Open Access
REVIEW
Reconstruction of the Hindlimb Locomotion of Confuciusornis (Aves) and Its Implication for the Origin of Avian Flight
1 Institute of Geology and Paleontology, Linyi University, Linyi, 276000, China
2 Fengning Museum of Paleontology, Chengde, 068300, China
* Corresponding Author: Ying Guo. Email:
Molecular & Cellular Biomechanics 2023, 20(2), 49-61. https://doi.org/10.32604/mcb.2023.041173
Received 13 April 2023; Accepted 27 June 2023; Issue published 15 September 2023
Abstract
As one of the most basal avian clades, the Confuciusornithids are ideal in revealing the early evolution of avian flight. Birds’ hindlimbs are functionally diverse and contain a wealth of information about their behavior. The hindlimb of Confuciusornis, however, has only been studied in detail in terms of functional morphology, and quantitative studies that directly assess locomotor ability are relatively lacking. This has led to certain controversies on the behavior of Confuciusornis. This paper reviews the debates over the life habits and take-off ability of Confuciusornis, which are closely related to their hindlimb function. Several methodologies adopted engineering techniques, including the geometrical analysis of long bones, physiological reconstruction of muscles, kinematic and kinetic characteristics estimating, and appendage locomotor mechanism analysis, are recommended for estimating the hindlimb functions of Confuciusornis. Considering that the fossil bones are fragile, irregular in shape, and usually deformed, it is appropriate to apply computer numerical simulation techniques to such studies. A sufficient functional quantitative study will help clarify early bird locomotor behavior, which will provide clues and evidence for further exploration of the origin of bird flight and early bird movement.Keywords
The origin of flight has attracted scientific attention since the advent of evolutionary theory, and several origin-of-flight hypotheses have been proposed [1–3]. However, there has been no consensus established. Because the framework used to construct the theories historically was rather polarized [2]. Discussion has primarily focused on whether flight evolved in an arboreal or terrestrial setting, and if it was preceded by gliding or flapping.
Many scholars posited that birds have compartmentalized the single locomotor unit of their ancestors into discrete wing and leg modules [4], and exploited terrestrial and aerial environments. The instinctive perspective is that wing modules function for aerial activities, whereas leg modules function for terrestrial (e.g., Butler [5]; Heers et al. [6]). However, this paradigm may be also polarized. Extant birds frequently use their wings and legs jointly to navigate complex situations [6–8]. For modern birds, the forelimbs, hindlimbs, and tail are three independent but coordinated locomotion modules. The forelimbs and tail are coupled functionally during flight; when the bird is not in flight, the forelimbs, hindlimbs, and tail are decoupled functionally [4,9]. The seemingly simple cooperation of locomotion modules in birds affects their speed, efficiency, persistence, and mobility, which are closely related to behaviors for meeting survival needs such as grabbing food, fleeing from enemies, and pursuing mates. The significance of comprehending hindlimb evolution is highlighted by the findings of Benson et al. [10]. Their study demonstrates that the rates of species diversification in birds did not immediately increase following the evolution of flight. Instead, it was the development of a distinctive hindlimb module that facilitated a Cretaceous radiation. These findings underscore the importance of studying hindlimb evolution in order to gain a comprehensive understanding of avian diversification.
According to the characters of Confuciusornis, such as the pectoral girdle that differs from that in modern birds, the relatively long pygostyle, sternum with an underdeveloped carina, the relatively large hindlimb feathers, and the lack of foramen triosseum [11–13], their three locomotion modules should be different from those of extant birds in terms of the relationship between function and configuration. However, the aerodynamic mechanism is extremely complicated when birds and air interact during flight, and there are still many questions beyond the current level of understanding [14,15]. Therefore, it is necessary to start with relatively simple problems to study Confuciusornis flight.
In this review, we summarise the main puzzles on the hindlimb locomotion of Confuciusornis that have obstructed our further understanding of the origin of avian flight. We also present an overview of the engineering approaches applicable to clarify the hindlimb function of Confuciusornis and other early birds, highlighting experimental strategies with the considerable potential and providing a certain methodological synthesis for future research.
2 Status of Flight Ability of Confuciusornis
Confuciusornis is a large, primitive bird that existed 120~131 million years ago but is now extinct. During the Lower Cretaceous, Confuciusornis, an important genus of basal avian taxa, went through a massive increase in both population size and evolutionary diversity [12,16–19]. It is known for its richly preserved specimens, extremely long period of existence, and being the first to evolve the beak in birds [1,11,17]. Locomotion-related structures of Confuciusornis, such as the sternum, forelimb, hindlimb, feathers, soft tissues et al., have been studied in detail [13,20–32], which provides valuable information to our understanding of the habit of early birds. Although Confuciusornis are primitive for modern birds, they already possessed many relatively advanced avian anatomical characteristics. They have imbricate arranged wing feathers [24], long and asymmetrical primary feathers with robust rachises [24], the pro- and postpatagium [25], the propatagium ligament [32], the larger ossified sternum, flexible hands [23]. These factors suggest that Confuciusornis was capable of powered flight and was probably a better flyer than Archaeopteryx. There is even a computational fluid dynamic study clearly shows that Confuciusornis can glide for a certain distance when its initial flight speed exceeded 4 m/s [31]. Nonetheless, it is still a long way from fully understanding the habits of Confuciusornis, and even some crucial issues such as whether Confuciusornis preferred arboreal or terrestrial habits, and whether it could take-off from the ground is somewhat controversial [12,13,17,18,20,23–26].
