Open Access
ARTICLE
Bio-Based Rigid Polyurethane Foams for Cryogenic Insulation
Latvian State Institute of Wood Chemistry, Riga, LV-1006, Latvia
* Corresponding Author: Laima Vevere. Email:
Journal of Renewable Materials 2024, 12(3), 585-602. https://doi.org/10.32604/jrm.2024.047350
Received 03 November 2023; Accepted 25 January 2024; Issue published 11 April 2024
Abstract
Cryogenic insulation material rigid polyurethane (PU) foams were developed using bio-based and recycled feedstock. Polyols obtained from tall oil fatty acids produced as a side stream of wood biomass pulping and recycled polyethylene terephthalate were used to develop rigid PU foam formulations. The 4th generation physical blowing agents with low global warming potential and low ozone depletion potential were used to develop rigid PU foam cryogenic insulation with excellent mechanical and thermal properties. Obtained rigid PU foams had a thermal conductivity coefficient as low as 0.0171 W/m·K and an apparent density of 37–40 kg/m3. The developed rigid PU foams had anisotropic compression strength properties, which were higher parallel to the foaming direction. Moreover, the compression strength was also influenced by the type of applied bio-based polyol. The bio-based polyols with higher OH group functionality delivered higher crosslinking density of polymer matrix; thus, the mechanical properties were also higher. The mechanical strength of the foams increased when materials were tested at liquid nitrogen temperature due to the stiffening of the polymer matrix. The thermal properties of the developed materials were determined using differential scanning calorimetry, dynamic mechanical analysis, and thermogravimetric analysis methods. Lastly, the developed rigid PU foams had good adhesion to the aluminium substrate before and after applying cyroshock and an excellent safety coefficient of 4–5. Rigid PU foams developed using Solstice LBA delivered adhesion strength of ~0.5 MPa and may be considered for application as cryogenic insulation in the aerospace industry.Graphical Abstract
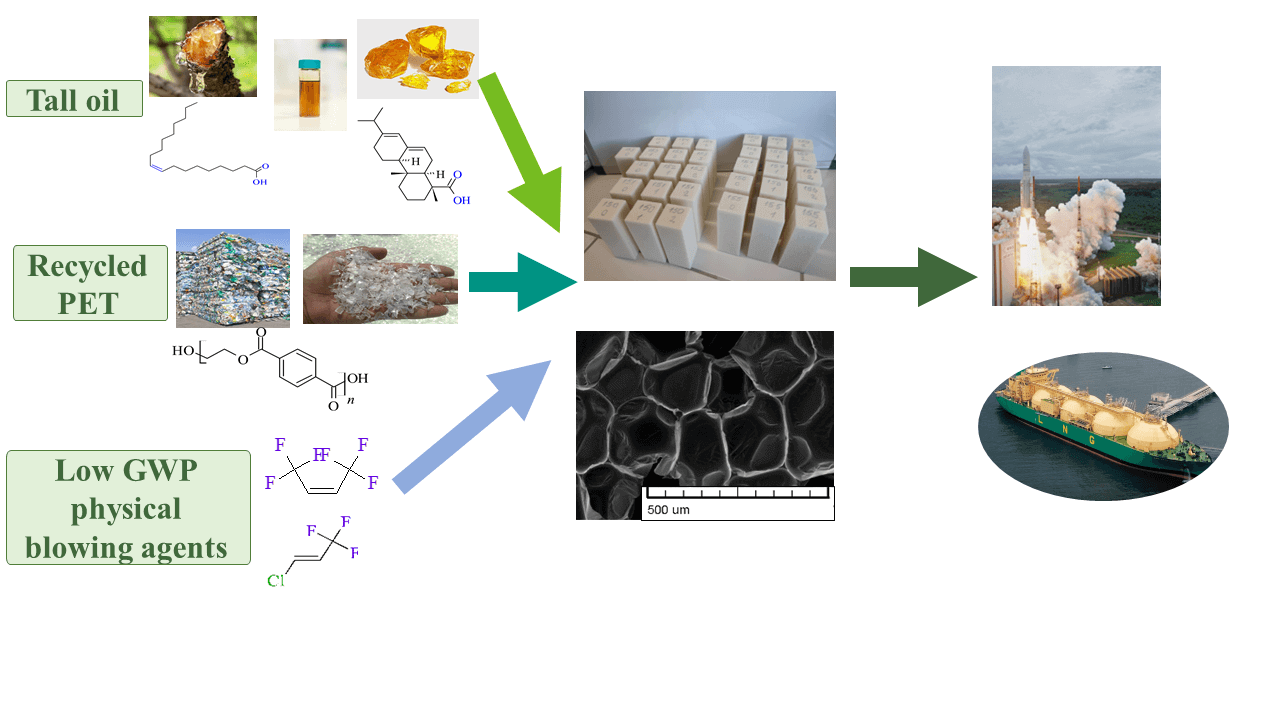
Keywords
Nomenclature
DEG | Diethylene glycol |
DMA | Dynamic mechanical analysis |
DSC | Differential scanning calorimetry |
DEOA | Diethanolamine |
E’ | Storage modulus |
ETOFA | Epoxidized tall oil fatty acids |
ETOFA–DEG | ETOFA and diethylene glycol polyol |
ETOFA–DEOA | ETOFA and diethanolamine polyol |
ETOFA–TEOA | ETOFA and triethanolamine polyol |
ETOFA–TMP | ETOFA and trimethylolpropane polyol |
HFOs | Hydrofluoroolefins |
GWP | Global warming potential |
Mc | Molecular weight between the branching unit |
LBA | Liquid blowing agent |
NEO240 | Trade name of aromatic polyester polyol from NEO Group |
PET | Polyethylene terephthalate |
PU | Polyurethane |
pMDI | Polymeric diphenylmethane diisocyanate |
SEM | Scanning electron microscopy |
TCPP | Tris(2-chloroisopropyl) phosphate |
TEOA | Triethanolamine |
TMP | Trimethylolpropane |
TGA | Thermogravimetric analysis |
TMA | Thermomechanical analysis |
ε77 | Tensile elongation at break at 77 K, % |
Δl295-77 | Shrinkage of material cooling it from 295 to 77 K, % |
Cryogenic insulation is a necessary and effective tool to prevent undesired heat gain, save energy and prevent the liquefication or solidification of gases at these ultra-low temperatures. It is used starting from cold storage units and liquefied natural gas carriers up to insulation for space launchers’ cryogenic propellant tanks. Selecting the suitable insulation material for cryogenic application might be challenging as excellent insulators at room temperature may not perform as well at cryogenic temperatures. Cryogenic insulation must withstand mechanical stresses without degrading or losing their insulating properties. Aerogels [1–3], rigid polyurethane foams [4,5], on their own, as well as multilayer insulation [6–9] are being explored to provide good thermal resistance while minimizing the thickness and weight of insulation