Open Access
REVIEW
Opportunities and challenges of CD47-targeted therapy in cancer immunotherapy
1 Key Laboratory for Translational Medicine, The First Affiliated Hospital, Huzhou University School of Medicine, Huzhou, 313000, China
2 Department of Hematology, Puyang Youtian General Hospital, Puyang, 457001, China
3 Department of Medicine, Moores Cancer Center, Sanford Stem Cell Institute, University of California San Diego, La Jolla, San Diego, 92093, USA
* Corresponding Authors: Qiuqiang Chen, ; Wenxue Ma,
(This article belongs to the Special Issue: Advances in Cancer Immunotherapy)
Oncology Research 2024, 32(1), 49-60. https://doi.org/10.32604/or.2023.042383
Received 28 May 2023; Accepted 09 August 2023; Issue published 15 November 2023
Abstract
Cancer immunotherapy has emerged as a promising strategy for the treatment of cancer, with the tumor microenvironment (TME) playing a pivotal role in modulating the immune response. CD47, a cell surface protein, has been identified as a crucial regulator of the TME and a potential therapeutic target for cancer therapy. However, the precise functions and implications of CD47 in the TME during immunotherapy for cancer patients remain incompletely understood. This comprehensive review aims to provide an overview of CD47’s multifaced role in TME regulation and immune evasion, elucidating its impact on various types of immunotherapy outcomes, including checkpoint inhibitors and CAR T-cell therapy. Notably, CD47-targeted therapies offer a promising avenue for improving cancer treatment outcomes, especially when combined with other immunotherapeutic approaches. The review also discusses current and potential CD47-targeted therapies being explored for cancer treatment and delves into the associated challenges and opportunities inherent in targeting CD47. Despite the demonstrated effectiveness of CD47-targeted therapies, there are potential problems, including unintended effects on healthy cells, hematological toxicities, and the development if resistance. Consequently, further research efforts are warranted to fully understand the underlying mechanisms of resistance and to optimize CD47-targeted therapies through innovative combination approaches, ultimately improving cancer treatment outcomes. Overall, this comprehensive review highlights the significance of CD47 as a promising target for cancer immunotherapy and provides valuable insight into the challenges and opportunities in developing effective CD47-targeted therapies for cancer treatment.Graphical Abstract
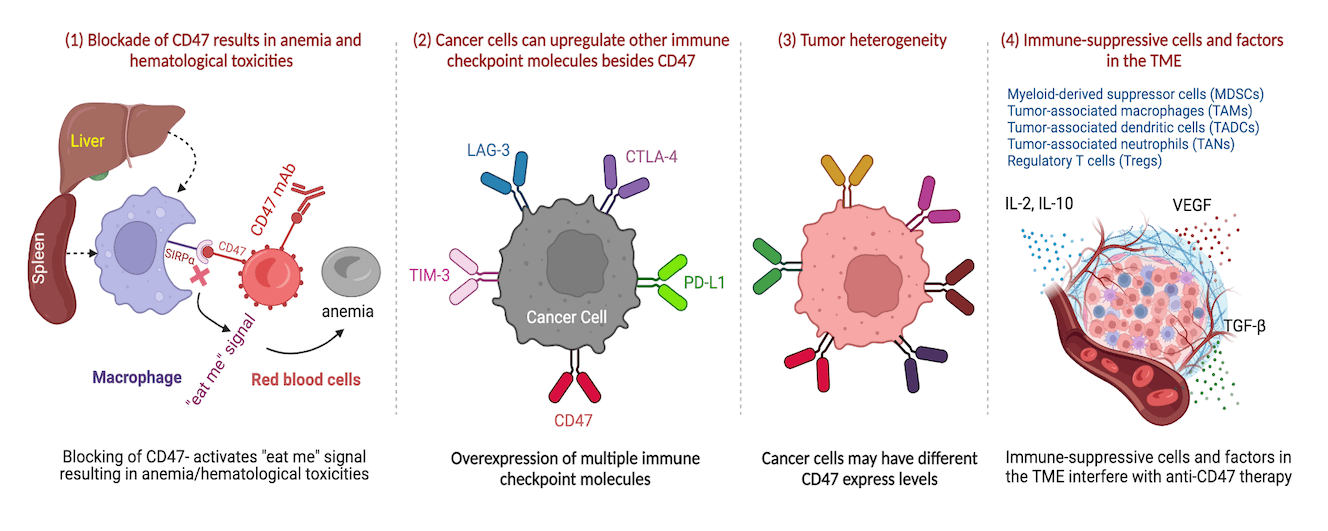
Keywords
Cancer remains a significant global and public health challenge, necessitating the pursuit of new approaches to address its complexities and unanswered questions [1]. While traditional cancer treatments have improved patient outcomes, they often come with significant side effects and limited efficacy for certain cancer types. In recent years, immunotherapy has emerged as a promising alternative, particularly for patients with advanced or metastatic cancers resistant to traditional treatments like chemotherapy and radiation therapy. Immunotherapy leverages the immune system’s power to recognize and eliminate cancer cells, providing more targeted and effective cancer treatment options with fewer side effects [2].
While immunotherapy has demonstrated remarkable success in some cancer patients, but response rates vary, and not all individuals benefit from current treatments. The TME, consisting of various cells, molecules, and factors that interact with the tumor, playing a pivotal role in shaping the immune response to cancer. Within the TME, cancer cells employ various strategies to evade immune surveillance, such as expressing immune checkpoint proteins like programmed death ligand-1 (PD-L1), which suppress their activity by binding to receptors on their surface [3]. Furthermore, the TME can foster an immunosuppressive environment that promotes the development of regulatory immune cells, including regulatory T cells (Tregs), which inhibit anti-tumor immune responses [4]. Thus, the TME exerts a crucial role in the growth and progression of cancer cells [5,6].
CD47, a cell surface protein expressed on multiple cell types, including cancer cells, acts as a key regulator of the TME and represents a potential target for cancer therapy [7,8]. The interaction between CD47 on cancer cells and signal regulatory protein alpha (SIRPα) on myeloid cells transmits a signal that prevents the cancer cells from immune detection and phagocytosis, allowing them to evade immune clearance and proliferate unchecked [9,10]. In recent years, CD47 blockade has emerged as a potential therapeutic strategy in cancer immunotherapy [10]. By blocking CD47, the “don’t eat me” signal is disrupted, enabling phagocytic immune cells to recognize and eliminate cancer cells [11]. Preclinical studies have demonstrated that CD47 blockade enhances the phagocytosis of cancer cells by macrophages and promote anti-tumor immune responses [12]. Various CD47-targeted therapies, including monoclonal antibodies (mAbs), small molecule inhibitors, and nanotechnology-based delivery systems are currently under development [13–16]. Promising results have been observed in preclinical studies and early-phase clinical trials, positioning CD47-targeted therapy as a potential breakthrough in cancer immunotherapy [12,17].
The success of immunotherapy approaches, such as immune checkpoint inhibitors (ICIs) and CD47-CAR-T cell therapy, relies on a favorable TME, characterized by the presence of immune cells and the absence of immunosuppressive factors [18,19]. However, the functions and implications of CD47 in the TME of cancer patients undergoing immunotherapy are still not fully elucidated. In addition, there are potential limitations and challenges associated with CD47-targeted therapy, including off-target effects and resistance mechanisms [20–22].
This review aims to provide a comprehensive overview of CD47’s role in the TME of cancer patients receiving immunotherapy. It will explore its interactions with other TME components, its impact on various immunotherapy outcomes, and the potential implications of CD47-targeted therapies in cancer treatment. By enhancing our understanding CD47’s role in the TME, further research can optimize the design and delivery of CD47-targeted therapies for cancer patients, ultimately improving treatment outcomes.
CD47 Function in the TME and Signaling Pathways
The TME encompasses a diverse array of cellular and non-cellular components, including blood vessels, immune cells, fibroblasts, extracellular matrix (ECM), and signaling molecules. These elements collectively influence the growth, survival, metastasis, and therapeutic response of cancer cells. Within the TME, various factors provide growth signals to cancer cells, suppress immune responses, and promote angiogenesis [23]. Therefore, understanding the components and interactions within the TME is critical for the development of effective cancer treatments.
Cellular components within the TME consist of cancer cells, stromal cells, and immune cells. Cancer cells engage in interaction with other TME components to promote their survival, growth, and invasive potential. Stromal cells, including cancer-associated fibroblasts (CAFs), endothelial cells, and pericytes, not only provide structural support and nutrients to tumor cells but also contribute to immunosuppression and angiogenesis [24]. Immune cells, such as T cells, B cells, natural killer (NK) cells, and myeloid-derived suppressor cells (MDSCs), exert either pro- or anti-tumor effects depending on their phenotype and activation state [25].
The ECM of the TME comprises a complex network of proteins, including collagens, fibronectin, laminin, and proteoglycans [26]. The ECM proteins provide physical and chemical cues that regulate cancer cell behavior and facilitate invasion and metastasis [27]. Additionally, the ECM influences the infiltration and activation of immune cells within the TME [28]. Alongside cellular and ECM constituents, a diverse range of signaling molecules, such as growth factors, cytokines, and chemokines, are present in the TME [29,30]. These molecules impact cancer cell behavior and immune cell activity and can be secreted by both cancer and stromal cells [31,32].
Overall, the TME constitutes a highly intricate and dynamic system consisting of a variety of cellular and non-cellular components. The intricate interactions among these components play a crucial role in regulating tumor growth, survival, and response to therapy, highlighting the critical importance of understanding TME for the development of effective cancer treatments.