The flight behaviour and apparatus that directly affect flight (e.g., wings, feathers) of Confuciusornis are always the focus of many studies; their take-off behaviour, which is also key to understanding the origin of avian flight, is somewhat ignored by researchers. Many studies on extant birds suggested that hindlimbs play a critical role in propelling birds to take off from the ground and perches [8,33–37]. Furthermore, the three widely accepted hypotheses about the origin of avian flight (Fig. 1), the ground-up, tree-down, and wing-assisted incline running, all contend that birds need to take flight by running or jumping to a specific speed and height [2,15,38]. In view of the limited quantitative research on the locomotor function of the hindlimb of Confuciusornis, further research should systematically investigate the hindlimb function of Confuciusornis and other Lower Cretaceous birds, focusing on biomechanically-based quantitative functional analysis. It will benefit studying critical evolutionary and ecological issues such as the origin of avian flight and locomotor evolution of early birds.
Figure 1: Functional roles of avian hindlimbs in three widely accepted hypotheses of origin of avian flight. (A) In tree-down hypothesis frame, birds jump to take-off. (B) In ground-up hypothesis frame, birds jump or run to take-off. (C) In wing-assisted incline running hypothesis frame, birds run to take-off
3 Hindlimbs: A New Perspective to Explore the Origins of Bird Flight
Bird forelimbs evolved into wings for flight, while their hindlimbs are responsible for body support and arboreal, terrestrial, and swimming locomotion. As early as the Mesozoic era, the hindlimbs were already diverged greatly among birds [39]. The multipurpose design of the hindlimbs is thought to be an important factor that help birds colonize diversity environments [9,40,41]. Avian hindlimbs show demonstrable direct links to ecology [42,43] and life habit [44,45]. Meanwhile, the adaptation to flight eased constraints on the hindlimb function and potentially increased interspecies differentiation of the hindlimb function of birds [4,41]. The hindlimb of birds with different habits can vary greatly in morphology and other characteristics of the skeleton and muscles, such as the length proportions of the three long leg bones [41,44], mechanical performance of bones [45], foot types [46], and myological traits [47,48]. Hindlimbs played an important role for studying the functional morphology of locomotion and phylogenetics of birds. Furthermore, compared to the drastic changes in the forelimbs and tails due to adaptation to flight, modern birds have hindlimb structures relatively similar to early birds and non-avian theropods [4,9,49]. The three-long boned hindlimb has long appeared in their dromaeosauridian ancestors, although they have many anatomical differences [41]. It indicates that they may have some similarities in appendage function and kinematic and kinetic characteristics [50]. Therefore, it is applicable and advantageous to use the functional characteristic of hindlimbs as an indicator of early bird habits.
Confuciusornis is a representative species of basal birds, and specimens of this species are numerous and well-preserved. The study of Confuciusornis has significantly improved our understanding of the ecology of early birds [1,11,17]. However, there are still controversies over the life habits and take-off of Confuciusornis and a lack of quantitative analysis of their locomotor ability. It hinders our intellect from understanding the origin and evolution of avian flight. The hindlimb function may provide new clues for further exploring these questions. Hindlimb function is key to understanding the habits and take-off mode of Confuciusornis, and therefore to exploring the origins of avian flight. Controversial arguments have been around for ages about whether the Confuciusornis had a terrestrial or arboreal lifestyle. Paleontologists have been profoundly concerned about this issue because it is directly related to the ground-up and tree-down hypotheses of the origin of avian flight. Confuciusornis has many characteristics adapted to arboreal lifestyle, such as separate hand phalanges, well-developed manual claws, highly recurved pedal claws, a reversed hallux, etc. [1,12,51]. Furthermore, Falk et al. [25] confirmed reticulate scales and robust phalangeal pads on the feet of Confuciusornis using laser-stimulate fluorescence, which strongly support Confuciusornis as an arboreal bird. However, the short and raised posterior digit of Confuciusornis indicates that it is not good at grasping branches [13,52]. Wang [20] deduced the ecology of Confuciusornis using principal components analysis on long bones and ternary plot analysis on phalanges and suggested that Confuciusornis may adapt for terrestrial life. In addition, Confuciusornis had claws on their wing digits, indicating their locomotion may be quite different from that of extant birds. They probably used both of their digit claws and pedal claws to move on branches or climb trunks, just like hoatzin nestlings do [2,52]. Their relatively unspecialized feet also suggested that Confuciusornis may adapt to arboreal or terrestrial life in entirely different ways than modern birds [21,22]. Therefore, it is difficult to infer the function of the hindlimbs of Confuciusornis based solely on morphological comparisons with extant birds. In this case, analysis based on biomechanical theory is needed to quantitatively clarify the locomotor ability of the hindlimb of Confuciusornis and other early birds. Such experiments and tests can directly estimate the functional implication of certain fossil characteristics, such as assessing the range of motion of joints according to their structure, and are relatively unrestricted by the locomotion patterns of modern birds. Furthermore, they can complement and validate the conclusions from qualitative analyses (e.g., morphological comparative studies) and vice versa.
The take-off behavior of early birds is closely related to the origin of avian flight, an in-depth study of the take-off ability of hindlimbs of Confuciusornis is the necessary work to explore the origin of avian flight. There are no studies specifically studying the take-off behavior of Confuciusornis, and whether Confuciusornis were capable of taking-off from the ground is often indirectly inferred based on their habits. Zhou et al. [51] suggested that the body mass of Confuciusornis were too large for their primary flight ability, so they could not take-off directly from the ground. In contrast, Chiappe et al. [13] argued that the flight-correlated skeletal features and the morphology of their wings and tail of Confuciusornis predict a considerable flight ability of them, they may therefore capable of taking-off from the ground. In the context of the habit and flight ability of Confuciusornis are still controversial [13,23,27,30], it is therefore unreliable to evaluate their take-off ability based on these factors. The take-off of most birds generally begins with jumping, running, paddling, etc. dominated by the hindlimbs, and when taking-off from the ground or perches, birds’ hindlimbs always produce most of the acceleration required to become airborne [8,34–37]. Even for hummingbirds with reduced hindlimbs, the leg thrust can contribute up to 59% to the take-off velocity [53]. The hindlimb propulsion contributes to the efficient taking-off of birds; it also helps birds obtain a higher initial flight speed to shorten the low-speed flight stage of high kinetic energy consumption [8,34,54]. Besides, an efficient take-off allows birds to quickly escape from predators [55]. Therefore, it is reasonable to assume that there was hindlimb propulsion in the take-off of Confuciusornis. Even if Confuciusornis can only glide, it may also need a certain initial speed to increase its gliding distance [31].