systems. Rigid polyurethane (PU) foams are one of the best materials for cryogenic insulation due to their light weight, good mechanical properties, and low thermal conductivity. They are also lightweight and have high mechanical qualities. Two factors stand out among the rest for the resilience of rigid PU foam insulation at cryogenic temperatures. First, the high adhesive strength of the foam to the base material (aluminium, steel, composites, etc.), which remains intact even at cryogenic temperatures. Second, materials often have different coefficients of thermal expansion. As a result of this, during cooling, cracks often appear. PU foams can withstand the formation of those cracks.
As with many industries, there is a growing emphasis on sustainability. Studies of rigid PU foams from bio-based feedstock are growing each year. Polyols for rigid PU foams have been made from lignin [10–12], rapeseed oil [13–15], soybean oil [16,17], palm oil [18–20], tall oil [21,22], tannins [23,24], and cashew nutshell [25], among other biomass. First-generation feedstock such as rapeseed oil, palm, or soybean oil is not favorable for this purpose due to competition with food and feed production. Second-generation feedstock, such as tall oil, is more favorable as it is produced as a side stream of wood biomass pulping. One way to obtain polyols from tall oil fatty acids is epoxidation following a ring-opening reaction [26]. The Polymer Laboratory of Latvian State Institute of Wood Chemistry has done extensive research on tall oil and tall oil fatty acids epoxidation [22,27]. In this research, we used four polyols from epoxidized tall oil fatty acids (ETOFA). The scheme showing the production of polyols used in this study is shown in Fig. 1.
Figure 1: Idealized scheme of polyol synthesis from ETOFA [27]
Second way how to increase sustainably in rigid PU foams is used polyols from recycled materials. Polyols from recycled polyethylene terephthalate (PET) are one of those options. There are several ways how to recycle PET chemically–alcoholysis, hydrolysis, glycolysis, ammonolysis, and aminolysis [28]. Polyols for polyurethanes are mainly produced using glycolysis and aminolysis (Fig. 2).
Figure 2: Chemical depolymerization of PET
Another essential component of rigid PU foams is the blowing agent. Many physical blowing agents have a negative effect on global warming and ozone layer depletion. The global market was recently introduced with 4th-generation blowing agents–hydrofluoroolefins (HFOs)–with low ozone depletion potential and global warming potential (GWP) due to regulatory pressure [29]. The two most commonly used are trans-1-chloro-3,3,3-trifluoropropene (one of the trade names–Solstice LBA) and cis-1,1,1,4,4,4-hexafluoro-2-butene (one of the trade names Opteon 1100). Structure formulas of both physical blowing agents are showed in Fig. 3. Both blowing agents’ ozone depletion potential is 0, and GWP values for Solstice LBA are 1 and for Opteon 1100 is 2 [30,31]. Both are suitable for rigid PU foam production as the current restriction for future F-gas use limits it to substances with GWP < 11 [29].
Figure 3: Structure formulas of Solstice LBA (A) and Opteon 1100 (B)
Although there is great emphasis on using bio-based polyols and 4th-generation blowing agents, there are very limited studies using those in cryogenic insulation. Uram et al. and Sture et al. have used epoxidized tall oil and epoxidized rapeseed oil in cryogenic insulation, but both of them used blend of 1,1,1,3,3-pentafluorobutane and 1,1,1,2,3,3,3-heptafluoropropane (trade name Solkane 365/227) as blowing agent [21,32] which is phased out starting of January 01, 2023 [29]. Yakushin et al. used Solstice LBA in cryogenic PU foams but used commercially available petroleum-based polyols [33]. It is vital to demonstrate that the next-generation physical blowing agents are compatible with bio-based polyols and that developing good-quality cryogenic insulation material is possible.