The role of CD47 in TME regulation and immune evasion
CD47 act as a high-affinity signaling receptor for the secreted matrix protein thrombospondin-1 (TSP1) and serves as the counter-receptor for signal regulatory protein-α (SIRPα). SIRPα is expressed on various myeloid cells, including macrophages, and dendritic cells (DCs) [33,34]. Additionally, CD47 interacts with multiple integrins, regulating integrin activation on various immune cells [35,36]. The binding of CD47-SIRPα inhibits macrophage phagocytosis of CD47-expressing cells, generating a “don’t eat me” signal and facilitating immune evasion by cancer cells [11,37,38].
CD47 is not only expressed on cancer cells but also stromal cells, including endothelial cells, fibroblasts, and immune cells within the TME [39,40]. CD47 expression on stromal cells contributes not only contribute to angiogenesis and tumor growth but also to immune evasion [7,41,42]. For instance, CD47 on endothelial cells inhibits T cell trafficking into the TME, while CD47 on fibroblasts promotes immunosuppressive functions of Treg [43]. Therefore, CD47 expression on stromal cells significantly impacts tumor growth and progression. CD47 blockade not only enhances macrophage-mediated phagocytosis of cancer cells but also directly affects stromal cell function and the TME. The dual effects make CD47 a promising therapeutic target for cancer immunotherapy.
Furthermore, CD47 interacts with TSP-1, an extracellular matrix protein expressed in the TME [44]. TSP 1 exerts inhibitory effects on angiogenesis through multiple mechanisms, including the stimulation of endothelial cell apoptosis, inhibition of endothelial cell migration and proliferation, and regulation of vascular endothelial growth factor (VEGF) bioavailability and activity [45]. Recent studies have demonstrated that TSP-1 plays a role in promoting dormancy by initially suppressing angiogenesis, which is a critical early step in tumor progression [46]. Notably, studies in preclinical models have demonstrated that CD47-TSP-1 interactions play a regulatory role in angiogenesis and tumor growth [8,47,48]. The findings from studies conducted in preclinical models provide strong evidence for the regulatory role of CD47-TSP-1 interactions in angiogenesis and tumor growth. These studies highlight the significance of CD47-TSP-1 interactions in the modulation of angiogenesis and tumor growth. Consequently, targeting CD47-TSP-1 interactions holds therapeutic potential for controlling angiogenesis and inhibiting tumor growth in cancer treatment, based on these compelling findings. Furthermore, CD47 expression is associated with a poor prognosis in various cancers [41,49], suggesting its involvement in tumor progression and metastasis.
Given its roles immune evasion and TME regulation, CD47 has emerged as a promising therapeutic target for cancer treatment. CD47-targeted therapies, including mAbs, small molecule inhibitors, and nanotherapeutics, have been developed to block the CD47-SIRPα interaction and enhance phagocytosis of cancer cells by myeloid cells, thereby promoting anti-tumor immunity (Fig. 1) [50,51]. Preclinical and early-stage clinical studies have demonstrated promising results for CD47-targeted therapies, both as monotherapy and in combination with other immunotherapies [17,52,53].
Figure 1: CD47-SIRPα blockade in activating the “don’t eat me” signal and enhancing anti-tumor immunity. (A) The interaction between CD47, expressed on the surface of cancer cells, and SIRPα on macrophages sends a “don’t eat me” signal, inhibiting phagocytosis of the cancer cell by the macrophage. This binding of CD47 and SIRPα allows cancer cells to evade detection by macrophages, which perceive them as “self” and do not attack them. (B) Disrupting the CD47-SIRPα interaction between cancer cells and macrophages promotes phagocytosis. Treatment with either anti-CD47 or anti-SIRPα mAbs blocks the CD47-SIRPα interaction, leading to the activation of an “eat me” signal that enables macrophages to recognize cancer cells as non-self and initiates phagocytosis. Targeted therapies that disrupt the CD47-SIRPα interaction have the potential to improve the efficacy of cancer immunotherapy by enabling macrophages to overcome the “don’t eat me” signal and phagocytose cancer cells.
In summary, CD47 plays a critical role in regulating the TME and evading the immune system, making it an attractive therapeutic target for cancer treatment. CD47 blockade not only enhances the macrophage phagocytosis of cancer cells but also directly impacts stromal cell function and the TME. These dual effects highlight the potential of CD47 as a promising therapeutic target for cancer immunotherapy.
Regulation of immune cell infiltration by CD47 in the TME
CD47 plays a crucial role in regulating immune cell infiltration into the TME [41]. As mentioned earlier, the interaction between CD47 and its receptor SIRPα on macrophages inhibits the phagocytosis of cancer cells. However, this interaction can also affect other immune cells. When CD47 is expressed on endothelial cells, it can prevent T cell trafficking into the TME by inhibiting the expression of adhesion molecules such as Intercellular Adhesion Molecule 1 (ICAM-1) and vascular cell adhesion molecule 1 (VCAM-1), which are essential for T cell adhesion and migration across the endothelium [54]. This can lead to a decreased anti-tumor immune response and increased immune evasion [55,56].
Furthermore, CD47 expressed on cancer cells and stromal cells can also interact with SIRPα on MDSCs and inhibit their activation and differentiation into mature macrophages and DCs. This, in turn, results in the accumulation of immunosuppressive MDSCs in the TME, which further inhibits the anti-tumor immune response and promote tumor growth and progression [57]. Additionally, CD47 expression on Tregs has been shown to regulate their function. When CD47 is expressed on fibroblasts, it can activate Tregs and promote their immunosuppressive function, leading to a reduced anti-tumor immune response [58].
In summary, CD47 expression on cancer cells and stromal cells can regulate immune cell infiltration into the TME by inhibiting the expression of adhesion molecules and promoting the accumulation of immunosuppressive cells such as MDSCs and Tregs. Consequently, this leads to a reduced anti-tumor immune response and increased immune evasion. Therefore, targeting CD47 may represent a promising strategy to enhance immune cell infiltration and overcome immune evasion in the TME.
CD47-mediated signaling between cancer and stromal cells
CD47-mediated signaling plays a critical role in regulating tumor growth and progression by facilitating communication between cancer cells and stromal cells in the TME. It interacts with TSP-1, which is expressed on stromal cells such as endothelial cells and fibroblasts in the TME [44]. This interaction can potentially influence downstream signaling events that involve Akt/mTOR and NF-κB pathways, which promote tumor cell survival, invasion, and angiogenesis while inhibiting immune cell function. In preclinical studies, inhibiting CD47-TSP-1 signaling has been shown to reduce tumor cell survival and invasion [59].
CD47-mediated signaling is complex, highlighting its potential as a therapeutic target for cancer treatment. Targeting CD47-mediated signaling may represent a promising strategy for cancer immunotherapy by disrupting the communication between cancer cells and stromal cells in the TME [60]. Overall, the diverse signaling pathways activated by CD47 underscore its importance in regulating cellular responses and its potential as a therapeutic target for cancer treatment. The Table 1 below summarizes the signaling pathways activated by CD47 in cancer cells. CD47 interacts with several receptors and molecules, including TSP-1 and SIRPα, to activate downstream pathways such as Akt/mTOR, NF-κB, PI3K/Akt, RhoA/ROCK, and others, to regulate cellular processes such as tumor cell survival, invasion, angiogenesis, cell migration, and apoptosis.
CD47 is an overexpressed protein on many cancer cells that plays a critical role in regulating immune cell interactions within the TME. By interacting with immune checkpoint receptors and SIRPα on immune cells, CD47 can suppress anti-tumor immunity and promote immune evasion [50,60]. Immunotherapies, including ICIs, CAR T-cell therapy, and antibody-based therapies, are being developed to target CD47 or its interactions with other TME components to enhance anti-tumor immunity and improve clinical outcomes. Combining CD47-targeted immunotherapy with other immunotherapies has shown promise in further enhancing response rates. Thus, there is a growing interest in developing combination therapies targeting multiple components of the TME, including CD47.
Types of immunotherapy relevant to CD47
Immunotherapy is a promising cancer treatment that leverages the immune system to combat cancer. CD47, which is expressed on numerous cancer cell types, presents an attractive target for CAR T-cell therapy. CD47 has been found to play a role in various forms of immunotherapy. Checkpoint inhibitors can hinder CD47’s interaction with checkpoint receptors like programmed death-1 (PD-1) and T-cell immunoglobulin and mucin-domain containing-3 (TIM-3), bolstering anti-tumor immunity and potentially enhancing clinical outcomes in cancer patients [66]. CAR T-cell therapy can target CD47 by genetically modifying a patient’s T cells to recognize and attack cancer cells. Several preclinical studies have investigated the efficacy of chimeric antigen receptor (CAR) T-cells targeting CD47 in eliminating cancer cells. Results from in vitro and in vivo studies have consistently shown that CAR T-cells that CD47 targeted CAR T-cells can efficiently eliminate cancer cells while sparing normal cells [67,68].
Several clinical trials are currently evaluating the safety and efficacy of CD47-targeted CAR T-cell therapy in cancer patients, along with pre-clinical studies. According to the information available on https://www.clinicaltrials.gov, currently, there are 13 ongoing CD47 trials in China (data not shown), and an additional 29 clinical trials targeting CD47 for cancer immunotherapy are underway in the United States (data not shown).