Recent research on the locomotion of extinct birds heavily relies on morphological comparisons with living birds. These studies estimated the functional implications of certain characteristics of extinct birds based on the form-function relationships derived from extant birds [21,22,41]. This methodology is conducive to integrating phylogeny and character optimization ideas into the locomotor reconstruction of extinct birds, preventing erroneous conclusions from analysing a certain group in isolation from ancestral characteristics [49]. It also provides data for systematic character evolution research. Nonetheless, some limitations of this approach should be noted. Firstly, most of the parameters used in these studies are morphological characteristics (such as bone length, claw curvature, etc.) that can only indirectly reflect the functional role of the structure. Secondly, comparative morphological analysis can generally infer the behaviour of extinct birds. Still, it is challenging to quantify their locomotor ability (such as the grasping force of the claw, running speed, etc.). As this methodology is an indirect functional analysis, its conclusions should be confirmed by additional experiments. Due to the above limitations, further work should pay more attention to the quantitative study on the locomotor ability of extinct birds. Such research can directly evaluate avian locomotor ability, which complements and validates comparative and statistical analyses of morphological traits.
4 Adopting Engineering Techniques to Study Bird Locomotion
Long bones and muscles are central apparatus of a vertebrate’s appendage, and their properties are key to locomotion. Long bones, as the rigid support for appendages, are primarily responsible for carrying and transmitting loads during locomotion [56,57]. Even though bone fossils lose their original material properties, the adaptation of vertebrate long bones to locomotion is primarily accomplished by modifying their structural properties [58,59]. Therefore, it is possible and plausible to reconstruct the locomotion of extinct birds using the structural properties of long bones. The geometrical analysis of bones has been widely used by extant vertebrate locomotion research, and it has shown advantages in studies such as the interspecies comparison of animal habits [45,60–62] and the change of locomotor characteristics during ontogeny of animals [62–64]. The extinct birds and other extinct vertebrates mainly retain bones. Due to the close connection between the structure of long bones and animal locomotion, it is essential to clarify the structural properties of long bones to analyze and reconstruct the locomotion of Confuciusornis.
Vertebrate locomotion is driven by muscle, and muscle’s physiological characteristics significantly impact the vertebrates’ locomotor ability [65–67]. Muscles and other soft tissues are rarely preserved in fossils, but the type, number, and physiological characteristics of muscles of extinct vertebrates can be inferred from osteological features preserved on fossil bones and by referencing muscles of living species [68–71]. Corresponding studies have proposed relatively complete protocols to reconstruct muscles in extinct vertebrates. These protocols have been used in studies such as estimating the locomotor ability of extinct vertebrates [72–75] and concluding the trend of the evolution of specific locomotor characteristics of vertebrates [50,76,77]. Studies on Confuciusornis muscle reconstruction are still relatively limited. Although some studies discussed muscles of Confuciusornis based on osteological features such as Zhou et al. [23] and Zhang et al. [17], but neither the muscle anatomical properties, such as mass and volume nor muscle architectural properties such as physiological cross-sectional area were estimated. It is necessary to supplement such work further to clarify the habit of Confuciusornis and provide data support for exploring the origin of avian flight and the paleoecology in Lower Cretaceous.
The question of how animals move should be clarified after the biomechanics of bones and muscles are determined. There are a range of kinematic (motion-based or geometric) and kinetic (force-based) parameters to describe an vertebrate’s hindlimb locomotion, such as posture (joint motion), speed, acceleration, ground reaction force, and gait (stride length, stride frequence, duty factor, etc.). For extant species, much of such data can be collected by in vivo experiments. The ground reaction force can be tested with a force platform [34], the motion can be recorded with a high-speed camera [78]. Moreover, X-ray Reconstruction of Moving Morphology can be used to record the motion of bones [79]. For extinct vertebrates, however, it is impossible to measure kinematic and kinetic parameters in vivo and it is also difficult (if not impossible) to do that in vitro. The musculoskeletal modeling and simulation are applicable solution to this problem. Its workflows involve acquiring digital fossil morphology and developing skeletal models, articulating digital bones together in jointed, defying joint mobility, reconstructing body shape and dimensions, reconstructing soft tissue, simulation and calculation [80–82]. Although each step has its challenges that must be deal with to make the model precise, the methodology offers a hopeful solution to the problem of locomotion reconstruction of extinct vertebrates. Another applicable approach to estimate the locomotion of extinct vertebrates is trackway analysis. Fossil trackways are the records of behavior that exist for extinct vertebrates; they provide evidences of some aspects of locomotion that bones cannot [70,83]. For example, the distance between corresponding point on successive prints of the same foot is the stride length, based on what the speed can be estimated [84,85]. Step width, the mediolateral (transverse) distance between successive prints, is closely related to mediolateral stability during bipedal locomotion. Therefore, it reflects the gait that bipeds adopted in locomotion [86]. Additionally, fossil trackways are also used in estimating limb posture of tetrapod [87] and revealing gaits of sauropod dinosaurs [88]. Fossil trackways of Mesozoic birds are abundant and distributed worldwide [83,89,90]. The walking, running, courtship, feeding and even flight (taking-off and landing) of extinct birds have been record [83,89,91], reflcting the high behavioral diversity of Mesozoic. Unfortunately, no fossil trackways of Confuciusornis have been found so far.