This is continuation of our previous work on cryogenic insulation–cryogenic insolation from commercially available polyols with Solstice LBA as blowing agent [33] and cryogenic insulation from epoxidized rapeseed oil and tall oil fatty acids with and without nanocellulose and Solkane 365/227 as blowing agent [21,32]. Our previous research allowed to optimize catalysts, checked bio-polyol suitability for cryogenic rigid PU foams, as well as introduce 4th-generation generation blowing agent Solstice LBA in cryogenic rigid PU foams. This is more extended research, that focuses on mechanical and thermal properties of rigid PU foams produced using polyols from epoxidized tall oil previously developed by the Polymer Laboratory of Latvian State Institute of Wood Chemistry [27] and polyols from recycled polyethylene terephthalate (PET) and 4th-generation generation blowing agents with low global warming potential–Opteon 1100 and Solstice LBA. Obtained results show that it is possible to produce excellent cryogenic thermal insulation material from sustainable feedstock with the next generation physical blowing agents.
For rigid PU foams, the following materials were used: polyols from epoxidized tall oil fatty acids ETOFA-DEG, ETOFA-DEOA, ETOFA-TEOA, and ETOFA-TMP polyols were synthesized in Latvian State Institute of Wood Chemistry, NEO 240 (Neo Group, Lithuania), diethylene glycol (Chempur, Germany), flame retardant tris(2-chloroisopropyl)phosphate (TCPP) (Albermarle, Belgium), blowing agents Opteon 1100 (The Chemours Company FC, Netherlands), Solstice LBA (Honeywell Fluorine Products Europe B.V., Weert, The Netherlands), catalysts Polycat®203 (Evonik, Germany), Polycat®218 (Evonik, Germany), Dabco®MB20 (Evonik, Germany), surfactant Tegostab® B 84711 Evonik, Germany), polymeric 4,4-methylene diphenyl isocyanate (pMDI) (Desmodur® 44V20L from Covestro AG, Lervekusen, Germany). In total, 30 different rigid PU foams were tested. Formulations of rigid PU foams are shown in Tables 1–3. The crosslinking of the developed rigid PU foam polymer matrix was characterized by molecular weight between the branching unit–Mc. The Mc of the rigid PU foam polymer matrix was calculated using the number average functionality and molecular weight of the reagents used [34]. Both parameters were determined by the GPC/SEC chromatography, and it was assumed that the full conversion of all reacting groups had occurred [27]. The Mc values of the developed rigid PU foams are summarized in Tables 1–3.
2.2 Preparation of Rigid PU Foams
Rigid PU foams were obtained using pouring method at room temperature (~22°C). Polyols, catalysts, surfactant, flame retardant and blowing agents in necessary amounts were mixed together for a minute, then isocyanate in necessary amount were added and mixed for 10 s. Everything was poured into open top mold. Foam was left at room temperature for 24 h. After 24 h obtained foam was released from mold and cut into necessary samples.
The apparent density of the obtained PU foams was tested according to the ISO 845:2006 standard.
2.4 Coefficient of Thermal Conductivity
The Linseis Heat flow meter 200 was used for determining the coefficient of thermal conductivity. The test was carried out in compliance with ISO 8301:1991. A 200 × 200 × 50 mm sample was placed between two plates, the top plate at 20°C and the bottom plate at 0°C in temperature. The thermal conductivity coefficient was determined at mean value +10°C.
In keeping with EN ISO 844:2014, compression tests were conducted at room temperature with a Zwick/Roell Z010 (10 kN) static materials testing device coupled with a 1 kN force cell. For compression tests, cylindrical samples with a 20 mm diameter and a 22 mm height have been used. The two directions in which the tests were carried out were perpendicular (X) and parallel (Z) to the foam rise. A total of dozen samples were used, six in each direction. Characteristics were ascertained with a compressive rate of 10%/min and a preload of 1 kN.
In keeping with EN ISO 844:2014, compression tests were conducted out at liquid nitrogen temperature with a Zwick/Roell Z100 (100 kN) + 1 kN force cell with a cryostat. Tests on cylinders with dimensions ranging from 20 mm in diameter and 22 mm in height were conducted.
Tensile strength tests at room temperature were conducted following the ISO 1926:2005 standard, utilizing a Zwick/Roell Z010 (10 kN) static materials testing machine with an additional 1 kN force cell. Dumbbell-type samples (length: 153 mm, thickness: 12 mm, width: 40 mm, bottleneck: 26 mm) were employed for the tests, totaling six samples, and assessments were executed in a parallel direction.
For Young’s modulus and tensile strength at liquid nitrogen temperature, testing was carried out in accordance with ISO 1926:2005 standard using the Zwick/Roell Z100 (100 kN) + 1 kN force cell equipped with a cryostat. Ring-type samples (width: 13 mm, inner diameter: 43 mm, outer diameter: 53 mm) were utilized, and six samples were tested in parallel. The samples were placed in a specially adjusted cryogenic equipment, as depicted in Fig. 4. The rings were cut out in-plane perpendicular to the foam rise direction, and the reliability of this testing method, akin to ASTM D 2290, is detailed in [33].
Figure 4: Appliance for tensile tests at cryogenic temperature
The adhesion of rigid PU foam to an aluminium plate was evaluated through tensile strength measurements. Testing was conducted following the EN 1607:2013 standard, utilizing the Zwick/Roell Z010 (10 kN) static materials testing device coupled with a 1 kN force cell. The foam material and aluminium plates, each with a total thickness of 20 mm, were bonded using PU adhesive between two plates. A total of 16 samples were tested, consisting of 8 without immersion in liquid nitrogen and 8 subjected to immersion in liquid nitrogen. Adhesion strength measurements were taken both before and after cryo-shock to assess the impact of extreme temperature conditions on the adhesion properties of the foam-aluminium bond.