Evidence of the impact of CD47 expression on immunotherapy outcomes
Studies have extensively investigated the impact of CD47 expression on immunotherapy outcomes in cancer patients. High levels of CD47 expression on cancer cells have consistently correlated with poor clinical response rates and worse overall survival (OS) in patients receiving immunotherapy [12,19]. This suggests that CD47 may serve as an important biomarker for predicting response to immunotherapy [49,69]. Preclinical studies have demonstrated that blocking CD47 can enhance the efficacy of immunotherapy by enhancing immune cell infiltration and inducing tumor cell death [70]. For instance, combination therapies incorporating CD47 blockade and checkpoint inhibitors have exhibited improved anti-tumor immunity and enhanced tumor regression [71]. Additionally, CD47-targeted CAR T-cell therapy has demonstrated potent anti-tumor effects in preclinical models [68,72]. Nevertheless, further research is necessary to validate the clinical relevance of CD47 as a predictive biomarker for the response to immunotherapy and to optimize the use of CD47-targeted therapies in combination with other immunotherapies [69].
CD47 has emerged as a potential biomarker in cancer research, displaying distinct expression patterns in various malignancies [12,73,74]. Elevated levels of CD47 expression have been consistently observed across multiple cancer types, encompassing solid tumors and hematological malignancies [7,12,49,60,75]. For instance, Huang et al. emphasized the importance of cautiously selecting of patients for anti-CD47 therapy based on CD47 expression levels and clustering status [42]. Additionally, Additionally, Jiang et al. conducted a comprehensive analysis of CD47-related clinical research, including 23 therapeutic agents with 46 clinical trials listed in the NCT registry platform. Among these trials, 29 were focused on solid tumors, 14 on hematological malignancies, and 3 on both solid tumors and hematological malignancies. Various anti-CD47 mAbs were investigated with diverse strategies in these clinical trials, and patient enrollment was often guided by their CD47 expression levels [12]. Upregulation of CD47 on cancer cells has consistently been associated with immune evasion, tumor progression, and poor prognosis [76–78]. Furthermore, CD47 expression on both tumor cells and stromal cells within the TME has been implicated in promoting immune suppression and conferring resistance to the immunotherapy [79,80]. As a biomarker, CD47 holds promise in predicting therapeutic responses and guiding treatment strategies [75,81,82]. Further investigations are required to elucidate the specific mechanisms underlying CD47-mediated immune evasion and its potential as a predictive biomarker for immunotherapy outcomes. An enhanced understanding the role of CD47 as a biomarker may pave the way for developing personalized therapeutic approaches targeting CD47 in cancer patients.
Implication of CD47 in Cancer Immunotherapy
CD47-targeted therapies, including antibody-based therapies, CAR T-cell therapy, and combination therapies, have shown promise in enhancing anti-tumor immunity and improving clinical outcomes.
CD47-targeted immunotherapies for cancer
CD47-targeted immunotherapy approaches aim to enhance anti-tumor immunity and improve clinical outcomes by disrupting the “don’t eat me” signal between CD47 (on cancer cells) and SIRPα (on myeloid cells). One approach is to use blocking antibodies or small molecules that inhibit this interaction, leading to increased phagocytosis of cancer cells by macrophages and promoting an anti-tumor immune response. CD47-blocking antibodies such as Hu5F9-G4 and CC-90002 are currently being evaluated in clinical trials for various solid tumors and hematological malignancies [52,83]. Combining CD47 inhibitors with PD-1/PD-L1 inhibitors or cytotoxic T-lymphocyte–associated antigen 4 (CTLA-4) inhibitors may enhance the anti-tumor immune response by promoting T cell activation and reducing immune suppression in the TME [84]. Additionally, some studies have explored the use of engineered immune cells that express a modified SIRPα receptor with higher binding affinity for CD47 or CD47-targeted CAR T-cell therapy to directly kill cancer cells and enhance the anti-tumor immune response [68,85].
CD47 is often overexpressed on cancer cells, acting as a “don’t eat me” signal that allows cancer cells to evade detection by the immune system. To combat this, a variety of CD47-targeted therapies are being developed. Magrolimab is currently the most advanced CD47-targeted therapy in development, with ongoing clinical trials for several types of cancer, including acute myeloid leukemia (AML) and solid tumors [86–88]. This mAb blocks the CD47-SIRPα interaction, leading to increased phagocytosis of cancer cells. Other CD47-targeted therapies currently in development include bispecific antibodies like TTI-621, which target both CD47 and other immune cell surface markers, as well as small molecule inhibitors that disrupt the CD47-SIRPα interaction. Gene therapies are also being explored as potential cancer treatment [89]. Combination therapies that target both CD47 and other immune checkpoints, such as PD-1 and CTLA-4, are also being studied to enhance anti-tumor immunity [12,49,90].
CD47-targeted therapies show promise in both preclinical and clinical studies, with the potential to improve outcomes for cancer patients. However, further research is needed to optimize these therapies and identify biomarkers that can predict response to CD47-targeted treatments.
Potential biomarkers for predicting response to CD47-targeted therapy
Identifying biomarkers that can predict response to CD47-targeted therapy is crucial for selecting patients who are most likely to benefit from this treatment approach. Several potential biomarkers are currently under investigation, including (1) CD47 and SIRPα expression levels: Higher levels of CD47 expression in tumors may indicate a higher resistance to CD47-targeted therapy, while higher levels of SIRPα expression may suggest greater sensitivity to this therapy [50]. Measuring the expression levels of both CD47 and SIRPα in tumors may help identify patients who are more likely to respond to CD47 blockade. (2) Tumor-infiltrating immune cells: The presence of certain immune cell types, such as macrophages and T cells, in the TME may be associated with response to CD47-targeted therapy. Therefore, measuring the presence of these immune cell types in tumors may help identify patients who are more likely to respond to CD47 blockade. (3) Immune checkpoint expression levels: A novel affinity-tuned bispecific antibody targeting CD47 and PD-L1 was developed to antagonize both innate and adaptive immune checkpoint pathways in tumors with higher expression levels of immune checkpoint molecules, such as PD-1 [91]. Therefore, measuring immune checkpoint expression levels in tumors may help identify patients who are more likely to respond to combination therapy with CD47 blockade and checkpoint inhibitors. (4) Tumor mutational burden (TMB): Tumors with a higher TMB may be more responsive to CD47-targeted therapy, as they may have a higher number of neoantigens that can be recognized by the immune system [49,92]. Therefore, measuring TMB in tumors may help identify patients who are more likely to respond to CD47 blockade.
In summary, CD47 expression levels, SIRPα expression levels, tumor-infiltrating immune cells, immune checkpoint expression levels, and TMB are potential biomarkers that can help predict response to CD47-targeted therapy. Identifying patients who are most likely to benefit from this treatment approach can improve treatment outcomes while minimizing the risk of unnecessary side effects.
Challenges and opportunities of CD47-targeted therapies
CD47-targeted therapies have shown potential in cancer immunotherapy, but they also present challenges that need to be addressed. One challenge is that CD47 is not only expressed on cancer cells but also on healthy cells, which may lead to unintended effects of therapy. CD47 blockade can cause anemia and other hematological toxicities [69,93,94] due to the phagocytosis of healthy red blood cells, which express CD47 [95] as a “don’t eat me” signal to prevent their destruction by macrophages in the spleen and liver. The degree of toxicity can depend on the dose, timing, and baseline health status of the patient. Strategies to mitigate these toxicities include dose optimization, co-administration with erythropoietin, and the use of modified or alternative CD47-targeting agents [86].
Another challenge is that CD47 is not the only checkpoint molecule involved in immune evasion. Other checkpoint molecules, such as PD-L1, CTLA-4, lymphocyte-activation gene 3 (LAG-3), TIM-3, and indoleamine 2,3-dioxygenase (IDO), also play a role in suppressing the immune response to tumors [96]. Targeting these molecules, either alone or in combination with CD47, has shown promise in preclinical and clinical studies [78]. Therefore, targeting CD47 alone may not be sufficient for a durable anti-tumor response.
Resistance to CD47-targeted therapies has been observed, highlighting the need for combination approaches and further research to fully understand the mechanisms of resistance [97]. In addition to CD47, cancer cells may upregulate other immune checkpoint molecules such as PD-L1, CTLA-4, LAG-3, and TIM-3 to evade immune surveillance and escape destruction by immune cells [78]. Tumor heterogeneity is also a challenge of CD47-targeted therapies, as some cancer cells may have varying expression levels of CD47, which can lead to differences in susceptibility to CD47-targeted therapies among different cells [9,98].
Moreover, the presence of immune-suppressive cells, including MDSCs [99], tumor-associated macrophages (TAMs) [100,101], tumor-associated dendritic cells (TADCs) [102], and tumor-associated neutrophils (TANs) [103] may upregulate other immune checkpoint molecules (i.e., PD-L1, CTLA-4, LAG-3, TIM-3, etc.) to evade immune surveillance and escape immune evasion dendritic cells (TADCs), tumor-associated neutrophils (TANs) and Treg, the immune-suppressive factors in the TME such as cytokines (e.g., IL-2, IL-10, TGF-b, VEGF, etc.) can also interfere with the effectiveness of CD47-targeted therapies [104]. All these challenges and resistance are summarized in Fig. 2.
Figure 2: CD47-targeted therapies present challenges and potential unintended effects due to the expression of CD47 on healthy cells. CD47 blockade can cause anemia and hematological toxicities. However, CD47 is not the only immune checkpoint molecule involved in immune evasion, and targeting other molecules such as PD-L1, CTLA-4, LAG-3, TIM-3, and IDO, either alone or in combination with CD47, has shown promise in preclinical and clinical studies. Resistance to CD47-targeted therapies has also been observed, and tumor heterogeneity and the presence of immune-suppressive cells and factors in the TME can interfere with the effectiveness of CD47-targeted therapies. Therefore, combination approaches and further research are needed to fully understand the mechanisms of resistance.
