that bind to the α and β integrin subunits, which connect to the cell membrane and actin cytoskeleton through focal adhesions. Tension in the collagen fibrils can induce tension in the attached cell membrane. Both the collagen fibril and the cellular membrane tension can lead to changes in the cell cytoplasm and actin-myosin components of the cytoskeleton (see Fig. 5) and modify mechanotransduction.
Figure 5: Diagram showing connections between α and β integrins, collagen fibrils, focal adhesion kinase (FAK), kindlin (a focal adhesion activation protein), talin (a membrane-associated protein), vinculin (mediates adhesion to cellular actin), actin, and myosin. Collagen molecules in the form of fibrils adhere to cell surface integrins, activating force and energy transfer to the cell cytoskeleton containing actin and myosin via associated proteins FAK, kindlin, talin, and vinculin. Activated cytoskeletal molecules can transfer this energy to the nucleus via yes-associated protein (YAP), transcriptional co-activator with PDZ binding motif (TAZ), and the linker of nucleoskeleton and cytoskeleton (LINC) complex.
Integrins are heterodimeric transmembrane receptors composed of α and β subunits that bind to molecules in the ECM, such as collagen fibrils, as illustrated in Fig. 4 [37,38]. Activated integrins are dynamically coupled to the actomyosin cytoskeletal system through integrin- and F-actin–binding proteins, such as talin and vinculin (refer Fig. 5) [39,40]. The applied forces and the resulting cellular and tissue strains change the amount of stored energy. Stored energy is converted into protein and macromolecular conformation changes that can then be converted into kinetic energy required for cell shape changes or fluid movement between cells and tissues. The resulting equilibrium at the cell-ECM interface will depend on the amount of energy applied to the interface. The increased cell stiffness of epithelial-derived cancer cells [17] affects the energy stored at cell-cell interfaces and at cell-ECM interfaces, altering the equilibrium resting point for mechanotransduction. At cell-cell adhesion sites, integrins connect the ECM and collagen fibrils with the F-actin cytoskeleton, allowing energy storage that may be involved in actin retrograde flow. This occurs through mechanosensitive focal adhesion proteins that are collectively termed the “molecular clutch” [41–43]. The transmission of forces and strains (applied energy) across integrin-based adhesions establishes a mechanical equilibrium between the ECM and the cell cytoskeleton (Fig. 5). This equilibrium can be altered by changes in tissue loading, and strain and activation of ion channels, growth factor and hormone receptors, and other cellular effects. Further, the energy supplied by collagen-integrin interactions during stretching can induce stretching of the cell membrane and focal adhesion clustering. Collagen-induced cell membrane stretching may affect other parts of the cell membrane, including growth factors, hormones, and ion channel receptors. The energy storage and dissipation ability of the ECM and the attached cells are modified by ECM crosslinking that occurs with age and subsequent fragmentation of collagen and elastic fibers after UV light exposure [17]. This resulting imbalance may alter the mechanical equilibrium between cells and ECM and explain some changes that occur during normal aging [41].
Focal adhesions (FAs) are the macromolecular assemblies of proteins serving as the primary link connecting the actin cytoskeleton to the ECM through the integrin family of receptors [43]. These assemblies are large, elongated structures, typically about 2 mm wide and 3–10 mm long, in which clustered integrins bind ECM fibrils on the outside of the cell and connect on the inside to contractile actomyosin stress fiber bundles [43] (refer Figs. 5 and 6). Further, FAs and other integrin-associated complexes are fundamental components of mechanotransductive machinery. They work as an interface bridging the ECM with the cytoskeleton and inner cellular space [39–41]. The formation of focal adhesions through integrin clustering has been proposed to regulate the Rho signaling pathway, which controls myosin II phosphorylation and affects the cytoskeleton tension in adherent cells. Additionally, actin filaments connect directly to the nucleus through LINC complex proteins [41–43]. Tension and energy applied through the attachments with the ECM may lead to FA clustering and provide additional energy to promote the activation of nuclear involvement in mechanotransduction.
Figure 6: Diagrammatic relationship between focal adhesions and the clustering of integrin subunits. Focal adhesions consist of localized integrin assemblies that are activated by forces and energy applied by the attached collagen fibrils. Focal adhesions increase the force and energy applied to the cell membrane by clustering, which results in stress concentration.
Given that the integrin cytoplasmic domain lacks an actin-binding site, other proteins are required to complete the actin-integrin-ECM linkage [42–44]. Proteomic studies have unveiled integrin-actin-linking proteins as members of what has been described as the ‘integrin adhesome’ containing more than 500 proteins [42–44]. Some of these proteins have been shown in Fig. 5. The mechanism of coupling between integrins and actin during cell migration is called the ‘molecular clutch’ hypothesis [42,43]. This coupling may be dependent on collagen-integrin binding and energy transfer from the extracellular matrix.
During stretching, collagen molecules store energy in the flexible regions of the triple helix that have no proline and hydroxyproline residues [3,4]. These regions are found in the b2 and d bands on collagen fibrils where integrins bind to collagen fibrils [15]. These regions store changes in free energy via reversible conformational changes during mechanical deformation [22]. Stretching of these regions increases the free energy of both collagen and attached cells [3,22]. Further, these regions are devoid of proline and hydroxyproline residues [3,4,22]. The energy derived from this tension can then be transferred through the cell membrane to the cell cytoskeleton and eventually to the nucleus. Activated integrins are dynamically coupled to the actomyosin skeletal system through integrin- and F-actin–binding proteins, such as talin, kindlin, and vinculin [39,40] (see Figs. 4 and 5). Binding of integrins to the ECM induces their clustering and formation of the adhesion complex. Clustering of focal adhesions increases the amount of stored energy that can be transferred through the cell cytoskeleton, leading to cell nuclear changes.
The connections between the cell membrane and cytoskeleton include several molecules, such as FAK, kindlin, talin, and vinculin. Talins are cytoskeletal linker proteins that consist of an N-terminal head domain, a flexible neck region, and a C-terminal rod domain made of 13 helical bundles. Activation of FAs and the cell cytoplasmic components leads to stress concentration and higher levels of energy applied to the cell components, as diagrammed in Fig. 6.