2.8 Thermogravimetric Analysis
Discovery TGA instruments was used to perform thermogravimetric analysis (TGA). Samples before testing were grounded-up in cryogenic ball mill. Data was processed using the OriginPro 2021 9.8.0.200 and TA Instruments TRIOS version #5.0.0.44608 software. Rigid PU foam samples were placed on platinum scale pans and heated in a nitrogen atmosphere at 10°C/min, between 30°C–700°C.
2.9 Differential Scanning Calorimetry
Mettler Toledo DSC 823e was used for the differential scanning calorimetry (DSC). Samples before testing were grounded-up in cryogenic ball mill. The tested samples weighed about 5 mg; aluminium crucibles were used for the test. The rigid PU foam sample was heated from 25°C to 180°C (10°C/min), cooled from 180°C to −100°C (10°C/min), and heated back to 180°C (10°C/min).
2.10 Dynamic Mechanical Analysis
Mettler Toledo DMA/SDTA861e was used for dynamic mechanical analysis (DMA). Cylindrical samples with a 13 mm diameter and a 9 mm height have been used. Samples were tested in compression oscillation mode in temperature range from −100°C to 180°C (heating/cooling rate 3°C/min), frequency was 1 Hz, amplitude–30 μm, and maximal force–5 N.
2.11 Thermomechanical Analysis and Safety Coefficient
Thermomechanical analysis (TMA) tests were conducted with a Linseis TMA PT instrument from Linseis in Selb, Germany. Rectangular cuboid samples with a height (h) of approximately 2 cm were prepared for analysis. The testing involved cooling each sample from 20°C to −160°C at a rate of 3°C/min, followed by heating to 50°C (3°C/min). Material shrinkage was determined based on TMA results obtained during the cooling phase, spanning from 22°C (295 K) to −196°C (77 K).
The ability of a material to tolerate thermal strains is indicated by its safety coefficient. Eq. (1) is used for its calculation.
where: ε77-tensile elongation at break at 77 K, %
Δl295-77-shrinkage of material cooling it from 295 to 77 K, %
2.12 Scanning Electron Microscopy (SEM)
Rigid PU foam samples were analyzed by obtaining images with scanning electron microscopy (SEM) using an Edax Tescan 5136 MM microscope.
Rigid PU foam systems were tailored to have a foam apparent density of 37–40 kg/m3 to ensure the balance between lightweight and good mechanical properties. Obtained rigid PU foams have closed cell structure, and cells in foam samples were elongated in the foam rise direction (Fig. 5). The cell elongation with the direction of the foaming was consistent throughout all of the foam samples. Different polyols or blowing agents used to develop rigid PU foams did not change it. The cell elongation with the foam rise direction is typical for rigid PU foam and is mainly influenced by the foam preparation method and foaming kinetics. Open-top mold typically produce elongated cell as foams has one direction to rise and faster growing foams also produce more elongated cells than slower growing foams. It is important to access the cell morphology as it will significantly affect the mechanical properties of the developed thermal insulation material.
Figure 5: SEM images of rigid PU foam perpendicular (A) and parallel (B) to the foaming direction
Obtained rigid PU foams have a coefficient of thermal conductivity in the range between 0.0171–0.0211 W/m·K (Fig. 6). This result is similar to or lower than other bio-based rigid PU foams−0.0187–0.0275 W/m·K [32], 0.029–0.031 W/m·K [35], and 0.025 W/m·K [36]. Rigid PU foams containing ETOFA-DEOA polyol had a higher coefficient of thermal conductivity than rigid PU foams containing ETOFA-TEOA polyol. This trend persists regardless of the selected physical blowing agent and a second bio-based polyol selected for the rigid PU foam development. This could be explained by higher functionality of ETOFA-TEOA polyol (fn = 7.9) than ETOFA-DEOA polyol (fn = 5.0) [27]. Higher functionality leads to higher amount of used pMDI in the preparation of rigid PU foams to maintain the same isocyanate index of 110. Higher pMDI content leads to higher aromatic group content, furthermore the higher functionality of ETOFA-TEOA polyol could also contribute to lower coefficient of thermal conductivity due to higher crosslinking of the polymer matrix [37]. Bio-based polyol content (both ETOFA-TMP and ETOFA-DEG) did not affect the coefficient of thermal conductivity. The physical blowing agent had the most significant influence on the coefficient of thermal conductivity–rigid PU foams containing Solstice LBA had a lower coefficient of thermal conductivity (0.0171–0.0179 W/m·K) than rigid PU foams containing Opteon 1100 (0.0185–0.0211 W/m·K). This could be explained by the lower vapor thermal conductivity of Solstice LBA (0.0102 W/m·K) [31] than Opteon 1100 (0.0107 W/m·K) [38].