To overcome these challenges, several strategies are being investigated. One approach is to combine CD47-targeted therapies with other immune checkpoint inhibitors, such as anti-PD-1/PD-L1 antibodies, to overcome the immune evasion mechanisms employed by tumor cells [12,105]. Another approach is to develop bispecific antibodies that can target both CD47 and another immune checkpoint molecule simultaneously [12,91,106]. Additionally, researchers are exploring ways to modulate the TME to enhance the efficacy of CD47-targeted therapies [60,107,108]. These strategies hold promise for improving the outcomes of CD47-targeted therapies and overcoming resistance in patients.
Despite the challenges, CD47-targeted therapies present opportunities for improving cancer treatment. These therapies have the potential to enhance anti-tumor immunity by promoting phagocytosis of cancer cells and reducing immune suppression in the TME [7,50,58,77]. CD47-targeted therapies may also work synergistically with other immunotherapies, such as checkpoint inhibitors, to further enhance anti-tumor immunity and improve clinical outcomes [17,109]. CD47 is expressed on many different types of cancer cells, making it a potentially broad target for cancer treatment.
In summary, CD47-targeted therapies offer a promising opportunity to enhance anti-tumor immunity and improve clinical outcomes in cancer patients. Overcoming challenges such as resistance mechanisms [22] and off-target effects [50], while identifying biomarkers that predict treatment response [44], will be essential to optimizing these therapies. Strategies to mitigate toxicities and improve efficacy, such as combination approaches and modulation of the tumor microenvironment, are currently being investigated and hold promise for improving outcomes in patients.
Clinical Trials and Future Directions
Current CD47-targeted clinical trials in cancer patients
CD47-targeted therapies are being evaluated in clinical trials for various cancer types. Several ongoing clinical trials investigating CD47-targeted therapies in cancer patients include: (1) Magrolimab (Hu5F9-G4) is a mAb that targets CD47. It is currently being evaluated in several clinical trials, including a phase III trial for patients with previously untreated higher-risk myelodysplastic syndrome (MDS) and a phase II trial for patients with acute myeloid leukemia (AML) [110,111]. Magrolimab is also being evaluated in combination with other therapies, such as azacitidine and venetoclax, for the treatment of AML and MDS [112,113]. (2) CC-90002 is a humanized anti-CD47 antibody that inhibits CD47-SIRPα interaction and enables macrophage-mediated killing of tumor cells in hematological cancer cell lines [83,114]. The results indicate the potential effectiveness of CC-90002 in treating various hematological malignancies, forming the rationale for conducting clinical trials such as CC-90002-ST-001 (NCT02367196) and CC-90002-AML-001 (NCT02641002). (3) TTI-622 is a bispecific antibody that targets CD47 and CD19. It is currently being evaluated in a phase I trial for patients with relapsed or refractory B-cell lymphoma and chronic lymphocytic leukemia (CLL) [115]. (4) ALX148 is a fusion protein that targets CD47 and is currently being evaluated in several clinical trials, including a phase I/II trial for patients with advanced solid tumors [17] and a phase I trial for patients with relapsed or refractory non-Hodgkin’s lymphoma [52]. (5) KSI-3716 is a mAb that targets CD47 and is currently being evaluated in phase I/II trials for patients with advanced solid tumors [116]. (6) AO-176 is a mAb that targets both CD47 and CD19. It is currently being evaluated in a phase I trial for patients with relapsed or refractory B-cell lymphoma [12,85].
Overall, these clinical trials are testing the safety and efficacy of CD47-targeted therapies in various cancer types and stages. The results of these trials will provide valuable insights into the potential of CD47-targeted therapies for cancer treatment.
Limitations of CD47-targeted therapies
While CD47-targeted therapy has shown promise as a potential cancer treatment, there are several limitations that need to be addressed. These limitations include resistance mechanisms, toxicity, lack of predictive biomarkers, inadequate effectiveness as a monotherapy, and production difficulties.
One significant limitation is that tumor cells can develop resistance to CD47-targeted therapy through various mechanisms, such as upregulating alternative phagocytic checkpoints or increasing expression of CD47 on the surface of tumor cells [53]. Additionally, tumor cells can alter the TME to be less favorable to phagocytosis. CD47 is expressed on many healthy cells, so targeting CD47 can lead to unintended damage to healthy tissues. This can cause side effects such as anemia, thrombocytopenia, and immune-related adverse events [12].
Another limitation is the lack of validated biomarker to predict which patients will respond to CD47-targeted therapy [44]. This makes it challenging to select patients who are most likely to benefit from this treatment approach. CD47-targeted therapy may have limited efficacy as a monotherapy, especially in solid tumors, due to the complex and heterogeneous nature of the TME. Combination therapies with other immune checkpoint inhibitors or targeted therapies may be necessary to enhance the efficacy of CD47-targeted therapy. Developing CD47-targeted therapies can be challenging due to the complexity of the molecule and the difficulty in manufacturing large quantities of high-quality mAbs or bispecific antibodies.
In summary, CD47-targeted therapy has shown promise as a potential cancer treatment, but there are several limitations and challenges that need to be addressed. Addressing these challenges will be critical in advancing CD47-targeted therapy toward becoming a more effective treatment option for cancer patients.
Future directions and potential for CD47-targeted therapy in cancer treatment
CD47-targeted therapy is a rapidly evolving and active area of research with many potential future directions. Combination therapies with other immunotherapies, such as ICIs, as well as the development of bispecific antibodies that target CD47 and another immune checkpoint, hold promise for enhancing the efficacy of treatment. Identifying predictive biomarkers and improving the manufacturing process can help select patients who will benefit from this treatment and make its production more efficient and cost-effective. Moreover, targeting stromal cells and combining CD47-targeted therapy with other targeted therapies such as tyrosine kinase inhibitors or PARP inhibitors may enhance its efficacy in specific cancer types. Expanding the use of CD47-targeted therapy beyond hematological malignancies to other cancer types is also a future direction for research. While combining multiple therapies can enhance treatment efficacy, it is important to consider the potential for increased adverse events. The occurrence of adverse events in combination therapy can vary and depends on several factors, including the specific therapies used, their mechanisms of action, and the patient population being treated. It is crucial to carefully evaluate the safety and tolerability of combination regimens through rigorous clinical trials. By conducting comprehensive studies, researchers aim to identify optimal combination strategies that maximize treatment benefits while minimizing the risk of adverse events.
CD47-targeted therapy offers a promising approach to improving cancer treatment outcomes by blocking CD47 and helping immune cells find and destroy cancer cells. However, further research is needed to fully understand its potential in cancer treatment, including the identification of biomarkers that can predict response to these therapies, evaluation of their potential benefits and risks, and development of guidelines for appropriate use. Additionally, continued research is important for optimizing their efficacy and safety in different cancer types and patient populations, enhancing the efficacy of other cancer treatments, and reducing costs to make CD47-targeted therapy more accessible to patients. Clinical trials are necessary to assess their potential benefits and risks, determine the optimal dosing and treatment regimen, and evaluate their safety and efficacy.
Acknowledgement: This paper represents the authors’ interpretation of the relevant and latest publications and existing literature on the topic.
Funding Statement: This work was supported by a grant from the Huzhou Science and Technology Bureau, Zhejiang Province, China (2020GZ41).
Author Contributions: Conceptualization, writing, and editing: QC, XG, and WM. All authors have read and agreed to the published version of the manuscript.
Availability of Data and Materials: The data and material used in the current study are available from the corresponding author upon reasonable request.
Ethics Approval: Not applicable.
Conflicts of Interest: The authors declare that they have no conflicts of interest to report regarding the present study.