Mitogen-activated protein kinase family mediated pathways
Once integrin-ECM interactions stimulate focal adhesion formation and clustering at the cell-ECM interface, this activates MAPK pathways, including ERK 1/2 (Fig. 7). All eukaryotic cells possess multiple MAPK pathways, which coordinately regulate gene expression, mitosis, metabolism, motility, survival, apoptosis, and differentiation. The conventional MAPK pathways include ERK 1/2, p38, JNK, and ERK 5 (see Fig. 7) [27]. Further, ERK 1/2 is activated by integrins and epidermal growth factor (EGF) and leads to modification of transcription in the cell nucleus. The molecules involved in the MAPK pathways are numerous and lead to several biological functions, including altered protein synthesis and cell division [27]. The pathway can also be activated by the activation of several different mechanosensing mechanisms. The ERK pathway is activated by extracellular matrix stresses, growth factors, and hormones [27,44–46]. For instance, flow stimulation of focal adhesion kinase (FAK) in arterial cells leads to MAP kinase pathway activation in endothelial cells [10,47]. Furthermore, stretching and activation of collagen-integrin connections can potentially activate ion channels, growth factor and hormone receptors, and other pathways associated with mechanotransduction. It is also possible that any membrane perturbation that provides excess energy to the cell membrane may influence mechanotransduction pathways.
Figure 7: The mitogen-activated protein kinase (MAPK) family of pathways includes the extracellular regulated kinase (ERK 1/2), c-JUN-terminal kinase (JNK), p38, and ERK [27]. The extracellular regulated kinase (ERK 1/2) cascade is stimulated by growth factors including epidermal growth factor (EGF), mechanical forces, and factors released during inflammation. The JNK pathway is involved in stress signaling and leads to cell differentiation and proliferation. The p38 mitogen-activated protein kinases are responsive to stress stimuli, UV radiation, and cytokines. These are involved in cell differentiation, apoptosis, and autophagy. p38 is also activated by stress stimuli and promotes cell proliferation and differentiation.
The Hippo (Salvador-Warts-Hippo) pathway (Fig. 8) is a highly conserved signaling pathway in higher-order vertebrates that regulates biological processes, including cellular proliferation, differentiation, organ size, and tissue homeostasis [28,30]. The dysregulation of the Hippo pathway is believed to be involved in excessive tissue synthesis that leads to the uncontrolled growth of tumors [28,30]. Yes-associated protein (YAP) and transcriptional co-activator with PDZ-binding motif (TAZ) are the major downstream complexes of the Hippo pathway that regulate tissue homeostasis, organ size, regeneration, and tumor formation. These factors have received much attention given that their regulation is potentially disrupted in cancer [48,49]. Focal adhesion kinase (FAK) is activated by flow stimulation in arterial cells [47], leading to MAP kinase pathway activation in endothelial cells. This indicates that the Hippo and MAP kinase pathways are interrelated (see Fig. 7). These pathways may be stimulated by forces applied to the ECM. Upon activation, YAP and TAZ migrate through pores through the nuclear membrane to affect cellular DNA [48,49]. It is not clear whether energy transfer to the cell cytoskeleton through collagen-integrin stretching can activate FAK and the MAPK pathway. However, this may be the link that connects integrin-mediated mechanotransduction with YAP and TAZ.
Figure 8: Diagram of the hippo pathway. This pathway regulates cell growth, proliferation, and apoptosis. This pathway may be related to integrin-mediated mechanotransduction through the activation of FAK, YAP, and TAZ. It is hypothesized that family of transcription proteins (TEAD) is required for the coactivation of YAP and TAZ. When YAP and TAZ enter the nucleus through the nucleoskeleton, gene expression can be altered.
Hedgehog pathway and mechanotransduction
Hedgehog (Hh) signaling pathway plays an essential role during vertebrate embryonic development and tumorigenesis [31,50,51]. There are three mammalian Hh proteins: Sonic Hedgehog (Shh), Indian Hedgehog (Ihh), and Desert Hedgehog (Dhh). The Shh pathway has been shown to be involved in the development and resistance of several tumor types [50]. Further, Hh is important for the organogenesis of almost all organs in mammals, as well as in regeneration and homeostasis. Additionally, Shh and Ihh have important, and sometimes coinciding, functions in several tissues. Shh signaling pathway can crosstalk with several pathways, especially EGF, WNT, and transforming growth factor β (TGFβ) [50]. This pathway is involved in cancer progression, given that inhibitors like vismodegib clinically block the growth of metastatic basal cell carcinomas [31]. However, whether this pathway interacts with other mediators of mechanotransduction remains to be studied.
The wingless-related integration site/β catenin pathway and mechanotransduction
The WNT/β catenin pathway (shown in Fig. 9) comprises a family of proteins that play critical roles in embryonic development and adult tissue homeostasis. The deregulation of WNT/β catenin signaling often leads to various serious diseases, including cancer and non-cancer conditions [32,33]. β catenin is a core component of the cadherin protein complex, the stabilization of which is essential for activating WNT/β catenin signaling [52–54]. The stability of β catenin is increased by its binding to N-cadherin because it is protected from degradation in the cytoplasm [52–54]. The N-cadherin to E-cadherin transition is suggested to be associated with epithelial-derived skin cancers [52–54].
Figure 9: Diagram of wingless-related integration site (WNT) activation of target genes in the nucleus via β catenin activation. The WNT signaling pathways are a group of signal transduction pathways that begin with WNT proteins binding to the cell membrane. It then passes signals into the cell that releases β catenin to affect target genes in the nucleus.
In the absence of WNT signaling, β catenin is degraded by protein complexes within the cell. When the destruction complex is broken, β catenin stabilizes and binds to TCF (T-cell factor/lymphoid enhancement factor) in the nucleus to regulate the target gene [52]. Abnormal activation of WNT/β catenin signaling promotes the progression of various diseases and the oncogenic transformation of tumors. This makes key factors involved in WNT/β catenin signal transduction attractive therapeutic targets for cancers [55]. The WNT/β catenin pathway may be involved in mechanotransduction related to the E-cadherin to N-cadherin transition.
Ion channels involved in mechanotransduction and other receptors (Fig. 10)
Piezo family of cation channels and membrane stretch receptors
The Piezo family forms mechanosensitive protein cation channels [56–58]. The Piezo channels play crucial roles in numerous physiological and pathological processes by functioning as cellular mechanotransducers [56,57]. Piezo1 and Piezo2 are large membrane proteins that consist of 2500–2800 amino acids [59–61]. Under mechanical stimuli, Piezo channels are opened to allow cationic ions to cross the cell membrane, which promotes cellular mechanotransduction to adapt to the microenvironment. Piezo1 is expressed in cells of the genitourinary system, especially in renal tubular epithelial cells and in uroepithelial cells. Studies on Piezo1-expressing HEK293 cells demonstrated shear stress activation of Piezo1 followed by calcium influx similar to endothelial cells [58]. Further, Piezo1 activation leads to cellular DNA activation.