During the evolution of avian flight, the limb muscle and bones of bird-line theropods changed drastically in anatomical characteristics and therefore their functions [4,92,93]. Clarifing the forces exerted by muscles and the mechanical environment in which bones are subjected during locomotion, here we called them appendage locomotor mechanism, is necessary to analyze their evolution changes. Unlike engineering components, muscles and bones have irregular geometry, anisotropic material properties, and complex mechanical environments. Once again, computer technology acts as a powerful tool for this problems. Inverse simulation is a popular technique for studying the locomotor mechanisms of vertebrates (Fig. 2). Substrate reaction loads (kinetics) and limb posture (kinematics) are taken as input data in the inverse simulation, based on which to back-calculate muscle forces and joint load required to balance the limb during locomotion [80,94,95]. Due to its low computational cost and high accuracy, inverse simulation is widely used in the locomotion study of both extinct and extant vertebrates [76,96,97]. An alternative to the inverse simulation is the forward simulation. It uses the model and a physics engine to solve the optimal solution of kinematic and kinetic patterns that satisfies the set conditions [98,99]. Although the forward simulation is extremely computationally expensive, it does not require kinematic and dynamic parameters as known conditions, giving it an advantage in the study of the locomotion of extinct species [75,97,99]. Another computer technology, finite element analysis (FEA), is a powerful tool for handling biomechanical problems of biological structures of complex geometries and material properties (Fig. 3). FEA simplifies the complex structure, material, and mechanical condition by discretizing the object into a large number of simple elements so that complex mechanical problems can be solved [100–102]. Moreover, FEA offers the option to change the parameter of interest while maintaining the values of all other parameters, isolating a specific characteristic’s impact on the mechanical behaviour [100]. Therefore, it is frequently used in studies of skeletal function [101–104]. FEA provides an important advantage in applying the inductive form-function relationship of biological structure and hypothesis-driven research.
Figure 2: Musculoskeletal model of an ostrich (Struthio camelus) hindlimb. The musculoskeletal model built by Rankin et al. [97] is anatomically accurate, and the authors computed the force and power of hindlimb muscles of an ostrich based on it. Adapted from Seth et al. [80]
Figure 3: Stepwise description of finite element modelling of a bone. (A) The bone was CT scanned to digitize its geometry. Cross-sectional images (B) were reconstructed based on micro-CT images, which were then used to build the 3D model (C). (D) The 3D model was discretized into many simple elements to build the finite element model of the bone. Adapted from Wei et al. [104]
At present, few studies have systematically analyzed the locomotion mechanisms of Confuciusornis. Even though some studies on the functional evolution of biological structure have dabbled in this topic [50,76], they have mainly concentrated on the evolution of the form-function relationship of biological structure and have not performed in-depth analyses of individual taxa. Therefore, future research should use computer technology to conduct more systematic studies on the locomotion mechanism of Confuciusornis, which can significantly improve our understanding of how early birds move.
Confuciusornis, which lived in the Lower Cretaceous, has attracted widespread attention from paleontologists and geologists because of its key status in avian evolution and high quality of fossil preservation. The habit reconstruction of Confuciusornis is of great value for fundamental evolutionary questions such as the origin of avian flight and locomotor evolution of early birds. Given that most research focuses on the flight ability of birds and the apparatus most closely related to flight, such as the forelimbs and feathers. The hindlimbs, as the primary apparatus for various functions of birds, such as take-off, landing, running, and predation, have the potential to serve as a new entry point for studying early birds’ behavior.
By systematically analyzing the biomechanical characteristics of hindlimb long bones, muscle, and the hindlimb locomotion mechanism, we can further investigate questions concerning the origin of avian flight and their environmental adaptation strategies. With the advancement of modern computer technology, numerical simulation analysis provides an innovative and potent tool for the study of the habits of early birds. The inverse and forward simulations can quantitatively determine early bird kinetic and kinematic characteristics in various locomotion. Exploring the complex mechanical state and mechanical performance of apparatus during locomotion is facilitated by FEA. Further research should examine the locomotor function of the hindlimbs of Confuciusornis, emphasizing quantitative functional analysis grounded in biomechanical theory. It is essential to utilize computer technology to identify and reconstruct the hindlimb locomotion mechanism of early birds and provide evidence and clues for further exploration of the origin and evolution of avian flight for the advancement of scientific knowledge.
Acknowledgement: The authors’ sincere gratitude is offered to Fucheng Zhang from Linyi University for his constructive comments on the early discussion.
Funding Statement: This research was funded by Shandong Provincial Natural Science Foundation, China (ZR2022QD084), the National Natural Science Foundation of China (42002016), and the project from the Natural Resources and Planning Bureau of Fengning Manchu Autonomous County, China (HX180056 and HX210003).
Author Contributions: Conceptualization, X. W. and Y. G.; methodology, X. W.; software, X. W. and Y. G.; validation, X. W., Y. G. and Y. Z.; investigation, X. W.; resources, Y. G.; data curation, X. W.; writing—original draft preparation, X. W. and Y. G.; writing—review and editing, X. W. and Y. G. All authors have read and agreed to the published version of the manuscript.
Availability of Data and Materials: The data underlying this article are available in the article and in its references. The derived data generated in this research will be shared on reasonable request to the corresponding author.
Conflicts of Interest: The authors declare that the research was conducted in the absence of any commercial or financial relationships that could be construed as a potential conflict of interest.