References
1. Broeders, M., Elfstrom, K. M. (2020). Importance of international networking and comparative research in screening to meet the global challenge of cancer control. JCO Glob Oncolology, 6, 180–181. https://doi.org/10.1200/JGO.19.00388 [Google Scholar] [PubMed] [CrossRef]
2. Waldman, A. D., Fritz, J. M., Lenardo, M. J. (2020). A guide to cancer immuNOTHERapy: From T cell basic science to clinical practice. Nature Reviews Immunology, 20(11), 651–668. https://doi.org/10.1038/s41577-020-0306-5 [Google Scholar] [PubMed] [CrossRef]
3. Kim, M. H., Kim, C. G., Kim, S. K., Shin, S. J., Choe, E. A. et al. (2018). YAP-induced PD-L1 expression drives immune evasion in BRAFi-resistant melanoma. Cancer Immunology Research, 6(3), 255–266. https://doi.org/10.1158/2326-6066.CIR-17-0320 [Google Scholar] [PubMed] [CrossRef]
4. Itahashi, K., Irie, T., Nishikawa, H. (2022). Regulatory T-cell development in the tumor microenvironment. European Journal of Immunology, 52(8), 1216–1227. https://doi.org/10.1002/eji.202149358 [Google Scholar] [PubMed] [CrossRef]
5. Labani-Motlagh, A., Ashja-Mahdavi, M., Loskog, A. (2020). The tumor microenvironment: A milieu hindering and obstructing antitumor immune responses. Frontiers in Immunology, 11, 940. https://doi.org/10.3389/fimmu.2020.00940 [Google Scholar] [PubMed] [CrossRef]
6. Pena-Romero, A. C., Orenes-Pinero, E. (2022). Dual effect of immune cells within tumour microenvironment: Pro- and anti-tumour effects and their triggers. Cancers, 14(7), 1681. https://doi.org/10.3390/cancers14071681 [Google Scholar] [PubMed] [CrossRef]
7. Eladl, E., Tremblay-LeMay, R., Rastgoo, N., Musani, R., Chen, W. et al. (2020). Role of CD47 in hematological malignancies. Journal of Hematology & Oncology, 13(1), 96. https://doi.org/10.1186/s13045-020-00930-1 [Google Scholar] [PubMed] [CrossRef]
8. Kaur, S., Cicalese, K. V., Bannerjee, R., Roberts, D. D. (2020). Preclinical and clinical development of therapeutic antibodies targeting functions of CD47 in the tumor microenvironment. Antibody Therapeutics, 3(3), 179–192. https://doi.org/10.1093/abt/tbaa017 [Google Scholar] [PubMed] [CrossRef]
9. Cham, L. B., Adomati, T., Li, F., Ali, M., Lang, K. S. (2020). CD47 as a potential target to therapy for infectious diseases. Antibodies, 9(3), 44. https://doi.org/10.3390/antib9030044 [Google Scholar] [PubMed] [CrossRef]
10. Lin, F., Xiong, M., Hao, W., Song, Y., Liu, R. et al. (2020). A novel blockade CD47 antibody with therapeutic potential for cancer. Frontiers in Oncology, 10, 615534. https://doi.org/10.3389/fonc.2020.615534 [Google Scholar] [PubMed] [CrossRef]
11. Wang, H., Sun, Y., Zhou, X., Chen, C., Jiao, L. et al. (2020). CD47/SIRPα blocking peptide identification and synergistic effect with irradiation for cancer immunotherapy. Journal of Immunotherapy of Cancer, 8(2), e000905. https://doi.org/10.1136/jitc-2020-000905 [Google Scholar] [PubMed] [CrossRef]
12. Jiang, Z., Sun, H., Yu, J., Tian, W., Song, Y. (2021). Targeting CD47 for cancer immunotherapy. Journal of Hematology & Oncology, 14(1), 180. https://doi.org/10.1186/s13045-021-01197-w [Google Scholar] [PubMed] [CrossRef]
13. Liu, J., Meng, Z., Xu, T., Kuerban, K., Wang, S. et al. (2022). A SIRPαFc fusion protein conjugated with the collagen-binding domain for targeted immunotherapy of non-small cell lung cancer. Frontiers in Immunology, 13, 845217. https://doi.org/10.3389/fimmu.2022.845217 [Google Scholar] [PubMed] [CrossRef]
14. Tian, L., Xu, B., Teng, K. Y., Song, M., Zhu, Z. et al. (2022). Targeting Fc receptor-mediated effects and the “Don’t Eat Me” signal with an oncolytic virus expressing an anti-CD47 antibody to treat metastatic ovarian cancer. Clinical Cancer Research, 28(1), 201–214. https://doi.org/10.1158/1078-0432.CCR-21-1248 [Google Scholar] [PubMed] [CrossRef]
15. Upton, R., Banuelos, A., Feng, D., Biswas, T., Kao, K. et al. (2021). Combining CD47 blockade with trastuzumab eliminates HER2-positive breast cancer cells and overcomes trastuzumab tolerance. Proceedings National Academy of Sciences USA, 118(29), e2026849118. https://doi.org/10.1073/pnas.2026849118 [Google Scholar] [PubMed] [CrossRef]
16. Ozaniak, A., Smetanova, J., Bartolini, R., Rataj, M., Capkova, L. et al. (2023). A novel anti-CD47-targeted blockade promotes immune activation in human soft tissue sarcoma but does not potentiate anti-PD-1 blockade. Journal of Cancer Research and Clinical Oncology, 149(7), 3789–3801. https://doi.org/10.1007/s00432-022-04292-8 [Google Scholar] [PubMed] [CrossRef]
17. Dizman, N., Buchbinder, E. I. (2021). Cancer therapy targeting CD47/SIRPα. Cancers, 13(24), 6229. https://doi.org/10.3390/cancers13246229 [Google Scholar] [PubMed] [CrossRef]
18. Peterson, C., Denlinger, N., Yang, Y. (2022). Recent advances and challenges in cancer immunotherapy. Cancers, 14(16), 3972. https://doi.org/10.3390/cancers14163972 [Google Scholar] [PubMed] [CrossRef]
19. Behrens, L. M., van den Berg, T. K., van Egmond, M. (2022). Targeting the CD47-SIRPα innate immune checkpoint to potentiate antibody therapy in cancer by neutrophils. Cancers, 14(14), 3366. https://doi.org/10.3390/cancers14143366 [Google Scholar] [PubMed] [CrossRef]
20. Nigro, A., Ricciardi, L., Salvato, I., Sabbatino, F., Vitale, M. et al. (2019). Enhanced expression of CD47 is associated with off-target resistance to tyrosine kinase inhibitor gefitinib in NSCLC. Frontiers in Immunology, 10, 3135. https://doi.org/10.3389/fimmu.2019.03135 [Google Scholar] [PubMed] [CrossRef]
21. Weiskopf, K., Jahchan, N. S., Schnorr, P. J., Cristea, S., Ring, A. M. et al. (2016). CD47-blocking immunotherapies stimulate macrophage-mediated destruction of small-cell lung cancer. Journal of Clinical Investigation, 126(7), 2610–2620. https://doi.org/10.1172/JCI81603 [Google Scholar] [PubMed] [CrossRef]
22. Yang, Y., Yang, Z., Yang, Y. (2021). Potential role of CD47-directed bispecific antibodies in cancer immunotherapy. Frontiers in Immunology, 12, 686031. https://doi.org/10.3389/fimmu.2021.686031 [Google Scholar] [PubMed] [CrossRef]
23. Neophytou, C. M., Panagi, M., Stylianopoulos, T., Papageorgis, P. (2021). The role of tumor microenvironment in cancer metastasis: Molecular mechanisms and therapeutic opportunities. Cancers, 13(9), 2053. https://doi.org/10.3390/cancers13092053 [Google Scholar] [PubMed] [CrossRef]
24. Sun, H., Wang, X., Wang, X., Xu, M., Sheng, W. (2022). The role of cancer-associated fibroblasts in tumorigenesis of gastric cancer. Cell Death & Disease, 13(10), 874. https://doi.org/10.1038/s41419-022-05320-8 [Google Scholar] [PubMed] [CrossRef]
25. Yang, Y., Li, C., Liu, T., Dai, X., Bazhin, A. V. (2020). Myeloid-derived suppressor cells in tumors: From mechanisms to antigen specificity and microenvironmental regulation. Frontiers in Immunology, 11, 1371. https://doi.org/10.3389/fimmu.2020.01371 [Google Scholar] [PubMed] [CrossRef]
26. Henke, E., Nandigama, R., Ergun, S. (2019). Extracellular matrix in the tumor microenvironment and its impact on cancer therapy. Frontiers in Molecular Biosciences, 6, 160. https://doi.org/10.3389/fmolb.2019.00160 [Google Scholar] [PubMed] [CrossRef]
27. Cruz-Acuna, R., Vunjak-Novakovic, G., Burdick, J. A., Rustgi, A. K. (2021). Emerging technologies provide insights on cancer extracellular matrix biology and therapeutics. iScience, 24(5), 102475. https://doi.org/10.1016/j.isci.2021.102475 [Google Scholar] [PubMed] [CrossRef]
28. Simsek, H., Klotzsch, E. (2022). The solid tumor microenvironment-breaking the barrier for T cells: How the solid tumor microenvironment influences T cells. BioEssays, 44(6), e2100285. https://doi.org/10.1002/bies.202100285 [Google Scholar] [PubMed] [CrossRef]
29. Anderson, N. M., Simon, M. C. (2020). The tumor microenvironment. Current Biology, 30(16), R921–R925. https://doi.org/10.1016/j.cub.2020.06.081 [Google Scholar] [PubMed] [CrossRef]
30. Baghban, R., Roshangar, L., Jahanban-Esfahlan, R., Seidi, K., Ebrahimi-Kalan, A. et al. (2020). Tumor microenvironment complexity and therapeutic implications at a glance. Cell Communication and Signaling, 18(1), 59. https://doi.org/10.1186/s12964-020-0530-4 [Google Scholar] [PubMed] [CrossRef]