Figure 10: Diagram of some of the mechanisms that alter cellular and genetic behavior of cells through mechanotransduction. The left side of the illustration shows how direct membrane, ion channel, growth factor, and hormone activation can lead to activation of the phosphorelay pathways, including extracellular regulated kinase (ERK 1/2). The arrows indicate that both collagen and cell membranes are under tension under normal physiological conditions. The right side of the figure shows how collagen-integrin interactions, growth factors, hormone receptors, and release of cytokines and interleukins activate phosphorelay pathways and alter mechanotransduction. Stress and energy equilibrium between cells and the ECM may be crucial in establishing mechanotransduction homeostasis in tissues and organs.
Hemodynamic shear stress is known to open many ion channels, including K+ channels, Cl- channels, transient receptor potential (TRP), and Piezo channels that transfer Ca2+ [53]. The Piezo genes, Piezo1 and Piezo2, encode exceptionally large mechanosensitive ion channels predicted to contain more than 14 transmembrane domains per monomer [50,54]. Expression of Piezo proteins is sufficient to confer mechanically induced ionic currents to cells [50,51], and these molecules are believed to be gated by force [55]. These channels are particularly sensitive to small changes in shear stress and fluid shear energies. This is because the energy levels involved during fluid flow are likely to be much lower than those involved in direct tensile or compressive deformation of the integrin-collagen connections. Further, ion channels may influence mechanotransduction when small changes in shear forces are too small to influence integrin-mediated changes.
Transient receptor potential vanilloid-type 4 and its role
Transient receptor potential vanilloid-type 4 (TRPV4) is a mechanosensitive, non-selective, Ca2+ cation channel. It is a non-selective, Ca2+ permeable cation channel [62]. TRPV4 is involved in calcium ion transport, cytoskeletal organization, and positive regulation of microtubule depolymerization [62,63]. TRPV4 permeable cation channel is widely expressed and responds to both chemical and mechanical signals [62]. TRPV4 activates calcium channels and leads to an influx into the cell nucleus. Like Piezo1, activation is a result of shear force mechanical stimuli. Membrane stretch may activate the TRPV4 channel, resulting in a Ca2+ influx [63].
Mechanical forces applied to cells can modify growth factor receptors and activate growth factor signaling (refer to Fig. 10). For instance, mechanical perturbations to epithelial E-cadherin receptors cause force-dependent activation of the epidermal growth factor receptor (EGFR) [64]. E-cadherin and EGFR form complexes at the plasma membrane that are disrupted by either EGF or increased tension on E-cadherin bonds. Although force applied to E-cadherin bonds disrupts the complex in the absence of EGF, dimerization of the EGF receptor activates growth factor signaling. Additionally, intercellular tension in epithelia lowers EGF concentrations, leading to a quiescent state of the epidermis [65]. While collagen-integrin interactions can transfer energy from the ECM to cells, energy transferred between cells through cell junctions may up-regulate mechanotransduction through growth factor receptor activation.
E-cadherin–mediated activation of EGFR signaling modulates local cytoskeletal remodeling and cell proliferation. E-cadherin and EGFR form a heteroreceptor complex at the membrane. Increased tension on E-cadherin bonds disrupts the complex in the absence of EGF. Further, integrin crosstalk with growth factor receptors stimulates growth factor signaling [64,65], suggesting that the two pathways are interrelated. Additionally, EGF modulates focal adhesions by amplifying levels of activated antagonists needed for cell motility. The observed EGF response underlies an EGFR-integrin crosstalk involving MAP kinase and other factors in the MAPK pathways [36].
Mechanosensitive hormone receptors are important in both the health of tissues and the development of cancer (Fig. 10). Prolonged changes in mechanical forces may further facilitate tumor initiation and the development of aggressive metastatic disease. Reproductive tissue cancers have been linked to aberrant hormone signaling. Estrogens and estrogen receptors modify how bone cells respond to mechanical strain [66]. Estrogens suppress bone resorption and formation, and estrogen withdrawal leads to reduced bone mass and increased risk of fracture [62]. Flow-mediated outward remodeling (FMR) has been documented in arteries via alterations in estrogen metabolism, oxidative stress, and inflammation. Estrogen and estrogen alpha receptor (ERα) play a key role in FMR by controlling dilatory pathways [67]. Flow-mediated mechanotransduction may be triggered through activation of both growth factor and hormone receptors.
Rho-associated GTP kinases (ROCK)-serine-threonine specific protein kinases-regulate cellular contraction, motility, morphology, polarity, cell division, and gene expression. As a subfamily of the Ras superfamily, Rho GTPases have been recognized as key intracellular mechanotransduction mediators that can regulate multiple cell activities, such as proliferation, migration, and differentiation, as well as biological processes such as cytoskeletal dynamics, metabolism, and organ development [49]. Mechanistically, integrins and FAs are involved in the complex signal network between ECM and Rho GTPases. Given that integrins and FAs link cells to the surrounding ECM, they are involved in the mechanical regulation of the Rho GTPase signaling [49,68].
Rho GTPases are regulators of cytoskeletal dynamics and are often described as molecular switches due to their ability to activate multiple downstream pathways. In this regard, ROCK has been shown to be an important modulator of actin-myosin interactions. An interplay between the Rho/ROCK pathway and transforming growth factor β (TGF-β) is evident as cell contraction requires ROCK activity independent of α-smooth muscle actin expression [69]. The RAS superfamily of kinases appears to be involved in downstream activation of the cytoskeleton involving integrins and the cytoskeleton. Given this finding, Rho GTPase signaling seems to be one of the mediators involved in integrin-mediated mechanotransduction.
There are two major protein networks in cells: the cytoskeleton in the cytoplasm network and the nucleoskeleton in the nucleus. They are separated by the nuclear envelope and connected through the linker of nucleoskeleton and cytoskeleton (LINC) complex embedded in the nuclear envelope [34]. The LINC complex is composed of two protein domains: the SUN domain, which spans the inner nuclear membrane, and the conserved C-terminal KASH domain, which spans the outer nuclear membrane [35]. Upon activation, YAP and TAZ can migrate into the nucleus through nuclear pores. Energy transfer through collagen-integrin stretching can activate FAK and the ERK1/2 pathway. This may be the link that connects integrin-mediated mechanotransduction, YAP, and TAZ and changes that occur in the cell nucleus associated with mechanotransduction.
