Open Access
REVIEW
Carbon Monoxide Modulates Auxin Transport and Nitric Oxide Signaling in Plants under Iron Deficiency Stress
1 Jiangsu Key Laboratory for Eco-Agricultural Biotechnology around Hongze Lake, Jiangsu Collaborative Innovation Center of Regional Modern Agriculture and Environmental Protection, Huaiyin Normal University, Huaian, 223300, China
2 School of Life Sciences, Nanjing Forestry University, Nanjing, 210037, China
3 School of Life Sciences, Shanghai University, Shanghai, 200444, China
* Corresponding Author: Yuming Luo. Email:
Phyton-International Journal of Experimental Botany 2024, 93(1), 45-61. https://doi.org/10.32604/phyton.2023.046389
Received 28 September 2023; Accepted 20 November 2023; Issue published 26 January 2024
Abstract
Carbon monoxide (CO) and nitric oxide (NO) are signal molecules that enhance plant adaptation to environmental stimuli. Auxin is an essential phytohormone for plant growth and development. CO and NO play crucial roles in modulating the plant’s response to iron deficiency. Iron deficiency leads to an increase in the activity of heme oxygenase (HO) and the subsequent generation of CO. Additionally, it alters the polar subcellular distribution of Pin-Formed 1 (PIN1) proteins, resulting in enhanced auxin transport. This alteration, in turn, leads to an increase in NO accumulation. Furthermore, iron deficiency enhances the activity of ferric chelate reductase (FCR), as well as the expression of the Fer-like iron deficiency-induced transcription factor 1 (FIT) and the ferric reduction oxidase 2 (FRO2) genes in plant roots. Overexpression of the long hypocotyl 1 (HY1) gene, which encodes heme oxygenase, or the CO donor treatment resulted in enhanced basipetal auxin transport, higher FCR activity, and the expression of FIT and FRO2 genes under Fe deficiency. Here, a potential mechanism is proposed: CO and NO interact with auxin to address iron deficiency stress. CO alters auxin transport, enhancing its accumulation in roots and up-regulating key iron-related genes like FRO2 and IRT1. Elevated auxin levels affect NO signaling, leading to greater sensitivity in root development. This interplay promotes FCR activity, which is crucial for iron absorption. Together, these molecules enhance iron uptake and root growth, revealing a novel aspect of plant physiology in adapting to environmental stress.Keywords
Abbreviations
ABA | Abscisic acid |
bHLH | Basic helix-loop-helix |
CO | Carbon monoxide |
FCR | Ferric chelate reductase |
FIT1 | Fer-like iron deficiency-induced transcription factor 1 |
FRO2 | Ferric reduction oxidase 2 |
GSH | Glutathione |
IRT | Iron-regulated transporter gene |
HO | Heme oxygenase |
hy1 | Long hypocotyl 1 |
NADH | Nicotinamide adenine dinucleotide |
NO | Nitric oxide |
NR | Nitrate reductase |
PIN1 | Pin formed 1 |
PIN2 | Pin formed 2 |
RNS | Reactive nitrogen species |
XOR | Xanthinine oxidoreductase |
Iron is a micronutrient indispensable for the growth and development of plants. It plays a pivotal role within plant organisms [1], encompassing crucial functions such as chlorophyll synthesis [2], DNA replication [3], nitrogen metabolism [4], and antioxidant defense [5]. Insufficient iron levels can detrimentally impact photosynthesis, resulting in diminished growth and reduced yields [6]. Furthermore, iron deficiency impedes fundamental biological processes, including cell division and the development of new tissues [7]. Iron deficiency also exerts adverse effects on protein synthesis in plants [8]. Iron plays a pivotal role in enabling plants to mitigate oxidative damage [9] and is essential for root development and nitrogen fixation—processes intimately linked to the growth and viability of plants [10]. Consequently, the investigation of how plants adapt to iron deficiency stands as a matter of paramount significance in the realm of academic research.