31. Mao, X., Xu, J., Wang, W., Liang, C., Hua, J. et al. (2021). Crosstalk between cancer-associated fibroblasts and immune cells in the tumor microenvironment: New findings and future perspectives. Molecular Cancer, 20(1), 131. https://doi.org/10.1186/s12943-021-01428-1 [Google Scholar] [PubMed] [CrossRef]
32. Morris, R. M., Mortimer, T. O., O’Neill, K. L. (2022). Cytokines: Can cancer get the message? Cancers, 14(9), 2178. https://doi.org/10.3390/cancers14092178 [Google Scholar] [PubMed] [CrossRef]
33. van Duijn, A., van der Burg, S. H., Scheeren, F. A. (2022). CD47/SIRPα axis: Bridging innate and adaptive immunity. Journal for ImmunoTherapy of Cancer, 10(7), e004589. https://doi.org/10.1136/jitc-2022-004589 [Google Scholar] [PubMed] [CrossRef]
34. Yang, J., Deresa, I., Ho, W. H., Long, H., Maslyar, D. et al. (2023). Al008 enhances myeloid antitumor function by inhibiting SIRPα signaling and activating Fc receptors. Journal of Immunology, 210(2), 204–215. https://doi.org/10.4049/jimmunol.2200157 [Google Scholar] [PubMed] [CrossRef]
35. Hayat, S. M. G., Bianconi, V., Pirro, M., Jaafari, M. R., Hatamipour, M. et al. (2020). CD47: Role in the immune system and application to cancer therapy. Cellular Oncology, 43(1), 19–30. https://doi.org/10.1007/s13402-019-00469-5 [Google Scholar] [PubMed] [CrossRef]
36. Kaur, S., Livak, F., Daaboul, G., Anderson, L., Roberts, D. D. (2022). Single vesicle analysis of CD47 association with integrins and tetraspanins on extracellular vesicles released by T lymphoblast and prostate carcinoma cells. Journal of Extracellular Vesicles, 11(9), e12265. https://doi.org/10.1002/jev2.12265 [Google Scholar] [PubMed] [CrossRef]
37. Logtenberg, M. E. W., Scheeren, F. A., Schumacher, T. N. (2020). The CD47-SIRPα immune checkpoint. Immunity, 52(5), 742–752. https://doi.org/10.1016/j.immuni.2020.04.011 [Google Scholar] [PubMed] [CrossRef]
38. Morrissey, M. A., Kern, N., Vale, R. D. (2020). CD47 ligation repositions the inhibitory receptor sirpa to suppress integrin activation and phagocytosis. Immunity, 53(2), 290–302.e6. https://doi.org/10.1016/j.immuni.2020.07.008 [Google Scholar] [PubMed] [CrossRef]
39. Xiao, Y., Yu, D. (2021). Tumor microenvironment as a therapeutic target in cancer. Pharmacology and Therapeutics, 221, 107753. https://doi.org/10.1016/j.pharmthera.2020.107753 [Google Scholar] [PubMed] [CrossRef]
40. Gao, L., Chen, K., Gao, Q., Wang, X., Sun, J. et al. (2017). CD47 deficiency in tumor stroma promotes tumor progression by enhancing angiogenesis. Oncotarget, 8(14), 22406–22413. https://doi.org/10.18632/oncotarget.9899 [Google Scholar] [PubMed] [CrossRef]
41. Yu, L., Ding, Y., Wan, T., Deng, T., Huang, H. et al. (2021). Significance of CD47 and its association with tumor immune microenvironment heterogeneity in ovarian cancer. Frontiers in Immunology, 12, 768115. https://doi.org/10.3389/fimmu.2021.768115 [Google Scholar] [PubMed] [CrossRef]
42. Huang, Y., Ma, Y., Gao, P., Yao, Z. (2017). Targeting CD47: The achievements and concerns of current studies on cancer immunotherapy. Journal of Thoracic Disease, 9(2), E168–E174. https://doi.org/10.21037/jtd.2017.02.30 [Google Scholar] [PubMed] [CrossRef]
43. Khantakova, J. N., Bulygin, A. S., Sennikov, S. V. (2022). The regulatory-T-cell memory phenotype: What we know. Cells, 11(10), 1687. https://doi.org/10.3390/cells11101687 [Google Scholar] [PubMed] [CrossRef]
44. Stirling, E. R., Terabe, M., Wilson, A. S., Kooshki, M., Yamaleyeva, L. M. et al. (2022). Targeting the CD47/Thrombospondin-1 signaling axis regulates immune cell bioenergetics in the tumor microenvironment to potentiate antitumor immune response. Journal of ImmunoTherapy of Cancer, 10(11), e004712. https://doi.org/10.1136/jitc-2022-004712 [Google Scholar] [PubMed] [CrossRef]
45. Huang, T., Sun, L., Yuan, X., Qiu, H. (2017). Thrombospondin-1 is a multifaceted player in tumor progression. Oncotarget, 8(48), 84546–84558. https://doi.org/10.18632/oncotarget.19165 [Google Scholar] [PubMed] [CrossRef]
46. Petrik, J., Lauks, S., Garlisi, B., Lawler, J. (2023). Thrombospondins in the tumor microenvironment. Seminars in Cell & Developmental Biology. https://doi.org/10.1016/j.semcdb.2023.05.010 [Google Scholar] [PubMed] [CrossRef]
47. Rohrs, J. A., Sulistio, C. D., Finley, S. D. (2016). Predictive model of thrombospondin-1 and vascular endothelial growth factor in breast tumor tissue. NPJ Systems Biology and Applications, 2, 16030. https://doi.org/10.1038/npjsba.2016.30 [Google Scholar] [PubMed] [CrossRef]
48. Kale, A., Rogers, N. M., Ghimire, K. (2021). Thrombospondin-1 CD47 signalling: From mechanisms to medicine. International Journal of Molecular Sciences, 22(8), 4062. https://doi.org/10.3390/ijms22084062 [Google Scholar] [PubMed] [CrossRef]
49. Huang, J., Liu, F., Li, C., Liang, X., Li, C. et al. (2022). Role of CD47 in tumor immunity: A potential target for combination therapy. Scientific Reports, 12(1), 9803. https://doi.org/10.1038/s41598-022-13764-3 [Google Scholar] [PubMed] [CrossRef]
50. Zhang, W., Huang, Q., Xiao, W., Zhao, Y., Pi, J. et al. (2020). Advances in anti-tumor treatments targeting the CD47/SIRPα axis. Frontiers in Immunology, 11, 18. https://doi.org/10.3389/fimmu.2020.00018 [Google Scholar] [PubMed] [CrossRef]
51. Kuo, T. C., Chen, A., Harrabi, O., Sockolosky, J. T., Zhang, A. et al. (2020). Targeting the myeloid checkpoint receptor SIRPα potentiates innate and adaptive immune responses to promote anti-tumor activity. Journal of Hematology & Oncology, 13(1), 160. https://doi.org/10.1186/s13045-020-00989-w [Google Scholar] [PubMed] [CrossRef]
52. Yang, H., Xun, Y., You, H. (2023). The landscape overview of CD47-based immunotherapy for hematological malignancies. Biomarker Research, 11(1), 15. https://doi.org/10.1186/s40364-023-00456-x [Google Scholar] [PubMed] [CrossRef]
53. Mantovani, A., Allavena, P., Marchesi, F., Garlanda, C. (2022). Macrophages as tools and targets in cancer therapy. Nature Review Drug Discovery, 21(11), 799–820. https://doi.org/10.1038/s41573-022-00520-5 [Google Scholar] [PubMed] [CrossRef]
54. de Vries, H. E., Hendriks, J. J., Honing, H., de Lavalette, C. R., van der Pol, S. M. et al. (2002). Signal-regulatory protein alpha-CD47 interactions are required for the transmigration of monocytes across cerebral endothelium. Journal of Immunology, 168(11), 5832–5839. https://doi.org/10.4049/jimmunol.168.11.5832 [Google Scholar] [PubMed] [CrossRef]
55. Dou, M., Chen, Y., Hu, J., Ma, D., Xing, Y. (2020). Recent advancements in CD47 signal transduction pathways involved in vascular diseases. Biomed Research Internaitonal, 2020, 4749135. https://doi.org/10.1155/2020/4749135 [Google Scholar] [PubMed] [CrossRef]
56. Soriano-Romani, L., Mir, F. A., Singh, N., Chin, I., Hafezi-Moghadam, A. et al. (2022). CD47 binding on vascular endothelial cells inhibits IL-17-mediated leukocyte adhesion. International Journal of Molecular Sciences, 23(10), 5705. https://doi.org/10.3390/ijms23105705 [Google Scholar] [PubMed] [CrossRef]
57. Pengam, S., Durand, J., Usal, C., Gauttier, V., Dilek, N. et al. (2019). SIRPα/CD47 axis controls the maintenance of transplant tolerance sustained by myeloid-derived suppressor cells. American Journal of Transplantation, 19(12), 3263–3275. https://doi.org/10.1111/ajt.15497 [Google Scholar] [PubMed] [CrossRef]
58. Wu, L., Yu, G. T., Deng, W. W., Mao, L., Yang, L. L. et al. (2018). Anti-CD47 treatment enhances anti-tumor T-cell immunity and improves immunosuppressive environment in head and neck squamous cell carcinoma. Oncoimmunology, 7(4), e1397248. https://doi.org/10.1080/2162402X.2017.1397248 [Google Scholar] [PubMed] [CrossRef]
59. Kaur, S., Bronson, S. M., Pal-Nath, D., Miller, T. W., Soto-Pantoja, D. R. et al. (2021). Functions of thrombospondin-1 in the tumor microenvironment. International Journal of Molecular Sciences, 22(9), 4570. https://doi.org/10.3390/ijms22094570 [Google Scholar] [PubMed] [CrossRef]
60. Zhao, H., Song, S., Ma, J., Yan, Z., Xie, H. et al. (2022). CD47 as a promising therapeutic target in oncology. Frontiers in Immunolology, 13, 757480. https://doi.org/10.3389/fimmu.2022.757480 [Google Scholar] [PubMed] [CrossRef]