Mechanotransduction and etiology of diseases
Several clinical studies suggest how alterations in forces and energy applied to tissues change the homeostasis and physiology of cells, tissues, and organs. These changes give us important information to understand mechanotransduction and the pathobiology of several diseases.
Prolonged space travel reduces cardiovascular capacity, impairs immune responses, reduces bone density, compromises neurological function, and leads to muscle atrophy to name a few of the consequences [11,16,70]. The liver, which is the largest visceral organ involved in detoxication and metabolism to maintain body homeostasis, also experiences changes due to gravity. YAP/TAZ target gene expressions appear to be altered under microgravity, suggesting that YAP/TAZ activation disrupts these pathways via cytoskeletal remodeling, nuclear deformation, or disturbing homeostasis [70–72]. In addition, microgravity exposure dysregulates liver functions, resulting in apoptosis and oxidative stress-associated liver injury and inflammation, compromised carbohydrate metabolism, altered hepatic xenobiotic biotransformation machinery, and lipid deposition [72].
Studies on the effect of reduced gravity on tissue homeostasis suggest that force and the resulting energy equilibrium must involve the ECM, integrins, cell cytoskeleton, YAP, and TAZ, as well as the cell nucleus. The results of these reports suggest that decreases in external forces on cells and tissues alter the energy balance and lead to changes that may contribute to diseases caused by mechanotransduction.
Plasma membrane disruption (PMD) caused by osmotic stress, mechanical forces, and ischemic injury in mammalian cells allows molecular flux across cell membranes that promotes tissue adaptation and mechanotransduction [73]. Calpains, annexins, growth factors, and nitric oxide (NO) have been reported to play roles in signaling downstream of PMD in several types of mechanosensitive tissues [74,75]. Further, PMD may play a role in integrin, ion channel, and cell-cell induced changes and be induced by tensile and shear loading of cells and tissues.
Mechanotransduction and beta cells
During development and adulthood, pancreatic beta-cells receive biochemical instructions from the extracellular environment and experience mechanical forces, including blood shear stress, and tensile and compressing forces, which are conveyed by cell-cell, and cell-matrix interactions [76]. The differentiation, proliferation, and function of pancreatic beta-cells are influenced by islet ECM stiffness, topography, geometry, and fluid shear forces.
Several cellular structures that have been reported to act as mechanosensors are expressed in beta cells. These include protein complexes of cell–ECM and cell–cell adhesions, primary cilia, stretch-activated ion channels, glycocalyx, G-protein coupled receptors, and growth factor receptors [77]. These reports suggest that a linkage exists between tensile integrin-induced and shear flow-induced changes in mechanotransduction, which may be important for organ homeostasis.
Vascular endothelium and mechanotransduction
Changes in shear stress associated with alterations in blood flow initiate a signaling cascade. Shear stress is sensed by a mechanosensitive complex on the endothelial membrane through ion channels [78]. In addition to the role of endothelium in mechanotransduction, vascular smooth muscle cells regulate contractility through the cell cytoskeleton. Cytoskeletal proteins are mechanosensitive and remodel their structure and function in response to external mechanical stimuli [78,79]. The linkage between changes in shear forces present during vessel blood flow and the resulting medial hyperplasia seen in atherosclerotic vessel walls further supports the existence of a mechanotransduction linkage between tensile integrin and shear flow-mediated mechanotransduction.
Mechanical ventilation can induce lung injury, which is known as ventilator-induced lung injury. Overdistension of the lung due to high tidal volumes or as a result of tensile distending pressures leads to alveolar rupture, resulting in lung disorders [80]. Another mechanism of lung injury results from cyclic collapse and opening of alveolar units [80]. Further, limiting tidal volume and plateau airway pressure based on body weight improves the survival of patients with compromised breathing. Mechanical ventilation support with high volumes and pressures can cause preventable morbidity and mortality in critically ill patients [81,82]. These observations suggest that the energy applied to the lungs during ventilation can lead to pathological consequences through changes in mechanotransduction.
Changes in mechanotranduction associated with cancer
Mechanotransduction and increased tissue stiffness have been documented in many cell types [1,2,32,49]. Mechanical signals initiate intracellular signaling pathways with a wide variety of effects, including activation of ion channels or protein kinases to long-term changes in cell phenotype that require initiation of gene transcription and protein production [83,84] The malignancy of metastatic ovarian cancer cells has been shown to be increased on soft matrices through a mechanosensitive Rho-ROCK pathway [85]. Further, matrix crosslinking forces support tumor progression by enhancing integrin signaling [86]. In another report, an increased metastatic potential is seen in a mechanically-induced colon cancer cell population showing an increased metastatic potential [87]. Furthermore, changes in cellular stiffness are also seen in epithelial-derived skin cancers [17]. Clearly, these studies suggest a relationship between substrate properties, mechanotransduction pathways, and cancer. The exact relationship between these properties and cell behaviors remains to be analyzed.
All tissues in the body are subjected externally to gravity and internally by cellular retractive forces that create stress and energy equilibrium required for homeostasis through mechanotransduction pathways. The balance of these forces and energies has not been fully investigated. However, it leads to changes in mechanotransduction and to changes in tissue structure, function, and displacement, as summarized in Fig. 10. Tissue displacement is altered as we walk, run, jump up and down, bicycle, or ride a roller coaster. All these displacements lead to energy storage in our tissues. In the cornea, this energy is transferred through the corneal-limbus-scleral junction to the back of the eye, where it is dissipated in the sclera and posterior chamber. If it were not transferred from the cornea and dissipated posteriorly, it would cause corneal delamination, collagen fibril slippage, and visual problems. In skin and cartilage, the applied energy is transferred away from its site of application through rearrangement of the collagen fibrils in the dermis of the skin and compression of the intermediate zone in cartilage. Energy is dissipated by fluid flow and collagen fibril arrangement along the axis of the highest stress concentration.
Mechanotransduction involves the application of energy directly to cells through integrin-mediated mechanotransduction, cell-cell connections, stretching of the cell cytoplasm, and activation of the cell nucleus by YAP and TAZ. While each of these processes involves numerous complexes and intermediate molecules, the interrelationships among the different pathways are still unclear. Integrin-mediated mechanotransduction likely involves direct tensile loading and energy being applied to the cell membrane via collagen fibril stretching. This energy is transferred between cells via the stretching of cell-cell connections involving cadherins and the WNT/β catenin pathway. Tensile stretching results in small changes in shear stress and shear energy due to the induction of fluid flow. This change may induce hormone and growth factor receptors and ion channel activation. These changes lead to intracellular events in the cytoskeleton and nuclear skeleton that cause the release of YAP and TAZ, which penetrate through the nuclear pores to affect nuclear cell function. Alteration in the balance of forces and energy applied to cells and tissues shifts the force and energy equilibria by modification of mechanotransduction. The shift in equilibria in some conditions can lead to morbidity and promote fibrotic diseases, including epithelial-derived cancers.