CO and NO hold substantial significance in the physiological reactions of plants to iron deficiency (ID). Recent research has elucidated the mechanisms by which CO and NO contribute to plants’ responses to ID. Under conditions of ID, an increase in heme oxygenase (HO) activity, responsible for CO generation, has been observed [11]. The increased CO production subsequently affects auxin transport by altering the polar subcellular distribution of PIN1 proteins. Additionally, NO has a discernible impact on auxin signaling. This phenomenon was observed in Arabidopsis plants subjected to a 12-h period of cadmium-induced stress, wherein a reduction in the accumulation of PIN1 protein and the stability of AXR3/IAA17 protein (which acts as an inhibitor of IAA signaling) were observed [12]. The occurrence of ID has been associated with an elevation in nitric oxide (NO) levels and an upregulation of FCR activity. FCR is a key enzyme involved in iron absorption, as substantiated by experiments conducted on pear trees [13]. Furthermore, the expression of critical genes associated with iron homeostasis, including Fer-like ID-induced transcription factor 1 (FIT), has also been upregulated [14]. Recent research has underscored that the absence of FIT function leads to a reduced capacity of plants to efficiently uptake iron, ultimately culminating in their mortality [15,16].
In recent times, there has been an increasing fascination among researchers regarding the exploration of signal molecules and their pivotal functions in enabling plants to adapt to a multitude of environmental stressors. Within this realm of signal molecules, carbon monoxide (CO) and nitric oxide (NO) have emerged as indispensable regulators, orchestrating the plant’s responses to a wide spectrum of environmental stimuli [17]. Moreover, auxin, a widely recognized phytohormone, plays a pivotal role in regulating the growth and development of plants. There has been a lot of interest in learning how CO, NO, and auxin interact to control how plants react to environmental stress, especially iron deficiency (ID) [18].
For many decades, CO has been predominantly recognized as a toxic gas, presenting significant harm to both humans and animals when present in elevated concentrations. Likewise, NO has primarily been the subject of research within the realm of mammalian physiology and pathology. Elevated levels of CO have been found to significantly hinder cellular activity and increase the responsiveness of cancer cells to chemotherapy [19]. In mammals, NO functions as a widely distributed signaling molecule, that assumes multifaceted roles encompassing vasodilation, platelet function, neurotransmission, immunity, and metabolism [20]. On the contrary, in the field of botany, there has been a scarcity of research on the functions of CO and NO, leading to an incomplete comprehension of their roles. Nonetheless, recent studies have unveiled the intricate and multifarious roles of CO and NO in plant biology. In plants, CO and NO primarily regulate plant iron homeostasis through their ability to induce the expression of ferritin transcription factors [21]. These molecules have shown their importance in orchestrating different facets of plant biology, including the regulation of plant growth, development, and responses to an array of environmental stressors such as drought, salinity, high temperature, cold, nutrient deficiency, and exposure to heavy metals.
The purpose of this review is to delve into the interactions among NO, CO, and Auxin, aiming to unveil novel mechanisms of their involvement in plant physiological regulation. While past research has elucidated the significance of these signaling molecules in plant physiological processes, further investigations are required to understand how they influence each other and collaborate at different growth stages. The objective of this paper is to consolidate existing literature, emphasizing the pivotal roles of NO, CO, and Auxin throughout the entire course of plant growth and development. Additionally, we aim to introduce new insights into how they collectively govern plant adaptability and growth performance. Through this review, our intention is to inspire future research and lay the foundation for further exploration and innovation in the field of plant biology.
2 The Roles of Carbon Monoxide, Nitric Oxide, and Auxin in Plant Physiology
Within the field of plant physiology, there has been a significant surge in attention towards small-molecule compounds like CO, NO, and auxin in recent times [22]. As signaling molecules, they play pivotal roles in plant growth and development and how plants respond to environmental stresses, nutrient uptake, and root development [23]. Iron, a crucial element for plants, is central to processes such as chlorophyll synthesis and cellular respiration [24]. CO finely tunes plant iron metabolism, ensuring an adequate supply of this essential element and contributing to plant health [25].
NO, acting as a signaling molecule, plays a pivotal role in regulating plant growth and development [26]. It regulates cell growth, differentiation, and organ formation, enabling plants to adapt to environmental stressors such as drought, diseases, and salt stress [27]. This regulatory mechanism equips plants to cope with various challenges, safeguarding their survival and growth [24].
Additionally, the plant hormone auxin influences root development [28], organ formation [29], and overall growth [30]. Small molecules like CO and NO fine-tune auxin transport and distribution, enhancing plant adaptability and survival [31].
In summary, CO and NO’s interplay is central to coordinating and regulating critical physiological processes in plants, ensuring their ability to thrive and adapt to diverse conditions while managing stressors, ultimately contributing to plant health and adaptability [24,25].