Figure 6: Coefficient of thermal conductivity of rigid PU foams: TMP-Opteon series (A), DEG-Opteon series (B) and DEG-Solstice series (C)
Compressive strength, a crucial technical factor for any functional material, describes the strength limitations of material to direct static force loads anticipated during the construction’s ground and flight stages. Compressive strength measurements must be made in both foam-rise (Z) and perpendicular to foam-rise (X) directions to adequately characterize the anisotropic foam material (Figs. 7–9). The compression strength of developed rigid PU foams in parallel to the foaming direction was 0.11–0.18 MPa, and perpendicular to the foaming direction was 0.12–0.24 MPa. Those results are similar to other bio-based rigid PU foams as other authors report compression strength 0.09–0.18 MPa parallel to the foaming direction and 0.19–0.24 MPa perpendicular to the foaming direction [32,36,39]. Compression strength was higher perpendicular to the foaming direction than parallel to the foaming direction for all tested rigid PU foams. This correlates with SEM results as cells in foam samples were elongated in the foam rise direction ensuring higher durability. This is typical for rigid PU foams obtained at laboratory scale by producing foam samples in an open-top mold. Compression strength is higher at cryogenic temperature than at room temperature because the material becomes more rigid as it cools down due to the lack of macromolecular mobility. There is no significant difference in compression strength for ETOFA-DEOA and ETOFA-TEOA polyol usage in rigid PU foams. Rigid PU foams containing ETOFA-TMP polyols tend to have slightly higher compression strength than rigid PU foams containing ETOFA-DEG polyol, especially evident at cryogenic temperature (see Figs. 7 and 8). That might be explained by the higher functionality of ETOFA-TMP polyol [27], which could have resulted in a higher crosslinking density of rigid PU foams. The rigid PU foams with 40 pbw of ETOFA-TMP polyol had Mc value of 449 g/mol, whereas rigid PU foams without ETOFA-TMP polyol had Mc value of 585 g/mol. The lower the Mc value, the higher the crosslinking density of the PU polymer matrix. The rigid PU foams described in Figs. 8 and 9 had relatively similar Mc of ~583 g/mol, thus the compression properties are also similar between the samples in the series. The slightly lower compression strength properties of Solstice LBA series (0.19 MPa for Solstice LBA to 0.21 MPa for Opteon 1100 at room temperature) rigid PU foams could be explained by the plasticizing effect of this physical blowing agent.
Figure 7: Compression strength of rigid PU foams containing ETOFA-TMP polyol and Opteon 1100 at room and cryogenic temperatures
Figure 8: Compression strength of rigid PU foams containing ETOFA-DEG polyol and Opteon 1100 at room and cryogenic temperatures
Figure 9: Compression strength of rigid PU foams containing ETOFA-DEG polyol and Solstice LBA at room and cryogenic temperatures
Tensile strength is another technical factor for any functional material. Although it is not typically determined for rigid PU foams, it is crucial for cryogenic insulation (Fig. 10). It is necessary to determine rigid PU foam tensile strength at room temperature and at cryogenic temperature to see the material’s toughness. The foam material experiences inner stresses derived from thermal expansion/shrinkage, which may damage the core of the foam, or they may delaminate the foam material from the substrate surface. The same trends were observed for tensile strength as for compression-tensile strength is higher in cryogenic temperature than at room temperature due to materials’ hardening while cooling down; there is no significant difference in tensile strength for ETOFA-DEOA and ETOFA-TEOA polyol usage in rigid PU foams. Trend that rigid PU foams containing ETOFA-TMP polyols have slightly higher tensile strength than rigid PU foams containing ETOFA-DEG polyol is less pronounced as it is for compression. All developed bio-based rigid PU foams had a tensile strength of 0.4 MPa at room temperature and 0.6 MPa at cryogenic temperature.
Figure 10: Tensile strength of rigid PU foams: TMP-Opteon series (A), DEG-Opteon series (B) and DEG-Solstice series (C)
Adhesion strength measures how well the material bonds or adheres to another surface-in our case, rigid PU adhesion strength to the aluminium was determined. All rigid PU foams in this research break of between rigid PU foam and aluminium plate. Rigid PU foams containing ETOFA-TEOA polyol had lower adhesion strength than rigid PU foams containing ETOFA-DEOA polyol (Fig. 11). Also, rigid PU foam series containing ETOFA-TMP polyol had higher adhesion strength than rigid PU foam series containing ETOFA-DEG polyol. Rigid PU foams containing ETOFA-TMP consistently had better mechanical properties through mechanical testing. That can indicate more crosslinked structures in PU foams containing ETOFA-TMP polyol. The most significant influence on adhesion strength was the chosen physical blowing agent in the formulation. Although tensile and compression strength is higher, the adhesion strength of rigid PU foams containing Solstice LBA is 2–3 times higher than rigid PU foams containing Opteon 1100. One possible explanation for this could be that Solstice LBA absorbs less heat per mass unit than Opteon 1100 [40]. This allows to rigid PU foams containing Solstice LBA form stronger bonds with aluminium surface.
Figure 11: Adhesion strength of rigid PU foams: TMP-Opteon series (A), DEG-Opteon series (B) and DEG-Solstice series (C)
The safety coefficient is the ratio of two parameters-tensile elongation at break and shrinkage of material when cooling it from 295 to 77 K and shows the material’s ability to withstand thermal strains and mechanical stress. A higher safety coefficient means a better material’s chance to withstand thermal strains. As the mechanical properties of rigid PU foams containing Opteon 1100 are higher than those containing Solstice LBA (Fig. 12), safety coefficients also are higher. The safety coefficient tends to decrease with increasing bio-based polyol content in rigid PU foams, especially for the ETOFA-TMP series. This series also had higher mechanical properties, once again showing a direct correlation between mechanical properties and safety coefficient.