61. Liu, Y., Chang, Y., He, X., Cai, Y., Jiang, H. et al. (2020). CD47 enhances cell viability and migration ability but inhibits apoptosis in endometrial carcinoma cells via the Pi3k/Akt/Mtor signaling pathway. Frontiers in Oncology, 10, 1525. https://doi.org/10.3389/fonc.2020.01525 [Google Scholar] [PubMed] [CrossRef]
62. Jiang, N., Xie, B., Xiao, W., Fan, M., Xu, S. et al. (2022). Fatty acid oxidation fuels glioblastoma radioresistance with CD47-mediated immune evasion. Nature Communications, 13(1), 1511. https://doi.org/10.1038/s41467-022-29137-3 [Google Scholar] [PubMed] [CrossRef]
63. Tanase, C., Enciu, A. M., Codrici, E., Popescu, I. D., Dudau, M. et al. (2022). Fatty Acids, CD36, thrombospondin-1, and CD47 in glioblastoma: Together and/or separately? International Journal of Molecular Sciences, 23(2), 604. https://doi.org/10.3390/ijms23020604 [Google Scholar] [PubMed] [CrossRef]
64. Zhao, X. W., van Beek, E. M., Schornagel, K., Van der Maaden, H., Van Houdt, M. et al. (2011). CD47-signal regulatory protein-alpha (SIRPα) interactions form a barrier for antibody-mediated tumor cell destruction. Proceedings National Academy of Sciences, 108(45), 18342–18347. https://doi.org/10.1073/pnas.1106550108 [Google Scholar] [PubMed] [CrossRef]
65. Wei, Z., Liu, J., Xie, H., Wang, B., Wu, J. et al. (2021). Mir-122-5p mitigates inflammation, reactive oxygen species and Sh-Sy5y apoptosis by targeting Cpeb1 after spinal cord injury via the Pi3k/Akt signaling pathway. Neurochemical Research, 46(4), 992–1005. https://doi.org/10.1007/s11064-021-03232-1 [Google Scholar] [PubMed] [CrossRef]
66. Chun, K. S., Kim, D. H., Raut, P. K., Surh, Y. J. (2022). Anticancer natural products targeting immune checkpoint protein network. Seminars in Cancer Biolology, 86, 1008–1032. https://doi.org/10.1016/j.semcancer.2021.11.006 [Google Scholar] [PubMed] [CrossRef]
67. Golubovskaya, V., Berahovich, R., Zhou, H., Xu, S., Harto, H. et al. (2017). CD47-CAR-T cells effectively kill target cancer cells and block pancreatic tumor growth. Cancers, 9(10), 139. https://doi.org/10.3390/cancers9100139 [Google Scholar] [PubMed] [CrossRef]
68. Beckett, A. N., Chockley, P., Pruett-Miller, S. M., Nguyen, P., Vogel, P. et al. (2023). CD47 expression is critical for car T-cell survival in vivo. Journal of ImmunoTherapy of Cancer, 11(3), e005857. https://doi.org/10.1136/jitc-2022-005857 [Google Scholar] [PubMed] [CrossRef]
69. Yang, Z., Peng, Y., Guo, W., Xu, J., Li, L. et al. (2021). PD-L1 and CD47 co-expression predicts survival and enlightens future dual-targeting immunotherapy in non-small cell lung cancer. Thoracic Cancer, 12(11), 1743–1751. https://doi.org/10.1111/1759-7714.13989 [Google Scholar] [PubMed] [CrossRef]
70. Luo, X., Shen, Y., Huang, W., Bao, Y., Mo, J. et al. (2023). Blocking CD47-SIRPα signal axis as promising immunotherapy in ovarian cancer. Cancer Control, 30, 10732748231159706. https://doi.org/10.1177/10732748231159706 [Google Scholar] [PubMed] [CrossRef]
71. Wang, Y., Ni, H., Zhou, S., He, K., Gao, Y. et al. (2021). Tumor-selective blockade of CD47 signaling with a CD47/PD-L1 bispecific antibody for enhanced anti-tumor activity and limited toxicity. Cancer Immunology Immunotherapy, 70(2), 365–376. https://doi.org/10.1007/s00262-020-02679-5 [Google Scholar] [PubMed] [CrossRef]
72. Chen, H., Yang, Y., Deng, Y., Wei, F., Zhao, Q. et al. (2022). Delivery of CD47 blocker SIRPα-Fc by CAR-T cells enhances antitumor efficacy. Journal for ImmunoTherapy of Cancer, 10(2), e003737. https://doi.org/10.1136/jitc-2021-003737 [Google Scholar] [PubMed] [CrossRef]
73. Xu, Y., Li, J., Tong, B., Chen, M., Liu, X. et al. (2020). Positive tumour CD47 expression is an independent prognostic factor for recurrence in resected non-small cell lung cancer. ESMO Open, 5(4), e000823. https://doi.org/10.1136/esmoopen-2020-000823 [Google Scholar] [PubMed] [CrossRef]
74. Wang, D. R., Wu, X. L., Sun, Y. L. (2022). Therapeutic targets and biomarkers of tumor immunotherapy: Response versus non-response. Signal Transduction and Targeted Therapy, 7(1), 331. https://doi.org/10.1038/s41392-022-01136-2 [Google Scholar] [PubMed] [CrossRef]
75. Chen, C., Wang, R., Chen, X., Hou, Y., Jiang, J. (2022). Targeting CD47 as a novel immunotherapy for breast cancer. Frontiers in Oncology, 12, 924740. https://doi.org/10.3389/fonc.2022.924740 [Google Scholar] [PubMed] [CrossRef]
76. Yordanov, A., Shivarov, V., Kostov, S., Ivanova, Y., Dimitrova, P. et al. (2023). Prognostic utility of CD47 in cancer of the uterine cervix and the sensitivity of immunohistochemical scores. Diagnostics, 13(1), 52. https://doi.org/10.3390/diagnostics13010052 [Google Scholar] [PubMed] [CrossRef]
77. Kosaka, A., Ishibashi, K., Nagato, T., Kitamura, H., Fujiwara, Y. et al. (2021). CD47 blockade enhances the efficacy of intratumoral sting-targeting therapy by activating phagocytes. Journal of Experimental Medicine, 218(11), e20200792. https://doi.org/10.1084/jem.20200792 [Google Scholar] [PubMed] [CrossRef]
78. Dutta, S., Ganguly, A., Chatterjee, K., Spada, S., Mukherjee, S. (2023). Targets of immune escape mechanisms in cancer: Basis for development and evolution of cancer immune checkpoint inhibitors. Biology, 12(2), 218. https://doi.org/10.3390/biology12020218 [Google Scholar] [PubMed] [CrossRef]
79. Zhao, H., Wu, L., Yan, G., Chen, Y., Zhou, M. et al. (2021). Inflammation and tumor progression: Signaling pathways and targeted intervention. Signal Transduction and Targeted Therapy, 6(1), 263. https://doi.org/10.1038/s41392-021-00658-5 [Google Scholar] [PubMed] [CrossRef]
80. Huang, Y., Ge, W., Zhou, J., Gao, B., Qian, X. et al. (2021). The role of tumor associated macrophages in hepatocellular carcinoma. Journal of Cancer, 12(5), 1284–1294. https://doi.org/10.7150/jca.51346 [Google Scholar] [PubMed] [CrossRef]
81. Sun, J., Muz, B., Alhallak, K., Markovic, M., Gurley, S. et al. (2020). Targeting CD47 as a novel immunotherapy for multiple myeloma. Cancers, 12(2), 305. https://doi.org/10.3390/cancers12020305 [Google Scholar] [PubMed] [CrossRef]
82. Yang, M., Mahanty, A., Jin, C., Wong, A. N. N., Yoo, J. S. (2022). Label-free metabolic imaging for sensitive and robust monitoring of anti-CD47 immunotherapy response in triple-negative breast cancer. Journal of ImmunoTherapy of Cancer, 10(9), e005199. https://doi.org/10.1136/jitc-2022-005199 [Google Scholar] [PubMed] [CrossRef]
83. Zeidan, A. M., DeAngelo, D. J., Palmer, J., Seet, C. S., Tallman, M. S. et al. (2022). Phase 1 study of anti-CD47 monoclonal antibody Cc-90002 in patients with relapsed/refractory acute myeloid leukemia and high-risk myelodysplastic syndromes. Annals of Hematolology, 101(3), 557–569. https://doi.org/10.1007/s00277-021-04734-2 [Google Scholar] [PubMed] [CrossRef]
84. Pandey, P., Khan, F., Qari, H. A., Upadhyay, T. K., Alkhateeb, A. F. et al. (2022). Revolutionization in cancer therapeutics via targeting major immune checkpoints PD-1, PD-L1 and CTLA-4. Pharmaceuticals, 15(3), 335. https://doi.org/10.3390/ph15030335 [Google Scholar] [PubMed] [CrossRef]
85. Qu, T., Li, B., Wang, Y. (2022). Targeting CD47/SIRPα as a therapeutic strategy, where we are and where we are headed. Biomarker Research, 10(1), 20. https://doi.org/10.1186/s40364-022-00373-5 [Google Scholar] [PubMed] [CrossRef]
86. Maute, R., Xu, J., Weissman, I. L. (2022). CD47-SIRPα-targeted therapeutics: Status and prospects. Immuno-Oncology and Technology, 13, 100070. https://doi.org/10.1016/j.iotech.2022.100070 [Google Scholar] [PubMed] [CrossRef]
87. Chao, M. P., Weissman, I. L., Majeti, R. (2012). The CD47-SIRPα pathway in cancer immune evasion and potential therapeutic implications. Current Opinion in Immunology, 24(2), 225–232. https://doi.org/10.1016/j.coi.2012.01.010 [Google Scholar] [PubMed] [CrossRef]
88. Advani, R., Flinn, I., Popplewell, L., Forero, A., Bartlett, N. L. et al. (2018). CD47 blockade by Hu5f9-G4 and rituximab in non-hodgkin’s lymphoma. New England Journal of Medicine, 379(18), 1711–1721. https://doi.org/10.1056/NEJMoa1807315 [Google Scholar] [PubMed] [CrossRef]