References
1. Zhou, Z. (2004). The origin and early evolution of birds: Discoveries, disputes, and perspectives from fossil evidence. Naturwissenschaften, 91, 455–471 [Google Scholar] [PubMed]
2. Heers, A. M., Dial, K. P. (2012). From extant to extinct: Locomotor ontogeny and the evolution of avian flight. Trends in Ecology & Evolution, 27, 296–305. [Google Scholar]
3. Xu, X., Zhou, Z., Dudley, R., Mackem, S., Chuong, C. M. et al. (2014). An integrative approach to understanding bird origins. Science, 346, 1253293 [Google Scholar] [PubMed]
4. Gatesy, S. M., Dial, K. P. (1996). Locomotor modules and the evolution of avian flight. Evolution, 50, 331–340 [Google Scholar] [PubMed]
5. Butler, P. J. (1991). Exercise in birds. Journal of Experimental Biology, 160, 233–262. [Google Scholar]
6. Heers, A. M., Dial, K. P. (2015). Wings versus legs in the avian bauplan: Development and evolution of alternative locomotor strategies. Evolution, 69, 305–320 [Google Scholar] [PubMed]
7. Dial, K. P. (2003). Wing-assisted incline running and the evolution of flight. Science, 299, 402–404 [Google Scholar] [PubMed]
8. Provini, P., Tobalske, B. W., Crandell, K. E., Abourachid, A. (2012). Transition from leg to wing forces during take-off in birds. Journal of Experimental Biology, 215, 4115–4124 [Google Scholar] [PubMed]
9. Abourachid, A., Höfling, E. (2012). The legs: A key to bird evolutionary success. Journal of Ornithology, 153, 193–198. [Google Scholar]
10. Benson, R. B. J., Choiniere, J. N. (2013). Rates of dinosaur limb evolution provide evidence for exceptional radiation in Mesozoic birds. Proceedings of Biological Sciences, 280, 20131780. [Google Scholar]
11. Wang, M., O’Connor, J., Zhou, Z. (2019). A taxonomical revision of the confuciusornithiformes (Aves: Pygostylia). Vertebrata Palasiatica, 57, 1–37. [Google Scholar]
12. Hou, L., Zhou, Z., Martin, L. D., Feduccia, A. (1995). A beaked bird from the Jurassic of China. Nature, 377, 616–618. [Google Scholar]
13. Chiappe, L. M., Ji, S. A., Ji, Q., Norell, M. A. (1999). Anatomy and systematics of the confuciusornithidae (Theropoda, Aves) from the late Mesozoic of Northeastern China. Bulletin of the American Museum of Natural History, 242, 1–89. [Google Scholar]
14. Sun, M. (2018). Aerodynamics of animal flight. Acta Aerodynamica Sinica, 36, 122–128. [Google Scholar]
15. Chatterjee, S., Templin, R. J. (2012). Palaeoecology, aerodynamics, and the origin of avian flight. In: Talent, J. A. (Ed.Earth and life: global biodiversity, extinction intervals and biogeographic perturbations through time, pp. 585–612. Dordrecht: Springer Netherlands. [Google Scholar]
16. Hou, L., Martin, L. D., Zhou, Z., Feduccia, A., Zhang, F. (1999). A diapsid skull in a new species of the primitive bird Confuciusornis. Nature, 399, 679–682. [Google Scholar]
17. Zhang, F., Zhou, Z., Benton, M. J. (2008). A primitive confuciusornithid bird from China and its implications for early avian flight. Science China Earth Sciences, 51, 625–639. [Google Scholar]
18. Zhang, F., Zhou, Z., Li, D., Li, Z. (2009). The present situation of confuciusornithids research. Chinese Journal of Nature, 31, 8–11. [Google Scholar]
19. Ji, Q., Chiappe, L. M., Ji, S. (1999). A new late Mesozoic confuciusornithid bird from China. Journal of Vertebrate Paleontology, 19, 1–7. [Google Scholar]
20. Wang, M. (2014). Taxonomical revision, ontogenetic, habitat and phylogenetic analyses of enantiornithes (Aves: Ornithothoraces) of China (Ph.D. Thesis). Chinese Academy of Sciences, Beijing. [Google Scholar]
21. McIntosh, A. P. (2017). Geometric morphometric analysis of the pedal claw of the early cretaceous bird confuciusornis sanctus (Confuciusornithidae) and its functional and behavioral implications (Master of Science). DePaul University. [Google Scholar]
22. Falk, A. R., Lamsdell, J. C., Gong, E. (2021). Principal component analysis of avian hind limb and foot morphometrics and the relationship between ecology and phylogeny. Paleobiology, 47, 314–336. [Google Scholar]
23. Zhou, Z., Farlow, J. (2001). Flight capability and habits of Confuciusornis. In: Gauthier, J. A., Gall, L. F. (Eds.New perspectives on the origin and early evolution of birds, pp. 237–254. New Haven: Yale University Press. [Google Scholar]
24. Longrich, N. R., Vinther, J., Meng, Q., Li, Q., Russell, A. P. (2012). Primitive wing feather arrangement in Archaeopteryx Lithographica and Anchiornis Huxleyi. Current Biology, 22, 2262–2267 [Google Scholar] [PubMed]
25. Falk, A. R., Kaye, T. G., Zhou, Z., Burnham, D. A. (2016). Laser fluorescence illuminates the soft tissue and life habits of the early cretaceous bird Confuciusornis. PLoS One, 11, e0167284 [Google Scholar] [PubMed]
26. Zheng, X., O’Connor, J. K., Wang, X., Pan, Y., Wang, Y. et al. (2017). Exceptional preservation of soft tissue in a new specimen of Eoconfuciusornis and its biological implications. National Science Review, 4, 441–452. [Google Scholar]
27. Nudds, R. L., Dyke, G. J. (2010). Narrow primary feather rachises in Confuciusornis and Archaeopteryx suggest poor flight ability. Science, 328, 887–889 [Google Scholar] [PubMed]
28. Paul, G. S. (2010). Comment on “Narrow Primary Feather Rachises in Confuciusornis and Archaeopteryx suggest poor flight ability”. Science, 330, 320 [Google Scholar] [PubMed]