Figure 12: Safety coefficient of rigid PU foams: TMP-Opteon series (A), DEG-Opteon series (B) and DEG-Solstice series (C)
The TGA method was used to determine the thermal stability of the rigid PU foams. Figs. 13 and 14displays the TGA curves of rigid PU foams. All rigid PU foams underwent four distinct degradation phases during the heating process. Based on literature data peak 1 (T~180°C) is related to the release of lower molecular weight compounds, such as moisture, catalysts, flame retardant, and unreacted polyols/isocyanate, peak 2 and peak 3 (T~300–350°C) is assigned to release of compounds from soft segments of the PU polymer matrix, and peak 4 (T~450°C) is attributed to the release of heavier hard segments of the PU polymer matrix such as aromatics [41]. The onset temperature remained similar for all 30 analyzed rigid PU foams. The only noticeable difference in onset temperature is that rigid PU foams containing ETOFA-TEOA polyol had slightly higher onset temperatures than rigid PU foams containing ETOFA-DEOA polyol. This might be explained by higher crosslinking for rigid PU foams containing ETOFA-TEOA polyols. Residues at 700°C are in the range of 17.6%–27.3%.
Figure 13: TGA curves of rigid PU foams with different polyols and blowing agents
Figure 14: TGA curves of rigid PU foams with different ETOFA-TMP polyol content
DSC analysis is a widely used technique for the structure investigation of different polymeric materials. DSC is used for the determination of crystallization and glass transition temperature. DMA also measures glass transition temperature but is more sensitive than DSC. The technique involves sinusoidally straining and relaxing a sample at a given frequency as the temperature is raised.
Both methods showed no thermal transitions in the negative temperature region, indicating that materials are suitable for cryogenic temperatures. The glass transition temperature detected by DSC (Fig. 15) was lower than that detected by DMA (Fig. 16). It might be due to the different test mechanisms of both methods and the larger sample size for DMA. Glass transition temperature tended to decrease by increasing ETOFA polyol content in rigid PU formulation, especially evident it is in DMA curves (Fig. 12). As ETOFA polyols mainly consist of long chain fatty acids as result rigid PU foams containing more ETOFA polyols have more flexible structures than rigid PU foams that have more NEO 240 polyol with more aromatic structures.
Figure 15: DSC curves of rigid PU foams with (A) different polyols and blowing agents and (B) rigid PU foams obtained with Opteon 1100 and different ETOFA-TMP polyol content
Figure 16: DMA curves of rigid PU foams with (A) different polyols and blowing agents and (B) rigid PU foams obtained with Opteon 1100 and different ETOFA-TMP polyol content
The highest storage modulus at maximum has rigid PU foams containing ETOFA-TMP polyol. With increasing temperature ETOFA-TMP polyol containing rigid PU foams show two distinct regions in storage modulus curve (Fig. 16). First region storage modulus starts to gradually decrease from about 20°C to about 75°C and, second region-after 75°C sudden decrease occurs. First region can be explained by more tightly packed and crosslinked polymer matrix of rigid PU foams containing ETOFA-TMP polyol. In this rigid PU foams became slightly more mobile not quite reaching glass transition. In the second stage rigid PU foams have reached glass transition. This stage is observed rigid PU foams containing ETOFA-DEG polyol as well. With increasing ETOFA-TMP polyol content storage modulus maximum increases as well, meaning that rigid PU foam stiffness also increases. It agrees with theoretical Mc values.
We have developed rigid PU foams containing bio-based and recycled polyols as well as 4th-generation generation blowing agents for cryogenic applications. These foams demonstrated several fundamental properties essential for effective cryogenic insulation, such as low thermal conductivity, mechanical strength, and adhesion to aluminium.
The obtained rigid PU foams coefficient of thermal conductivity is strongly influenced by the choice of physical blowing agent, indicating that Solstice LBA resulted in improved thermal conductivity compared to Opteon 1100.
Mechanical testing revealed the foam’s anisotropic behavior, with compression and tensile strength differing between directions parallel and perpendicular to the foam rise. Rigid PU foams containing ETOFA-TMP polyol have higher mechanical properties, likely due to increased crosslinking density within the foam structure.
Rigid PU foams containing Solstice LBA exhibit notably higher adhesion properties compared to Opteon 1100.
The thermal stability assessment using TGA and DSC confirmed the suitability of the foam materials for cryogenic temperatures.
This study has elucidated the viability of bio-based rigid PU foams as effective cryogenic insulation materials. The developed rigid PU foams exhibited competitive thermal, mechanical, and adhesion properties by incorporating recycled and renewable polyols and advanced blowing agents.
Acknowledgement: The authors are thankful to Bernadetta Boneberger from Evonik GmbH for supplying surfactants for rigid PU foams.
Funding Statement: This research was funded by projects “Smart Materials, Photonics, Technologies and Engineering Ecosystem (MOTE)” (Contract No. VPP-EM-FOTONIKA-2022/1-0001) and “Bio-Based Cryogenic Insulation for Aerospace Application (BioSpace)” (Contract No. 4000135271/21/NL/SC).
Author Contributions: The authors confirm their contribution to the paper as follows: study conception and design: L. Vevere, U. Cabulis, M. Kirpluks; data collection: L. Vevere, B. Sture, V. Yakushin; analysis and interpretation of results: L. Vevere, M. Kirpluks, B. Sture; draft manuscript preparation: L. Vevere; supervision: U. Cabulis. All authors reviewed the results and approved the final version of the manuscript.
Availability of Data and Materials: The data that support the findings of this study are available from the corresponding author, L. Vevere, upon reasonable request.
Conflicts of Interest: The authors declare that they have no conflicts of interest to report regarding the present study.