In plants, CO can trigger a series of physiological responses and has thus become an important signaling molecule. It has a positive impact on seed germination, root development, and stomatal closure. Furthermore, CO typically enhances the plant’s resistance to abiotic stress by strengthening the antioxidant defense system [32]. In plants, endogenous CO is generated through HO converting heme into biliverdin IXa (BV), CO, and free iron (Fe2+) [33]. This conversion occurs through a series of reduction and oxygenation reactions, which take place in the presence of molecular oxygen, with electrons supplied by NADPH [34] (Fig. 1). The involvement of HO in regulating iron homeostasis may be considered a pivotal factor in the modulation of cell death [22]. In Arabidopsis, four HO genes have been characterized and studied, including HY1, HO2, HO3, and HO4. However, it has been determined that only the enzyme encoded by the HY1 gene is responsible for CO production [35].
Figure 1: Pathways of CO generation in plants
NO is a gaseous signaling molecule that functions as a molecular messenger in both animal and plant cells. In eukaryotes, it is typically produced endogenously, whereas in higher plants, its production is mediated by a number of redox pathways [36]. NO has a crucial role in regulating several growth and developmental processes in plants, such as seed germination and flowering. Additionally, it is involved in the plant’s response to environmental stresses such as drought, high temperature, and salinity [37]. It is necessary for the symbiotic relationship between legumes and nitrogen-fixing bacteria [38]. According to recent studies, NO and ethylene signals enhance salt-alkali resistance in tomatoes, with NO acting as an upstream regulatory factor within the ethylene signaling pathway [39]. In Arabidopsis, the shoot apical meristem serves as a crucial organ for sensing high temperatures, as it exhibits a rapid release of NO in response to heat stress. Following that, the S-nitrosoglutathione (GSNO) molecule, which is a relatively stable active molecule of NO, functions as a mobile signaling molecule [40]. NO is also transported from the aboveground part of the plant to the roots through the vascular bundle [41]. This transport process subsequently initiates the response to high temperatures and establishes heat tolerance over the entire plant [42]. Furthermore, researchers have explored the impact of melatonin (MT) on pepper plants’ responses to salt stress (SS) and ID, revealing potential signal crosstalk between NO and H2S in MT-induced tolerance [43].
Auxin is an endogenous hormone characterized by the presence of an unsaturated aromatic ring and an acetic acid side chain. It is a small molecule with a low molecular weight widely present in plants, playing key roles in several physiological processes, including growth stimulation, the regulation of plant organ formation, stem elongation, lateral root generation [44], fruit development [45], and response to environmental stimuli. Even at low concentrations, auxin can regulate gene expression through the activation of specific transcription factors and proteins [46]. Recent research has revealed the role of iron in regulating root hair growth under phosphorus deficiency conditions; iron may influence auxin signal transduction by modulating vacuolar transport [47]. Auxin can be synthesized via two distinct pathways: tryptophan (Trp)-dependent and non-Trp-dependent pathways [48,49]. The Trp-dependent auxin synthesis pathways encompass a range of intermediates that give rise to pathways such as the indole-3-pyruvic acid (IPA) pathway, tryptamine (TAM) pathway, indole-3-acetonitrile (IAOx) pathway, and indole-3-acetamide (IAM) pathway [48,50]. In the IPA pathway, the conversion of Trp into indole acetic acid (IAA) occurs through a two-step process. Initially, Trp forms IPA, and then, under the action of a specific dehydrogenase, it oxidizes into indoleacetic acid. In the TAM pathway, Trp is first transformed into TAM by tryptophan decarboxylase [51]. Then, through a series of enzymatic reactions, TAM is transformed into indole-3-acetaldehyde (IAAld), which is ultimately oxidized into IAA by a specific dehydrogenase. In the indole-3-acetaldehyde oxime (IAOx) pathway, Trp is initially transformed into IAOx [52], IAOx is further converted into indole-3-acetonitrile (IAN), and finally, IAN is transformed into IAA by nitrilase [53]. In the IAM pathway, Trp is first converted into IAM and subsequently into IAA [48,54]. Although non-Trp-dependent auxin synthesis pathways have been confirmed in maize and Arabidopsis yet, their specific mechanisms remain unclear. This auxin synthesis pathway plays a crucial role in the different developmental stages of various species [50].