Acknowledgement: None.
Funding Statement: The authors received no funding for this study.
Author Contributions: The authors confirm their contribution to the paper as follows: Draft manuscript: FHS and TD. Both authors reviewed the results and approved the final version of the manuscript.
Availability of Data and Materials: Data sharing is not applicable to this article as no datasets were generated or analyzed during the current study.
Ethics Approval: Not applicable.
Conflicts of Interest: The authors declare that they have no conflicts of interest to report regarding the present study.
References
1. Leipzig ND, Shoichet MS. The effect of substrate stiffness on adult neural stem cell behavior. Biomaterials. 2009;30(36):6867–78. doi:10.1016/j.biomaterials.2009.09.002. [Google Scholar] [PubMed] [CrossRef]
2. Selig M, Lauer JC, Hart ML, Rolauffs B. Mechanotransduction and stiffness-sensing: mechanisms and opportunities to control multiple molecular aspects of cell phenotype as a design cornerstone of cell-instructive biomaterials for articular cartilage. Repair Int J Mol Sci. 2020;21(15):5399. doi:10.3390/ijms21155399. [Google Scholar] [PubMed] [CrossRef]
3. Silver FH. Mechanosensing and mechanochemical transduction in extracellular matrix. In: Biological, chemical, engineering, and physiological aspects. New York, NY: Springer; 2006. doi:10.1007/978-0-387-28176-6 [Google Scholar] [CrossRef]
4. Silver FH, Horvath I, Foran DJ. Mechanical implications of the domain structure of fiber-forming collagens: comparison of the molecular and fibrillar flexibilities of the α1-chains found in types I–III collagen. J Theor Biol. 2002;216(2):243–54. [Google Scholar] [PubMed]
5. Dunn MG, Silver FH. Viscoelastic behavior of human connective tissues: relative contribution of viscous and elastic components. Connect Tissue Res. 1983;12(1):59–70. doi:10.3109/03008208309005612. [Google Scholar] [PubMed] [CrossRef]
6. Sajid N, Convertino L, Friston K. Cancer niches and their Kikuchi free energy. Entropy. 2021;23(5):609. doi:10.3390/e23050609. [Google Scholar] [PubMed] [CrossRef]
7. Silver F, Landis W. Viscoelasticity, energy storage and transmission and dissipation by extracellular matrices in vertebrates. In: Fratzl P, editor. Collagen. Boston, MA: Springer; 2008. p. 133–54. doi:10.1007/978-0-387-73906-9_6 [Google Scholar] [CrossRef]
8. Sarvazyan A, Tatarinov A, Sarvazyan N. Ultrasonic assessment of tissue hydration status. Ultrasonics. 2005;43(8):661–71. [Google Scholar] [PubMed]
9. Sophia Fox AJ, Bedi A, Rodeo SA. The basic science of articular cartilage: structure, composition, and function. Sports Health. 2009;6(6):461–8. [Google Scholar]
10. Urcivoli E, Peruzzi B. Involvement of the FAK network in pathologies related to altered mechanotransduction. Int J Mol Sci. 2020;21(24):9426. doi:10.3390/ijms2124942610.1177/1941738109350438. [Google Scholar] [CrossRef]
11. Elgindi M, Sappudom J, Ibrahim IH, Al-Sayegh M, Chen W, Garcia-Sabate A, et al. May the force be with you (or notthe immune system under microgravity. Cells. 2021;10(8):1941. doi:10.3390/cells10081941. [Google Scholar] [PubMed] [CrossRef]
12. Kerneur C, Cano CE, Olive D. Major pathways involved in macrophage polarization in cancer. Front Immunol. 2022;3:1026954. doi:10.3389/fimmu.2022.1026954. [Google Scholar] [PubMed] [CrossRef]
13. Gonzalez H, Hagerling C, Werb Z. Roles of the immune system in cancer: from tumor initiation to metastatic progression. Genes Dev. 2018;32(19–20):1267–84. doi:10.1101/gad.314617.118. [Google Scholar] [PubMed] [CrossRef]
14. Silver FH, Shah RG, Silver LL. The use of vibrational optical coherence tomography in matching host tissue and implant mechanical properties. Biomed Med Appl. 2018;2(2). doi:10.4172/2577-0268.1000115. [Google Scholar] [CrossRef]
15. Silver FH, Siperko LM. Mechanosensing and mechanochemical transduction. Crit Rev Biomed Eng. 2003;31:255–331. [Google Scholar] [PubMed]
16. Silver FH. Mechanotransduction—the relationship between gravity, cells and tensile loading in extracellular matrix. BIOCELL. 2022;46(2):297–9. doi:10.32604/biocell.2022.017406. [Google Scholar] [CrossRef]
17. Silver FH, Deshmukh T, Ryan N, Romm A, Nadiminti H. “Fingerprinting” benign and cancerous skin lesions using vibrational optical coherence tomography: differentiation among cancerous lesion types based on the presence of new cells, blood vessels and fibrosis. Biomolecules. 2022;12:1332. doi:10.3390/biom12101332. [Google Scholar] [PubMed] [CrossRef]
18. Chmielewska A, Dean D. The role of stiffness-matching in avoiding stress-shielding-induced bone loss and stress concentration-induced skeletal reconstruction device failure. Acta Biomater. 2023;173:51–65. doi:10.1016/j.actbio.2023.11.11/011. [Google Scholar] [CrossRef]
19. Alexander RM. Elastic energy stores in running vertebrates. Zool. 1984;24:85–94. [Google Scholar]
20. Wilson AM, McGuigan MP, Su A, van den Bogert AJ. Horses damp the spring in their step. Nature. 2001;414:895–9. [Google Scholar] [PubMed]
21. Silver FH, Bradica G, Tria A. Do changes in the mechanical properties of articular cartilage promote catabolic destruction of cartilage and osteoarthritis? Matrix Biol. 2004;23(7):467–76. [Google Scholar] [PubMed]