References
1. Zhang, Z., Zhao, S., Li, K., Fei, Z., Chen, G. et al. (2023). Hydrophobic and elastic silica-based aerogels with organic and inorganic in-situ hybrid structure for cryogenic insulation. Materials Letters, 338, 134048. [Google Scholar]
2. Zhang, S., Wang, J., Lu, K., Xu, G., Wang, Z. et al. (2022). Polybenzoxazine aerogels for thermal protection at extremely high-temperature/cryogenic conditions. Polymer, 261, 125424. [Google Scholar]
3. Swanger, A. M., Guerrero Nacif, M. A. (2022). Passive cooling in aerogel-based insulation systems for liquid hydrogen upper stage launch vehicle tanks. Cryogenics, 128, 103591. [Google Scholar]
4. Zhang, X. B., Chen, J. Y., Gan, Z. H., Qiu, L. M., Zhang, K. H. et al. (2014). Experimental study of moisture uptake of polyurethane foam subjected to a heat sink below 30 K. Cryogenics, 59, 1–6. [Google Scholar]
5. Fesmire, J. E., Coffman, B. E., Meneghelli, B. J., Heckle, K. W. (2012). Spray-on foam insulations for launch vehicle cryogenic tanks. Cryogenics, 52(4–6), 251–261. [Google Scholar]
6. Miyakita, T., Hatakenaka, R., Sugita, H., Saitoh, M., Hirai, T. (2014). Development of a new multi-layer insulation blanket with non-interlayer-contact spacer for space cryogenic mission. Cryogenics, 64, 112–120. [Google Scholar]
7. Jiang, W., Yang, Y., Hu, C., Li, P., Sun, P. et al. (2023). Experimental study on composite insulation with foam, multilayer and vapor cooled shield for cryogen storage under different vacuum conditions. Cryogenics, 129, 103604. [Google Scholar]
8. Deng, B., Yang, S., Xie, X., Wang, Y., Bian, X. et al. (2019). Study of the thermal performance of multilayer insulation used in cryogenic transfer lines. Cryogenics, 100, 114–122. https://doi.org/10.1016/j.cryogenics.2019.01.005 [Google Scholar] [CrossRef]
9. Jiang, W., Sun, P., Li, P., Zuo, Z., Huang, Y. (2021). Transient thermal behavior of multi-layer insulation coupled with vapor cooled shield used for liquid hydrogen storage tank. Energy, 231, 120859. [Google Scholar]
10. Du, J., Wang, H., Huang, Z., Liu, X., Yin, X. et al. (2023). Construction and mechanism study of lignin-based polyurethane with high strength and high self-healing properties. International Journal of Biological Macromolecules, 248, 125925. [Google Scholar] [PubMed]
11. Huang, Z., Wang, H., Du, J., Liu, X., Pan, G. et al. (2023). High-strength, self-reinforcing and recyclable multifunctional lignin-based polyurethanes based on multi-level dynamic cross-linking. Chemical Engineering Journal, 473, 145423. [Google Scholar]
12. Kurańska, M., Pinto, J. A., Salach, K., Barreiro, M. F., Prociak, A. (2020). Synthesis of thermal insulating polyurethane foams from lignin and rapeseed based polyols: A comparative study. Industrial Crops and Products, 143, 111882. [Google Scholar]
13. Leszczyńska, M., Ryszkowska, J., Szczepkowski, L., Kurańska, M., Prociak, A. et al. (2020). Cooperative effect of rapeseed oil-based polyol and egg shells on the structure and properties of rigid polyurethane foams. Polymer Testing, 90, 106696. [Google Scholar]
14. Kairyte, A., Vejelis, S. (2015). Evaluation of forming mixture composition impact on properties of water blown rigid polyurethane (PUR) foam from rapeseed oil polyol. Industrial Crops and Products, 66, 210–215. [Google Scholar]
15. Kurańska, M., Prociak, A. (2016). The influence of rapeseed oil-based polyols on the foaming process of rigid polyurethane foams. Industrial Crops and Products, 89, 182–187. [Google Scholar]
16. Chi, J., Zhang, Y., Yu, S., Ju, A., Li, Y. et al. (2023). The thermal and combustion properties of soybean oil phosphate-based polyurethane foam composites containing polyol of etidronic acid. Reactive and Functional Polymers, 183, 105499. [Google Scholar]
17. Mizera, K., Ryszkowska, J. (2016). Polyurethane elastomers from polyols based on soybean oil with a different molar ratio. Polymer Degradation and Stability, 132, 21–31. [Google Scholar]
18. Marcovich, N. E., Kurańska, M., Prociak, A., Malewska, E., Kulpa, K. (2017). Open cell semi-rigid polyurethane foams synthesized using palm oil-based bio-polyol. Industrial Crops and Products, 102, 88–96. [Google Scholar]
19. Polaczek, K., Kurańska, M., Auguścik-Królikowska, M., Prociak, A., Ryszkowska, J. (2021). Open-cell polyurethane foams of very low density modified with various palm oil-based bio-polyols in accordance with cleaner production. Journal of Cleaner Production, 290, 125875. [Google Scholar]
20. Ng, W. S., Lee, C. S., Chuah, C. H., Cheng, S. F. (2017). Preparation and modification of water-blown porous biodegradable polyurethane foams with palm oil-based polyester polyol. Industrial Crops and Products, 97, 65–78. [Google Scholar]
21. Sture, B., Vevere, L., Kirpluks, M., Godina, D., Fridrihsone, A. et al. (2021). Polyurethane foam composites reinforced with renewable fillers for cryogenic insulation. Polymers, 44(3), 1494–1511. [Google Scholar]