As a signaling molecule, auxin’s signal transduction pathway is relatively simple. Most auxins form a receptor-enzyme complex by binding to their respective receptors [55], which leads to the direct hydrolysis of specific transcription inhibitors, thereby activating downstream auxin response genes [56]. Additionally, it has been suggested that there may be a potential interaction among CO, NO, and auxin signaling in plants under ID conditions [57]. NO negatively regulates auxin signal transduction by inhibiting the degradation of the IAA17 protein [58]. Increasing evidence indicates that NO and NO-mediated S-nitrosylation play a vital role in auxin signal transduction. These components are involved in various aspects of auxin-induced cellular processes, such as the cell division cycle, the formation of primary roots, lateral root development, adventitious root formation, and root hair development [59].
3 Nutrient Deficiency in Plants
Plants require a diverse array of nutrients to facilitate their growth and ensure their survival. These nutrients can be categorized into two main groups: non-mineral and mineral [60]. Hydrogen, carbon, and oxygen are classified as non-mineral, whereas nitrogen, phosphorus, potassium, calcium, magnesium, sulfur, iron, boron, copper, chloride, manganese, molybdenum, and zinc minerals are obtained from the soil through the roots [61]. Plant development can be limited in natural soils due to the scarcity of certain mineral nutrients. The ability of plants to perceive alterations in nutrient levels is a phenomenon that remains inadequately elucidated at the molecular level. Recent studies show the importance of NO and CO in nutrient absorption by plants [62].
During plant growth, auxin, through ARF, regulates nutrient absorption and distribution in plants, leading to the formation of distribution centers where auxin concentration is high [50,63]. A study found that IAA regulates iron chelate reductase and iron transport genes, impacting iron uptake by cucumber roots [64]. This suggests IAA is involved in the regulation of root responses to iron stress [65]. In mild nitrogen deficiency, Arabidopsis thaliana produces local auxin to boost lateral root growth, thus enhancing plants’ nutrient absorption [66]. Phosphorus deficiency in plants results in primary root growth stagnation and increased auxin content in lateral root formation [67]. In A. thaliana, OsAUX1 regulates auxin transport from the root tip to the differentiation zone [68]. In rice, OsPIN1b responds to phosphorus deficiency by regulating the activity of the root tip meristem, and participating in the elongation of rice SR (seminal root) [69,70].
Iron (Fe) plays a crucial role in plant growth, particularly in chlorophyll synthesis. However, the solubility of crystalline Fe minerals in soil is typically low [71]. The primary reason for iron deficiency in plants is the insolubility of iron (III) oxides in the soil [72]. The main factors that alter the solubility of iron in the soil include redox processes and the presence of chelators [73]. Plants adopt two distinct strategies for iron acquisition (Fig. 2): Enhancing the reduction of ferric iron in the roots to promote absorption in non-graminaceous plants such as Arabidopsis, and employing plant-specific iron transporters in graminaceous plants like barley [74]. The strategy I adopted by dicotyledonous plants to acquire iron from the external medium is based not only on the reduction of Fe3+ but also involves the acidification of the external medium and the secretion of several compounds, including organic acids and phenols. In most non-graminaceous plants, key transcription factors such as FER in tomato and its homolog FIT in Arabidopsis are responsible for the activation of FCR. This process induces soil acidification around roots, hence facilitating the transport of iron by the iron-regulated transporter gene (IRT) into the roots. Recently, a novel iron transporter, ZmIRT2, was discovered in maize, providing additional support to the previously reported research findings [75]. Remarkably, despite possessing distinct iron absorption systems, both Arabidopsis and rice employ a dual-molecular module (FIT-bHLH Ib) to initiate the iron uptake process under ID conditions [76]. Researchers have elucidated that bHLH transcription factors play a pivotal role in enabling plant cells to establish a photoprotective mechanism [77]. This mechanism, in turn, induces alterations in chloroplast morphology, thereby mitigating the generation of deleterious reactive oxygen species (ROS) while concurrently facilitating the restoration of photosystem II functionality [78]. These research findings unveil a compelling convergence: under iron stress, both of these plant species harness analogous molecular modules to govern iron absorption. This strategic response enhances their resilience to environmental stressors by modulating chloroplast status.