29. Lees, J., Garner, T., Cooper, G., Nudds, R. (2017). Rachis morphology cannot accurately predict the mechanical performance of primary feathers in extant (and therefore fossil) feathered flyers. Royal Society Open Science, 4, 160927 [Google Scholar] [PubMed]
30. Guo, Y., Zhao, Y., Zhou, Y., Yang, J., Wang, Y. et al. (2021). Computational fluid dynamics will shed a new light on functional-morphological studies of confuciusornithids (Aves) in Eastern Asia during early cretaceous. Fresenius Environmental Bulletin, 36, 7470–7475. [Google Scholar]
31. Wang, I., Guo, Y., An, X., Zhao, Y., Wang, J. (2019). Three-dimensional reconstruction and numerical simulation of flight performance of Confuciusornis. Open Journal of Natural Sciences, 7, 590–595. [Google Scholar]
32. Pittman, M., Kaye, T. G., Wang, X., Zheng, X., Dececchi, T. A. et al. (2022). Preserved soft anatomy confirms shoulder-powered upstroke of early theropod flyers, reveals enhanced early pygostylian upstroke, and explains early sternum loss. Proceedings of the National Academy of Sciences of the United States of America, 119, e2205476119 [Google Scholar] [PubMed]
33. Bonser, R., Rayner, J. (1996). Measuring leg thrust forces in the common starling. Journal of Experimental Biology, 199, 435–439 [Google Scholar] [PubMed]
34. Earls, K. D. (2000). Kinematics and mechanics of ground take-off in the starling Sturnis Vulgaris and the quail Coturnix coturnix. Journal of Experimental Biology, 203, 725–739 [Google Scholar] [PubMed]
35. Heppner, F. H., Anderson, J. G. T. (1985). Leg thrust important in flight take-off in the pigeon. Journal of Experimental Biology, 114, 285–288. [Google Scholar]
36. Provini, P., Abourachid, A. (2018). Whole-body 3D kinematics of bird take-off: Key role of the legs to propel the trunk. Science of Nature, 105, 12 [Google Scholar] [PubMed]
37. Crandell, K. E., Smith, A. F., Crino, O. L., Tobalske, B. W. (2018). Coping with compliance during take-off and landing in the diamond dove (Geopelia Cuneata). PLoS One, 13, e0199662 [Google Scholar] [PubMed]
38. Wang, M., Zhou, Z. (2017). The evolution of birds with implications from new fossil evidences. In: Maina, J. N. (Ed.The biology of the avian respiratory system: evolution, development, structure and function, pp. 1–26. Cham: Springer International Publishing. [Google Scholar]
39. Bell, A., Marugán-Lobón, J., Navalón, G., Nebreda, S. M., DiGuildo, J. et al. (2021). Quantitative analysis of morphometric data of pre-modern birds: Phylogenetic versus ecological signal. Frontiers in Earth Science, 9, 663342. [Google Scholar]
40. Provini, P., Goupil, P., Hugel, V., Abourachid, A. (2012). Walking, paddling, waddling: 3D kinematics anatidae locomotion (Callonetta leucophrys). Journal of Experimental Zoology Part A: Ecological Genetics and Physiology, 317, 275–282 [Google Scholar] [PubMed]
41. Gatesy, S. M., Middleton, K. M. (1997). Bipedalism, flight, and the evolution of theropod locomotor diversity. Journal of Vertebrate Paleontology, 17, 308–329. [Google Scholar]
42. Bell, A., Chiappe, L. M. (2011). Statistical approach for inferring ecology of Mesozoic birds. Journal of Systematic Palaeontology, 9, 119–133. [Google Scholar]
43. Navalón, G., Bjarnason, A., Griffiths, E., Benson, R. B. J. (2022). Environmental signal in the evolutionary diversification of bird skeletons. Nature, 611, 306–311. [Google Scholar]
44. Zeffer, A., Johansson, L. C., Marmebro, A. (2003). Functional correlation between habitat use and leg morphology in birds (Aves). Biological Journal of the Linnean Society, 79, 461–484. [Google Scholar]
45. Habib, M. B., Ruff, C. (2008). The effects of locomotion on the structural characteristics of avian limb bones. Zoological Journal of the Linnean Society, 153, 601–624. [Google Scholar]
46. Raikow, R. J. (1985). Systematic and functional aspects of the locomotor system of the Scrub-birds, Atrichornis, and lyrebirds, Menura (Passeriformes: Atrichornithidae and Menuridae). Records of the Australian Museum, 37(4), 211–228. [Google Scholar]
47. Wang, L., Wei, X., Liang, X., Zhang, Z. (2021). Ontogenetic changes of hindlimb muscle mass in Cabot’s tragopan (Galliformes, Phasianidae) and their functional implications. Anatomical Record, 304, 2841–2855. [Google Scholar]
48. Mosto, M. C. (2017). Comparative hindlimb myology within the family falconidae. Zoomorphology, 136, 241–250. [Google Scholar]
49. Gatesy, S. M. (2002). Locomotor evolution on the line to modern birds. In: Chiappe, L. M., Witmer, L. M. (Eds.Mesozoic birds: Above the heads of dinosaurs, pp. 432–447. Berkeley, CA: University of California Press. [Google Scholar]
50. Hutchinson, J. R., Allen, V. (2009). The evolutionary continuum of limb function from early theropods to birds. Naturwissenschaften, 96, 423–448 [Google Scholar] [PubMed]
51. Zhou, Z., Hou, L. (1998). Confuciusornis and the early evolution of birds. Vertebrata Palasiatica, 36, 136–146. [Google Scholar]
52. Zinoviev, A. V. (2009). An attempt to reconstruct the lifestyle of confuciusornithids (Aves, Confuciusornithiformes). Paleontological Journal, 43, 444–452. [Google Scholar]