89. Ansell, S. M., Maris, M. B., Lesokhin, A. M., Chen, R. W., Flinn, I. W. et al. (2021). Phase I study of the CD47 blocker TTI-621 in patients with relapsed or refractory hematologic malignancies. Clinical Cancer Research, 27(8), 2190–2199. https://doi.org/10.1158/1078-0432.CCR-20-3706 [Google Scholar] [PubMed] [CrossRef]
90. Tao, H., Qian, P., Wang, F., Yu, H., Guo, Y. (2017). Targeting CD47 enhances the efficacy of anti-PD-1 and CTLA-4 in an esophageal squamous cell cancer preclinical model. Oncology Research, 25(9), 1579–1587. https://doi.org/10.3727/096504017X14900505020895 [Google Scholar] [PubMed] [CrossRef]
91. Chen, S. H., Dominik, P. K., Stanfield, J., Ding, S., Yang, W. et al. (2021). Dual checkpoint blockade of CD47 and PD-L1 using an affinity-tuned bispecific antibody maximizes antitumor immunity. Journal for ImmunoTherapy of Cancer, 9(10), e003464. https://doi.org/10.1136/jitc-2021-003464 [Google Scholar] [PubMed] [CrossRef]
92. Kanemura, H., Hayashi, H., Tomida, S., Tanizaki, J., Suzuki, S. et al. (2022). The tumor immune microenvironment and frameshift neoantigen load determine response to PD-L1 blockade in extensive-stage SCLC. JTO Clinical and Research Reports, 3(8), 100373. https://doi.org/10.1016/j.jtocrr.2022.100373 [Google Scholar] [PubMed] [CrossRef]
93. Chen, Y. C., Shi, W., Shi, J. J., Lu, J. J. (2022). Progress of CD47 immune checkpoint blockade agents in anticancer therapy: A hematotoxic perspective. Journal of Cancer Research and Clinical Oncology, 148(1), 1–14. https://doi.org/10.1007/s00432-021-03815-z [Google Scholar] [PubMed] [CrossRef]
94. Liu, Y., Wang, Y., Yang, Y., Weng, L., Wu, Q. et al. (2023). Emerging phagocytosis checkpoints in cancer immunotherapy. Signal Transducttion and Targeted Therapy, 8(1), 104. https://doi.org/10.1038/s41392-023-01365-z [Google Scholar] [PubMed] [CrossRef]
95. Oldenborg, P. A., Zheleznyak, A., Fang, Y. F., Lagenaur, C. F., Gresham, H. D. et al. (2000). Role of CD47 as a marker of self on red blood cells. Science, 288(5473), 2051–2054. https://doi.org/10.1126/science.288.5473.2051 [Google Scholar] [PubMed] [CrossRef]
96. Mestrallet, G., Sone, K., Bhardwaj, N. (2022). Strategies to overcome Dc dysregulation in the tumor microenvironment. Frontiers in Immunology, 13, 980709. https://doi.org/10.3389/fimmu.2022.980709 [Google Scholar] [PubMed] [CrossRef]
97. Anderson, K. L., Snyder, K. M., Ito, D., Lins, D. C., Mills, L. J. et al. (2020). Evolutionarily conserved resistance to phagocytosis observed in melanoma cells is insensitive to upregulation of pro-phagocytic signals and to CD47 blockade. Melanoma Research, 30(2), 147–158. https://doi.org/10.1097/CMR.0000000000000629 [Google Scholar] [PubMed] [CrossRef]
98. Yuan, J., Shi, X., Chen, C., He, H., Liu, L. et al. (2019). High expression of CD47 in triple negative breast cancer is associated with epithelial-mesenchymal transition and poor prognosis. Oncology Letters, 18(3), 3249–3255. https://doi.org/10.3892/ol.2019.10618 [Google Scholar] [PubMed] [CrossRef]
99. Li, K., Shi, H., Zhang, B., Ou, X., Ma, Q. et al. (2021). Myeloid-derived suppressor cells as immunosuppressive regulators and therapeutic targets in cancer. Signal Transduction and Targeted Therapy, 6(1), 362. https://doi.org/10.1038/s41392-021-00670-9 [Google Scholar] [PubMed] [CrossRef]
100. Xu, S., Wang, C., Yang, L., Wu, J., Li, M. et al. (2023). Targeting immune checkpoints on tumor-associated macrophages in tumor immunotherapy. Frontiers in Immunology, 14, 1199631. https://doi.org/10.3389/fimmu.2023.1199631 [Google Scholar] [PubMed] [CrossRef]
101. Zhang, H., Liu, L., Liu, J., Dang, P., Hu, S. et al. (2023). Roles of tumor-associated macrophages in anti-Pd-1/Pd-L1 immunotherapy for solid cancers. Molecular Cancer, 22(1), 58. https://doi.org/10.1186/s12943-023-01725-x [Google Scholar] [PubMed] [CrossRef]
102. Guo, Z., Zhang, R., Yang, A. G., Zheng, G. (2023). Diversity of immune checkpoints in cancer immunotherapy. Frontiers in Immunology, 14, 1121285. https://doi.org/10.3389/fimmu.2023.1121285 [Google Scholar] [PubMed] [CrossRef]
103. Gibellini, L., Borella, R., Santacroce, E., Serattini, E., Boraldi, F. et al. (2023). Circulating and tumor-associated neutrophils in the Era of immune checkpoint inhibitors: Dynamics, phenotypes, metabolism, and functions. Cancers, 15(13), 3327. https://doi.org/10.3390/cancers15133327 [Google Scholar] [PubMed] [CrossRef]
104. Votava, M., Bartolini, R., Capkova, L., Smetanova, J., Jiri, V. et al. (2022). The expression profiles of CD47 in the tumor microenvironment of salivary gland cancers: A next step in histology-driven immunotherapy. BMC Cancer, 22(1), 1021. https://doi.org/10.1186/s12885-022-10114-4 [Google Scholar] [PubMed] [CrossRef]
105. Bian, Y., Lin, T., Jakos, T., Xiao, X., Zhu, J. (2022). The generation of dual-targeting fusion protein Pd-L1/CD47 for the inhibition of triple-negative breast cancer. Biomedicines, 10(8), 1843. https://doi.org/10.3390/biomedicines10081843 [Google Scholar] [PubMed] [CrossRef]
106. Du, K., Li, Y., Liu, J., Chen, W., Wei, Z. et al. (2021). A bispecific antibody targeting Gpc3 and CD47 induced enhanced antitumor efficacy against dual antigen-expressing HCC. Molecular Therapy, 29(4), 1572–1584. https://doi.org/10.1016/j.ymthe.2021.01.006 [Google Scholar] [PubMed] [CrossRef]
107. Qiu, Q., Lin, Y., Ma, Y., Li, X., Liang, J. et al. (2020). Exploring the emerging role of the gut microbiota and tumor microenvironment in cancer immunotherapy. Frontiers in Immunology, 11, 612202. https://doi.org/10.3389/fimmu.2020.612202 [Google Scholar] [PubMed] [CrossRef]
108. Cao, A., Yi, J., Tang, X., Szeto, C. W., Wu, R. et al. (2022). CD47-blocking antibody Zl-1201 promotes tumor-associated macrophage phagocytic activity and enhances the efficacy of the therapeutic antibodies and chemotherapy. Cancer Research Communications, 2(11), 1404–1417. https://doi.org/10.1158/2767-9764.CRC-22-0266 [Google Scholar] [PubMed] [CrossRef]
109. Zhang, B., Li, W., Fan, D., Tian, W., Zhou, J. et al. (2022). Advances in the study of CD47-based bispecific antibody in cancer immunotherapy. Immunology, 167(1), 15–27. https://doi.org/10.1111/imm.13498 [Google Scholar] [PubMed] [CrossRef]
110. Ciotti, G., Marconi, G., Sperotto, A., Giannini, M. B., Gottardi, M. et al. (2023). Biological therapy in elderly patients with acute myeloid leukemia. Expert Opinion on Biological Therapy, 23(2), 175–194. https://doi.org/10.1080/14712598.2023.2174015 [Google Scholar] [PubMed] [CrossRef]
111. DiNardo, K. W., LeBlanc, T. W., Chen, H. (2023). Novel agents and regimens in acute myeloid leukemia: Latest updates from 2022 ASH annual meeting. Journal of Hematology & Oncology, 16(1), 17. https://doi.org/10.1186/s13045-023-01411-x [Google Scholar] [PubMed] [CrossRef]
112. Bazinet, A., Bravo, G. M. (2022). New approaches to myelodysplastic syndrome treatment. Current Treatment Options in Oncology, 23(5), 668–687. https://doi.org/10.1007/s11864-022-00965-1 [Google Scholar] [PubMed] [CrossRef]
113. Madanat, Y. F., Xie, Z., Zeidan, A. M. (2023). Advances in myelodysplastic syndromes: Promising novel agents and combination strategies. Expert Review of Hematology, 16(1), 51–63. https://doi.org/10.1080/17474086.2023.2166923 [Google Scholar] [PubMed] [CrossRef]
114. Narla, R. K., Modi, H., Bauer, D., Abbasian, M., Leisten, J. et al. (2022). Modulation of CD47-SIRPα innate immune checkpoint axis with Fc-function detuned anti-CD47 therapeutic antibody. Cancer Immunology Immunotherapy, 71(2), 473–489. https://doi.org/10.1007/s00262-021-03010-6 [Google Scholar] [PubMed] [CrossRef]
115. Armengol, M., Santos, J. C., Fernandez-Serrano, M., Profitos-Peleja, N., Ribeiro, M. L. et al. (2021). Immune-checkpoint inhibitors in B-cell lymphoma. Cancers, 13(2), 214. https://doi.org/10.3390/cancers13020214 [Google Scholar] [PubMed] [CrossRef]
116. Llombart, V., Mansour, M. R. (2022). Therapeutic targeting of “Undruggable” MYC. eBioMedicine, 75(1), 103756. https://doi.org/10.1016/j.ebiom.2021.103756 [Google Scholar] [PubMed] [CrossRef]
Cite This Article
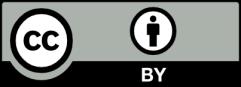