22. Freeman JW, Silver FH. Elastic energy storage in unmineralized and mineralized extracellular matrices (ECMsa comparison between molecular modeling and experimental measurements. J Theor Biol. 2004;229(3):371–8. [Google Scholar] [PubMed]
23. Silver FH, Desmukh T, Benedetto D, Gonzalez-Mercedes M, Pulido J. Energy storage and dissipation in the eye: the importance of the biomechanical connections between the cornea-limbus-scleral series biomechanical element and the scleral-optic nerve-posterior segment tissues in protecting sensitive visual components from mechanical damage. Austin J Clin Ophthalmol. 2023;10(6):1162. [Google Scholar]
24. Horvath I, Foran DJ, Silver FH. Energy analysis of flow induced harmonic motion in blood vessel walls. Cardiovasc Eng. 2005;5(1):21–8. doi:10.1007/s10558-005-3070-z. [Google Scholar] [CrossRef]
25. Kubow K, Vukmirovic R, Zhe L, Klotzsch E, Smith ML, Gourdon D, et al. Mechanical forces regulate the interactions of fibronectin and collagen I in extracellular matrix. Nat Commun. 2015;6(1):8026. doi:10.1038/ncomms9026. [Google Scholar] [PubMed] [CrossRef]
26. Dalton CJ, Lemmon CA. Fibronectin: molecular structure, fibrillar structure and mechanochemical signaling. Cells. 2021;10(9):2443. doi:10.3390/cells10092443. [Google Scholar] [PubMed] [CrossRef]
27. Cargnello M, Roux PP. Activation and function of the MAPKs and their substrates, the MAPK-activated protein kinases. Microbiol Mol Biol Rev. 2011;75(1):50–83. doi:10.1128/MMBR.00031-10. [Google Scholar] [PubMed] [CrossRef]
28. Dupont S, Morsut L, Aragona M, Enzo E, Giulitti S, Cordenosi M, et al. Role of YAP/TAZ in mechanotransduction. Nature. 2011;474(7350):179–83. doi:10.1038/nature10137. [Google Scholar] [PubMed] [CrossRef]
29. Robert G, Jullian V, Jacquel A, Ginet C, Dufies M, Torino S, et al. Simalikalactone E (SkEa new weapon in the armamentarium of drugs targeting cancers that exhibit constitutive activation of the ERK pathway. Oncotarget. 2012;3(12):1688–99. doi:10.18632/oncotarget.791. [Google Scholar] [PubMed] [CrossRef]
30. Liu Z, Li S, Qian X, Li L, Zhang H, Liu Z. RhoA/ROCK-YAP/TAZ axis regulates the fibrotic activity in dexamethasone-treated human trabecular meshwork cells. Front Mol Biosci. 2021;8:728932. doi:10.3389/fmolb.2021.728932. [Google Scholar] [PubMed] [CrossRef]
31. Yang X, Dinehart MS. Triple Hedgehog pathway inhibition for basal cell carcinoma. J Clin Aesthet Dermatol. 2017;10(4):47–9. doi:10.25251/skin.1.supp.120. [Google Scholar] [CrossRef]
32. Du J, Zu Y, Li J, Du S, Xu Y, Zhang L, et al. Extracellular matrix stiffness dictates Wnt expression through integrin pathway. Sci Rep. 2016;8(6):20395. doi:10.1038/srep20395. [Google Scholar] [PubMed] [CrossRef]
33. Pai SG, Carneiro BA, Mota JM, Costa R, Leite CA, Barroso-Sousa R, et al. Wnt/β-catenin pathway: modulating anticancer immune response. J Hematol Oncol. 2017;10(1):101. doi:10.1186/s13045-017-0471-6. [Google Scholar] [PubMed] [CrossRef]
34. Lambert MW. The functional importance of lamins, actin, myosin, spectrin and the LINC complex in DNA repair. Exp Biol Med. 2019;244(15):1382–1406. doi:10.1177/1535370219876651. [Google Scholar] [PubMed] [CrossRef]
35. Bouzid T, Kim E, Riehl BD, Esfahani AM, Rosenbohm J, Yang R, et al. The LINC complex, mechanotransduction, and mesenchymal stem cell functionand fate. J Biol Eng. 2019;13(1):68. doi:10.1186/s13036-019-0197-9. [Google Scholar] [PubMed] [CrossRef]
36. Eberwein P, Laird D, Schulz S, Reinhard T, Steinberg T, Tomakidi P. Modulation of focal adhesion constituents and their down-stream events by EGF: on the cross-talk of integrins and growth factor receptors. Biochim Biophys Acta. 2015;1853(10):2183–98. doi:10.1016/j.bbamcr.2015.06.004. [Google Scholar] [PubMed] [CrossRef]
37. Sun Z, Guo SS, Fassler R. Integrin-mediated mechanotransduction. J Cell Biol. 2016;215(4):445–56. doi:10.1083/jcb.201609037. [Google Scholar] [PubMed] [CrossRef]
38. Winograd-Katz SE, Fässler R, Geiger B, Legate KR. The integrin adhesome: from genes and proteins to human disease. Nat Rev Mol Cell Biol. 2014;15(4):273–88. doi:10.1038/nrm3769. [Google Scholar] [PubMed] [CrossRef]
39. Zeltz C, Lu N, Gullberg D. Integrin α11β1: a major collagen receptor on fibroblastic cells. Adv Exp Med Biol. 2014;819:73–83. doi:10.1007/978-94-017-9153-3. [Google Scholar] [CrossRef]
40. Swaminathan V, Waterman CM. The molecular clutch model for mechanotransduction evolves. Nat Cell Biol. 2016;18(5):459–61. doi:10.1038/ncb3350. [Google Scholar] [PubMed] [CrossRef]
41. Schwartz MA. Integrins and extracellular matrix in mechanotransduction. Cold Spring Harb Perspect Biol. 2010;2(12):a005066. doi:10.1101/cshperspect.a005066. [Google Scholar] [PubMed] [CrossRef]
42. Elosegui-Artola A, Trepat X, Roca-Cusachs P. Control of mechanotransduction by molecular clutch dynamics. Trends in Cell Biol. 2018;28(5):358–67. doi:10.1016/j.tcb.2018.01.008. [Google Scholar] [PubMed] [CrossRef]
43. Mitchison T, Kirschner M. Cytoskeletal dynamics and nerve growth. Neuron. 1988;1(9):761–72. doi:10.1016/0896-6273(88)90124-9. [Google Scholar] [PubMed] [CrossRef]