53. Tobalske, B. W., Altshuler, D. L., Powers, D. R. (2004). Take-off mechanics in hummingbirds (Trochilidae). Journal of Experimental Biology, 207, 1345–1352 [Google Scholar] [PubMed]
54. Tobalske, B. W., Hedrick, T. L., Dial, K. P., Biewener, A. A. (2003). Comparative power curves in bird flight. Nature, 421, 363–366 [Google Scholar] [PubMed]
55. Kullberg, C., Lafrenz, M. (2007). Escape take-off strategies in birds: The significance of protective cover. Behavioral Ecology and Sociobiology, 61, 1555–1560. [Google Scholar]
56. Currey, J. D. (2002). Bones: Structure and mechanics. Princeton: Princeton University Press. [Google Scholar]
57. Currey, J. D. (2012). The structure and mechanics of bone. Journal of Materials Science, 47, 41–54. [Google Scholar]
58. Biewener, A. A. (1982). Bone strength in small mammals and bipedal birds: Do safety factors change with body size? Journal of Experimental Biology, 98, 289–301 [Google Scholar] [PubMed]
59. Erickson, G. M., CataneseIII, J., Keaveny, T. M. (2002). Evolution of the biomechanical material properties of the femur. Anatomical Record, 268, 115–124 [Google Scholar] [PubMed]
60. Cowgill, L. W., Warrener, A., Pontzer, H., Ocobock, C. (2010). Waddling and toddling: The biomechanical effects of an immature gait. American Journal of Physical Anthropology, 143, 52–61 [Google Scholar] [PubMed]
61. Nadell, J. A. (2017). Ontogeny and adaptation: A cross-sectional study of primate limb elements (Ph.D. Thesis). Durham University, Durham. [Google Scholar]
62. Wei, X., Zhang, Z. (2019). Ontogenetic changes of geometrical and mechanical characteristics of the avian femur: A comparison between precocial and altricial birds. Journal of Anatomy, 235, 903–911 [Google Scholar] [PubMed]
63. Carlson, K. J., Judex, S. (2007). Increased non-linear locomotion alters diaphyseal bone shape. Journal of Experimental Biology, 210, 3117–3125 [Google Scholar] [PubMed]
64. Agostini, G. M., Ross, A. H. (2011). The effect of weight on the femur: A cross-sectional analysis. Journal of Forensic Sciences, 56, 339–343 [Google Scholar] [PubMed]
65. Dickinson, M. H., Farley, C. T., Full, R. J., Koehl, M. A. R., Kram, R. et al. (2000). How animals move: An integrative view. Science, 288, 100–106 [Google Scholar] [PubMed]
66. Lieber, R. L., Fridén, J. (2000). Functional and clinical significance of skeletal muscle architecture. Muscle & Nerve, 23, 1647–1666. [Google Scholar]
67. Alexander, R. M. (2003). Principles of animal locomotion. Princeton: Princeton University Press. [Google Scholar]
68. Bryant, H. N., Russell, A. P. (1992). The role of phylogenetic analysis in the inference of unpreserved attributes of extinct taxa. Philosophical Transactions of the Royal Society B: Biological Sciences, 337, 405–418. [Google Scholar]
69. Witmer, L. M. (1995). The extant phylogenetic bracket and the importance of reconstructing soft tissues in fossils. In: Thomason, J. J. (Ed.Functional morphology in vertebrate paleontology, pp. 19–33. Cambridge, UK: Cambridge University Press. [Google Scholar]
70. Benton, M. J. (2010). Studying function and behavior in the fossil record. PLoS Biology, 8, e1000321 [Google Scholar] [PubMed]
71. Rayfield, E. J. (2019). What does musculoskeletal mechanics tell us about evolution of form and function in vertebrates? In: Bels, V., Whishaw, I. (Eds.Feeding in vertebrates, pp. 45–70. Cham: Springer. [Google Scholar]
72. Carrano, M. T., Hutchinson, J. R. (2002). Pelvic and hindlimb musculature of Tyrannosaurus Rex (Dinosauria: Theropoda). Journal of Morphology, 253, 207–228 [Google Scholar] [PubMed]
73. Hutchinson, J. R. (2002). The evolution of hindlimb tendons and muscles on the line to crown-group birds. Comparative Biochemistry and Physiology Part A: Molecular & Integrative Physiology, 133, 1051–1086. [Google Scholar]
74. Hutchinson, J. R., Frank, C. A., Silvia, S. B., Scott, L. D. (2005). Analysis of hindlimb muscle moment arms in Tyrannosaurus rex using a three-dimensional musculoskeletal computer model: Implications for stance, gait, and speed. Paleobiology, 31, 676–701. [Google Scholar]
75. Sellers, W. I., Pond, S. B., Brassey, C. A., Manning, P. L., Bates, K. T. (2017). Investigating the running abilities of Tyrannosaurus rex using stress-constrained multibody dynamic analysis. PeerJ, 5, e3420 [Google Scholar] [PubMed]
76. Allen, V. R., Kilbourne, B. M., Hutchinson, J. R. (2021). The evolution of pelvic limb muscle moment arms in bird-line archosaurs. Science Advances, 7, eabe2778 [Google Scholar] [PubMed]
77. Hutchinson, J. R. (2001). The evolution of femoral osteology and soft tissues on the line to extant birds (Neornithes). Zoological Journal of the Linnean Society, 131, 169–197. [Google Scholar]
78. Rubenson, J., Lloyd, D. G., Besier, T. F., Heliams, D. B., Fournier, P. A. (2007). Running in ostriches (Struthio camelusThree-dimensional joint axes alignment and joint kinematics. Journal of Experimental Biology, 210, 2548–2562 [Google Scholar] [PubMed]
79. Brainerd, E. L., Baier, D. B., Gatesy, S. M., Hedrick, T. L., Metzger, K. A. et al. (2010). X-ray reconstruction of moving morphology (XROMMPrecision, accuracy and applications in comparative biomechanics research. Journal of Experimental Zoology Part A: Ecological Genetics and Physiology, 313A(5), 262–279. [Google Scholar]