22. Polaczek, K., Kaulina, E., Pomilovskis, R., Fridrihsone, A., Kirpluks, M. (2022). Epoxidation of tall oil fatty acids and tall oil fatty acids methyl esters using the SpinChem® rotating bed reactor. Journal of Polymers and the Environment, 30, 4774–4786. [Google Scholar]
23. Ajao, O., Benali, M., Faye, A., Li, H., Maillard, D. et al. (2021). Multi-product biorefinery system for wood-barks valorization into tannins extracts, lignin-based polyurethane foam and cellulose-based composites: Techno-economic evaluation. Industrial Crops and Products, 167, 113435. [Google Scholar]
24. Zhang, T., Yu, M., Huang, Y., Tan, J., Zhang, M. et al. (2022). Design and manufacturing of cost-effective tannin-based polyurethane foam as an efficient and reusable absorbent for oil and solvents. Industrial Crops and Products, 189, 115815. [Google Scholar]
25. Recupido, F., Lama, G. C., Ammendola, M., Bossa, F. D. L., Minigher, A. et al. (2023). Rigid composite bio-based polyurethane foams: From synthesis to LCA analysis. Polymer, 267, 125674. [Google Scholar]
26. Churchill, J. G. B., Borugadda, V. B., Dalai, A. K. (2024). A review on the production and application of tall oil with a focus on sustainable fuels. Renewable and Sustainable Energy Reviews, 191, 114098. [Google Scholar]
27. Kirpluks, M., Vanags, E., Abolins, A., Michalowski, S., Fridrihsone, A. et al. (2020). High functionality bio-polyols from tall oil and rigid polyurethane foams formulated solely using bio-polyols. Materials, 13(8), 1985. [Google Scholar] [PubMed]
28. Raheem, A. B., Noor, Z. Z., Hassan, A., Abd Hamid, M. K., Samsudin, S. A. et al. (2019). Current developments in chemical recycling of post-consumer polyethylene terephthalate wastes for new materials production: A review. Journal of Cleaner Production, 225, 1052–1064. [Google Scholar]
29. Regulation (EU) No 517/2014 of the European parliament and of the council of 16 April 2014 on fluorinated greenhouse gases and repealing Regulation (EC) No 842/2006. https://www.eea.europa.eu/policy-documents/regulation-eu-no-517-2014 (accessed on 20/01/2024). [Google Scholar]
30. OpteonTM 1100 data sheet. https://www.opteon.com/en/-/media/files/opteon/opteon-1100-data-sheet.pdf?la=en&rev=6fe188c74519495a9b96d744254906b2 (accessed on 20/01/2024). [Google Scholar]
31. SOLSTICE® data sheet. https://prod-edam.honeywell.com/content/dam/honeywell-edam/pmt/oneam/en-us/blowing-agents/documents/pmt-am-solstice-lba-datasheet.pdf (accessed on 20/01/2024). [Google Scholar]
32. Uram, K., Prociak, A., Vevere, L., Pomilovskis, R., Cabulis, U. et al. (2021). Natural oil-based rigid polyurethane foam thermal insulation applicable at cryogenic temperatures. Polymers, 13(24), 4276. [Google Scholar] [PubMed]
33. Yakushin, V., Rundans, M., Holynska, M., Sture, B., Cabulis, U. (2023). Influence of reactive amine-based catalysts on cryogenic properties of rigid polyurethane foams for space and on-ground applications. Materials, 16(7), 2798. [Google Scholar] [PubMed]
34. Andersons, J., Cabulis, P., Kirpluks, M. (2022). The effect of crosslink density on the physical and mechanical properties of bio-based polyurethane foams. Macromolecular Symposia, 404(1), 1–4. [Google Scholar]
35. Mazar, A., Paleologou, M. (2023). New approach to recycle and valorize the first filtrate of the LignoForce systemTM: Lignin extraction and its use in rigid lignin-based polyurethane foams. International Journal of Biological Macromolecule, 244, 125346. [Google Scholar] [PubMed]
36. Zemła, M., Prociak, A., Michałowski, S. (2022). Bio-based rigid polyurethane foams modified with phosphorus flame retardants. Polymers, 14(1), 102. [Google Scholar]
37. Vasilev, A., Lorenz, T., Breitkopf, C. (2021). Thermal conductivities of crosslinked polyisoprene and polybutadiene from molecular dynamics simulations. Polymers, 13(3), 315. [Google Scholar] [PubMed]
38. Zhang, C., Jia, X., Quan, H. (2016). Synthesis of Z-1,1,1,4,4,4-hexafluoro-2-butene from hexachlorobutadiene. Journal of Fluorine Chemistry, 191, 77–83. [Google Scholar]
39. Tran, H. T. T., Deshan, A. D. K., Doherty, W., Rackemann, D., Moghaddam, L. (2022). Production of rigid bio-based polyurethane foams from sugarcane bagasse. Industrial Crops and Products, 188, 115578. [Google Scholar]
40. Wang, H., Liu, Y., Lin, L. (2023). Behavior characteristics and thermal energy absorption mechanism of physical blowing agents in polyurethane foaming process. Polymers, 15(10), 2285. [Google Scholar] [PubMed]
41. Cervantes-Uc, J. M., Espinosa, J. I. M., Cauich-Rodríguez, J. V., Ávila-Ortega, A., Vázquez-Torres, H. et al. (2009). TGA/FTIR studies of segmented aliphatic polyurethanes and their nanocomposites prepared with commercial montmorillonites. Polymer Degradation and Stability, 94(10), 1666–1677. [Google Scholar]
Cite This Article
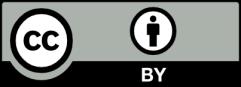