44. Davison PM, Cadet B. Actin on and around the nucleus. Trends Cell Biol. 2021;31(3):211–23. doi:10.1016/j.tcb.2020.11.009. [Google Scholar] [PubMed] [CrossRef]
45. Cobb MH. MAP kinase pathways. Prog in Biophys Mol Biol. 1999;71:479–500. [Google Scholar]
46. Goult BT, Brown NH, Schwartz MA. Talin in mechanotransduction and mechanomemoryat a glance. J Cell Sci. 2021;134(20):jcs258749. doi:10.1242/jcs.258749. [Google Scholar] [PubMed] [CrossRef]
47. Ishida T, Peterson TE, Kovach NL, Berk BC. MAP kinase activation by flow in endothelial cells: role of β1 integrins and tyrosine kinases. Cir Res. 1996;79(2):310–6. doi:10.1161/01.RES.79.2.310. [Google Scholar] [PubMed] [CrossRef]
48. Song X, Xu H, Wang P, Wang J, Affo S, Wang H, et al. Focal adhesion kinase (FAK) promotes cholangiocarcinoma development and progression via YAP activation. J Hep. 2021;75(4):888–99. doi:10.1016/j.jhep.2021.05.018. [Google Scholar] [PubMed] [CrossRef]
49. Xu Z, Orkwis JA, Harris GM. Cell shape and matrix stiffness impact Schwann cell plasticity via YAP/TAZ and rho GTPases. Int J Mol Sci. 2021;22(9):4821. doi:10.3390/ijms22094821. [Google Scholar] [PubMed] [CrossRef]
50. Carballo GB, Honorato JR, Farias de Lopes GP, de Sampaio E, Spohr CL. A highlight on Sonic Hedgehog pathway. Cell Comm Sig. 2018;16(1):11. doi:10.1186/s12964-018-0220-7. [Google Scholar] [PubMed] [CrossRef]
51. Nygren MK, Dosen G, Stubberud H, Walchle S, Munthe E, Rian E. β-catenin is involved in N-cadherin–dependent adhesion, but not in canonical Wnt signaling in E2A-PBX1–positive B acute lymphoblastic leukemia cells. Exp Hem. 2009;37(2):151–308. doi:10.1016/j.exphem.2008.10.007. [Google Scholar] [PubMed] [CrossRef]
52. Burandt E, Lübbersmeyer F, Gorbokon N, Büscheck F, Luebke AM, Menz A, et al. E-Cadherin expression in human tumors: a tissue microarray study on 10,851 tumors. Biomark Res. 2021;9(1):44. doi:10.1186/s40364-021-00299-4. [Google Scholar] [PubMed] [CrossRef]
53. Liu J, Xiao Q, Xiao J, Niu C, Li Y, Zhang X, et al. Wnt/β-catenin signalling: function, biological mechanisms, and therapeutic opportunities. Signal Transduct Target Ther. 2022;7(1):3. doi:10.1038/s41392-021-00762-6. [Google Scholar] [PubMed] [CrossRef]
54. Zhan T, Rindtoff N, Boutros M. Wnt signaling in cancer. Oncogen. 2017;36:1461–73. [Google Scholar] [PubMed]
55. Coste B, Mathur J, Schmidt M, Earley TJ, Ranade S, Petrus MJ, et al. Piezo1 and Piezo2 are essential components of distinct mechanically activated cation channels. Science. 2010;330(6000):55–60. doi:10.1126/science.1193270. [Google Scholar] [PubMed] [CrossRef]
56. Coste B, Xiao B, Santos JS, Syeda R, Grandl, Spencer KS, et al. Piezo proteins are pore-forming subunits of mechanicallyactivated channels. Nature. 2012;483(7388):176–81. [Google Scholar] [PubMed]
57. Bagriantsev SN, Gracheva EO, Gallagher PG. Piezo proteins: regulators of mechanosensation and other cellular processes. J Biol Chem. 2014;289(46):31673–81. doi:10.1074/jbc.R114.612697. [Google Scholar] [PubMed] [CrossRef]
58. Kefauver JM, Ward AB, Patapoutian A. Discoveries in structure and physiology of mechanically activated ion channels. Nature. 2020;587(7835):567–76. doi:10.1038/s41586-020-2933-1. [Google Scholar] [PubMed] [CrossRef]
59. Ge J, Li W, Zhao Q, Li N, Chen M, Zi P, et al. Architecture of the mammalian mechanosensitive Piezo1 channel. Nature. 2015;527(7576):64–9. doi:10.1038/nature15247. [Google Scholar] [PubMed] [CrossRef]
60. Syeda R, Xu J, Dubin AE, Coste B, Mathur J, Huynh T, et al. Chemical activation of the mechanotransduction channel Piezo1. eLife. 2015;4:e07369. doi:10.7554/eLife.07369. [Google Scholar] [PubMed] [CrossRef]
61. Christensen AP, Corey DP. TRP channels in mechanosensation: direct or indirect activation? Nat Rev Neurosci. 2007;8(7):510–21. doi:10.1038/nrn2149. [Google Scholar] [PubMed] [CrossRef]
62. Deng Z, Paknejad N, Maksaev G, Sala-Rabanal M, Nichols CG, Hite RK, et al. Cryo-EM and X-ray structures of TRPV4 reveal insight into ion permeation and gating mechanisms. Nat Struct Mol Biol. 2018;25(3):252–60. doi:10.1038/s41594-018-0037-5. [Google Scholar] [PubMed] [CrossRef]
63. Sullivan B, Light T, Vu V, Kapustka A, Hristova K, Leckband D. Mechanical disruption of E-cadherin complexes with epidermal growth factor receptor actuates growth factor-dependent signaling. Proc Natl Acad Sci U S A. 2022;119(4):e2100679119. doi:10.1073/pnas.2100679119. [Google Scholar] [PubMed] [CrossRef]
64. Kim JH, Asthagiri AR. Matrix stiffening sensitizes epithelial cells to EGF and enables the loss of contact inhibition of proliferation. J Cell Sci. 2011;124(8):1280–7. doi:10.1242/jcs.078394. [Google Scholar] [PubMed] [CrossRef]
65. Sarker H, Hardy E, Haimour A, Karim MA, Scholl-Bürgi S, Martignetti JA, et al. Comparative serum analyses identify cytokines and hormones commonly dysregulated as well as implicated in promoting osteolysis in MMP-2-deficient mice and children. Front Physiol. 2020;25(11):568718. doi:10.3389/fphys.2020.568718. [Google Scholar] [PubMed] [CrossRef]
66. Northey JJ, Weaver VM. Mechanosensitive steroid hormone signaling and cell fate. Endocr. 2022;163(8):bqac085. doi:10.1210/endocr/bqac085. [Google Scholar] [PubMed] [CrossRef]