80. Seth, A., Hicks, J. L., Uchida, T. K., Habib, A., Dembia, C. L. et al. (2018). Opensim: Simulating musculoskeletal dynamics and neuromuscular control to study human and animal movement. PLoS Computational Biology, 14, e1006223 [Google Scholar] [PubMed]
81. Delp, S. L., Anderson, F. C., Arnold, A. S., Loan, P., Habib, A. et al. (2007). Opensim: Open-source software to create and analyze dynamic simulations of movement. IEEE Transactions on Biomedical Engineering, 54, 1940–1950 [Google Scholar] [PubMed]
82. Bishop, P. J., Cuff, A. R., Hutchinson, J. R. (2021). How to build a dinosaur: Musculoskeletal modeling and simulation of locomotor biomechanics in extinct animals. Paleobiology, 47, 1–38. [Google Scholar]
83. Lockley, M. G., Harris, J. D. (2010). On the trail of early birds: A review of the fossil footprint record of avian morphological and behavioral evolution. In: Ulrich, P. K. (Ed.Trends in ornithology research, pp. 1–63. Hauppauge: Nova Science Publishers. [Google Scholar]
84. Alexander, R. M. (1976). Estimates of speeds of dinosaurs. Nature, 261, 129–130. [Google Scholar]
85. Thulborn, R. A. (1982). Speeds and gaits of dinosaurs. Palaeogeography, Palaeoclimatology, Palaeoecology, 38, 227–256. [Google Scholar]
86. Bishop, P. J., Clemente, C. J., Weems, R. E., Graham, D. F., Lamas, L. P. et al. (2017). Using step width to compare locomotor biomechanics between extinct, non-avian theropod dinosaurs and modern obligate bipeds. Journal of the Royal Society Interface, 14, 20170276 [Google Scholar] [PubMed]
87. Kubo, T., Benton, M. J. (2009). Tetrapod postural shift estimated from permian and triassic trackways. Palaeontology, 52, 1029–1037. [Google Scholar]
88. Lallensack, J. N., Falkingham, P. L. (2022). A new method to calculate limb phase from trackways reveals gaits of sauropod dinosaurs. Current Biology, 32, 1635–1640 [Google Scholar] [PubMed]
89. Falk, A. R. (2011). Tracking Mesozoic birds across the world. Journal of Systematic Palaeontology, 9, 85–90. [Google Scholar]
90. Xing, L., Lockley, M. G., Jia, C., Klein, H., Niu, K. et al. (2021). Lower cretaceous avian-dominated, theropod, thyreophoran, pterosaur and turtle track assemblages from the Tugulu Group, Xinjiang, China: Ichnotaxonomy and palaeoecology. PeerJ, 9, e11476 [Google Scholar] [PubMed]
91. Falk, A. R., Lim, J. D., Hasiotis, S. T. (2014). A behavioral analysis of fossil bird tracks from the haman formation (Republic of Korea) shows a nearly modern avian ecosystem. Vertebrata Palasiatica, 52, 129–152. [Google Scholar]
92. Gatesy, S. M. (1990). Caudofemoral musculature and the evolution of theropod locomotion. Paleobiology, 16, 170–186. [Google Scholar]
93. Middleton, K. M., Gatesy, S. M. (2000). Theropod forelimb design and evolution. Zoological Journal of the Linnean Society, 128, 149–187. [Google Scholar]
94. Erdemir, A., McLean, S., Herzog, W., van den Bogert, A. J. (2007). Model-based estimation of muscle forces exerted during movements. Clinical Biomechanics, 22, 131–154 [Google Scholar] [PubMed]
95. Lin, Y. C., Dorn, T. W., Schache, A. G., Pandy, M. G. (2012). Comparison of different methods for estimating muscle forces in human movement. Proceedings of the Institution of Mechanical Engineers, Part H: Journal of Engineering in Medicine, 226, 103–112 [Google Scholar] [PubMed]
96. Anderson, F. C., Pandy, M. G. (2001). Static and dynamic optimization solutions for gait are practically equivalent. Journal of Biomechanics, 34, 153–161 [Google Scholar] [PubMed]
97. Rankin, J. W., Rubenson, J., Hutchinson, J. R. (2016). Inferring muscle functional roles of the ostrich pelvic limb during walking and running using computer optimization. Journal of the Royal Society Interface, 13, 20160035 [Google Scholar] [PubMed]
98. Bates, K. T., Manning, P. L., Margetts, L., Sellers, W. I. (2010). Sensitivity analysis in evolutionary robotic simulations of bipedal dinosaur running. Journal of Vertebrate Paleontology, 30, 458–466. [Google Scholar]
99. Sellers, K. C., Middleton, K. M., Davis, J. L., Holliday, C. M. (2017). Ontogeny of bite force in a validated biomechanical model of the American alligator. Journal of Experimental Biology, 220, 2036–2046 [Google Scholar] [PubMed]
100. Morgan, E. F., Bouxsein, M. L. (2005). Use of finite element analysis to assess bone strength. BoneKEy-Osteovision, 2, 8–19. [Google Scholar]
101. Richmond, B. G., Wright, B. W., Grosse, I., Dechow, P. C., Ross, C. F. et al. (2005). Finite element analysis in functional morphology. Anatomical Record, 283, 259–274. [Google Scholar]
102. Rayfield, E. J. (2007). Finite element analysis and understanding the biomechanics and evolution of living and fossil organisms. Annual Review of Earth and Planetary Sciences, 35, 541–576. [Google Scholar]
103. Marcé-Nogué, J., Püschel, T. A., Kaiser, T. M. (2017). A biomechanical approach to understand the ecomorphological relationship between primate mandibles and diet. Scientific Reports, 7, 8364. [Google Scholar]
104. Wei, X., Zhang, Z. (2021). Femoral mechanical performance of precocial and altricial birds: A simulation study. Avian Research, 12, 18. [Google Scholar]
Cite This Article
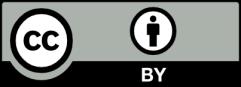