67. Guan G, Cannon RD, Coates DE, Mei L. Effect of the rho-kinase/ROCK signaling pathway on cytoskeleton components. Genes. 2023;14(2):272. doi:10.3390/genes14020272. [Google Scholar] [PubMed] [CrossRef]
68. Miura M, Hataa Y, Hirayamaa K, Kita T, Nodaa Y, Fujisawaa K, et al. Critical role of the Rho-kinase pathway in TGF-β2-dependent collagen gel contraction by retinal pigment epithelial cells. Exper Eye Res. 2006;82(5):849–59. [Google Scholar]
69. Prasad B, Grimm D, Strauch SM, Erzinger GS, Corydon TJ, Lebert M, et al. Influence of microgravity on apoptosis in cells, tissues, and other systems in vivo and in vitro. Int J Mol Sci. 2020;21(24):9373. doi:10.3390/ijms21249373. [Google Scholar] [PubMed] [CrossRef]
70. Li P, Shi J, Zhang P, Wang K, Li J, Liu H, et al. Simulated microgravity disrupts intestinal homeostasis and increases colitis susceptibility. FASEB J. 2015;29(8):3263–73. [Google Scholar] [PubMed]
71. Vinken M. Hepatology in space: effects of spaceflight and simulated microgravity on the liver. Liver Int. 2022;42(12):2599–606. doi:10.1111/liv.15444. [Google Scholar] [PubMed] [CrossRef]
72. Prado GR, LaPlaca MC. Neuronal plasma membrane integrity is transiently disturbed by traumatic loading. Neurosci Insights. 2020;27(15). doi:10.1177/2633105520946090. [Google Scholar] [PubMed] [CrossRef]
73. Hagan ML, Balayan V, McGee-Lawrence ME. Plasma membrane disruption (PMD) formation and repair in mechanosensitive tissues. Bone. 2021;149:115970. doi:10.1016/j.bone.2021.115970. [Google Scholar] [PubMed] [CrossRef]
74. Grembowicz KP, Sprague D, McNeil PL. Temporary disruption of the plasma membrane is required for c-fos expression in response to mechanical stress. Mol Biol Cell. 1999;10(4):1247–57. doi:10.1091/mbc.10.4.1247. [Google Scholar] [PubMed] [CrossRef]
75. Alessandra G, Algerta M, Paola M, Carsten S, Cristina L, Palo M, et al. Shaping pancreatic β-cell differentiation and functioning: the influence of mechanotransduction. Cells. 2020;9(2):413. doi:10.3390/cells902413. [Google Scholar] [CrossRef]
76. Jiang WJ, Peng YC, Yang KM. Cellular signaling pathways regulating beta-cell proliferation as a promising therapeutic target in the treatment of diabetes. Exp Ther Med. 2018;16(4):3275–85. doi:10.3892/etm.2018.6603. [Google Scholar] [PubMed] [CrossRef]
77. Chehaitly A, Vessieres E, Guihot AL, Henrion D. Flow-mediated outward arterial remodeling in aging. Mech Ageing Dev. 2021;194:111416. doi:10.1016/j.mad.2020.111416. [Google Scholar] [PubMed] [CrossRef]
78. Ye GJC, Nesmith AP, Parker KK. The role of mechanotransduction on vascular smooth muscle myocytes cytoskeleton and contractile function. Anat Rec. 2014;297(9):1758–69. doi:10.1002/ar.22983. [Google Scholar] [PubMed] [CrossRef]
79. Muscedere JG, Mullen JB, Gan K, Slutsky AS. Tidal ventilation at low airway pressures can augment lung injury. Am J Respir Crit Care Med. 1994;149(5):1327–34. doi:10.1164/ajrccm.149.5.8173774. [Google Scholar] [PubMed] [CrossRef]
80. Chatterlee S, Nieman GF, Christie JD, Fischer AB. Shear stress-related mechanosignaling with lung ischemia: lessons from basic research can inform lung transplantation. Am J Physiol Lung Cell Mol Physiol. 2014;307(9):L668–80. [Google Scholar] [PubMed]
81. Beitler JR, Sands SA, Loring SH, Owens RL, Malhotra A, Spragg RG, et al. Quantifying unintended exposure to high tidal volumes from breath stacking dyssynchrony in ARDS: the BREATHE criteria. Intensive Care Med. 2016;42(9):1427–36. doi:10.1007/s00134-016-4423-3. [Google Scholar] [PubMed] [CrossRef]
82. Chin L, Xia Y, Discher DE, Janmay PA. Mechanotransduction in cancer. Curr Opin Chem Eng. 2016;11:77–84. doi:10.1016/j.coche.2016.01.011. [Google Scholar] [PubMed] [CrossRef]
83. Mukherjee AG, Wanjari UR, Namachivayam A, Murali R, Prabakaran DS, Ganesan R, et al. Role of immune cells in cancer treatment. Vaccines. 2022;10(9):1493. doi:10.3390/vaccines10091493. [Google Scholar] [PubMed] [CrossRef]
84. Ji Q, Zhang L, Liu X, Zhou L, Wang W, Han Z, et al. Long non-coding RNA MALAT1 promotes tumour growth and metastasis in colorectal cancer through binding to SFPQ and releasing oncogene PTBP2 from SFPQ/PTBP2 complex. Br J Cancer. 2014;111(4):736–48. doi:10.1038/bjc.2014.383. [Google Scholar] [PubMed] [CrossRef]
85. McGrail DJ, Kieu QM, Dawson MR. The malignancy of metastatic ovarian cancer cells is increased on soft matrices through a mechanosensitive Rho-ROCK pathway. J Cell Sci. 2014;127(12):2621–6. doi:10.1242/jcs.144378. [Google Scholar] [PubMed] [CrossRef]
86. Levental KR, Yu H, Kass L, Lakins JN, Egeblad M, Erler JT, et al. Matrix crosslinking forces tumor progression by enhancing integrin signaling. Cell. 2009;139(5):891–906. doi:10.1016/j.cell.2009.10.027. [Google Scholar] [PubMed] [CrossRef]
87. Tang X, Kuhlenschmidt TB, Li Q, Ali S, Lezmi S, Chen H, et al. A mechanically-induced colon cancer cell population shows increased metastatic potential. Mol Cancer. 2014;13(1):131. doi:10.1186/1476-4598-13-131. [Google Scholar] [PubMed] [CrossRef]
Cite This Article
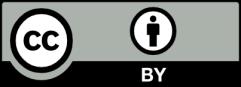