Figure 2: Strategies for Iron acquisition and adaptation in plants
3.2 The Roles of Carbon Monoxide and Nitric Oxide in Plant Response to Iron Deficiency
Numerous research state that NO is essential for plants to sense ID and controls the uptake of Fe. ID causes NO to build up, and exogenous NO applied to tomato roots lacking in iron boosts the expression of iron-uptake-related genes. These genes include the divalent cation transporter IRT1, the transcription factor FER, and the iron chelate reductase FRO1 [79]. Previous research has indicated that elevating NO levels in rice promotes the development of lateral roots and increases HO activity [80], hence increasing the plant’s resistance to ID [57]. Cucumber adventitious roots require the formation of CO, which is produced when NO stimulates the action of the HO enzyme [81].
Both NO and CO have been identified as crucial regulators of plant cell metabolism, participating in various stages of plant development, including maturation, seed dormancy and germination, hypocotyl elongation, and root development [82,83]. Research has indicated that ID elevates endogenous CO levels in Arabidopsis [84]. In Populus, the expression of PtIRT1 significantly increases during iron deficiency [85]. The same is true in Arachis hypgaea, with a significant increase in the expression of AhIRT1 during iron deficiency [86]. Additionally, exogenous CO application to iron-deficient Arabidopsis has been found to mitigate leaf chlorosis and increase chlorophyll accumulation, iron levels, and NO accumulation in the root tips.
Both NO and CO are required for FIT expression and FRO activity in plants under ID [87]. NO is reported to regulate target protein stability during the hormone response. NO can also stabilize the FIT protein in Arabidopsis [88]. However, it is unknown whether CO directly controls FIT and its associated protein degradation during ID or whether it requires interaction with the NO signal. In regulating environmental stress, over-accumulation of NO leads to the generation of reactive nitrogen species (RNS) damage, initiating the modification of proteins through S-nitrosylation, which plays a role in plant sensing abscisic acid (ABA) [89,90]. It is reported that CO can reduce RNS damage in recalcitrant seed germination [91]. Further work is required to determine whether the FIT protein undergoes post-translational modification by NO-mediated S-nitrosylation or if CO can affect this alteration. These studies indicate that the interaction between CO, NO, and their crosstalk may play important roles in improving plant tolerance to ID (Fig. 3).
Figure 3: Interplay of NO and CO in enhancing plant tolerance to iron deficiency
When the exogenous concentration of CO is 8 mM in an Fe-deficient medium, it has been observed that there is an increase in the accumulation of iron in C. mydomonas reinhardtii, which subsequently prevents the yellowing induced by ID and promotes the accumulation of chlorophyll. Additionally, the expression levels of FOX1, FTR1, and iron redox protein were up-regulated under these conditions. This upregulation further enhances the accumulation of NO in the plant. However, it should be noted that the presence of the NO scavenger may inhibit the effects of NO accumulation [92]. Cyg11, a Guanylate Cyclase in C. reinhardtii, has been suggested as a potential CO sensor and is believed to be involved in the CO-dependent recovery pathway that is activated under iron-deficient growth conditions [93]. AtHO1’s catabolism of iron homeostasis is linked with the production of CO. The CO generated by AtHO1 catalysis may promote the transport of iron in iron-containing components and increase its bioavailability to tissues that require iron [94]. Additionally, the application of an exogenous concentration of 50 μM CO can promote chlorophyll accumulation and greening in A. thaliana [95]. Similar observations were also found in corn [96], indicating that CO plays a certain role in maintaining Fe homeostasis in plants.
3.3 Role of Auxin during Iron Deficiency
Previous studies have indicated that the plant hormone auxin stimulates FCR activity in response to ID. Exogenous introduction of auxin can increase FCR activity as well as FIT and FRO2 expression [87]. Such effects could be impaired by auxin transport inhibitor NPA treatment or in the presence of auxin transport mutant, such as aux1-7. In contrast to wild-type plants, the Arabidopsis yuc1 mutant, which exhibits elevated auxin levels, showed higher FCR activity under ID conditions [57]. This suggests that the transportation of auxin from the shoot to the root is necessary for FCR activity and iron uptake during ID [97]. Sucrose can increase root-ward auxin transport, and the sucrose accumulation induced by Fe deficiency acts along with auxin in transmitting ID signals in Arabidopsis [98]. Scholars recent investigations have shown that Fe stimulates the elongation of lateral roots through the upregulation of the auxin influx carrier AUX1 expression, increasing the auxin content at the tips of lateral roots, and serving as a prerequisite for lateral root elongation [99]. According to a recent study, it has been found that Fe may regulate vacuolar transport, hence affecting auxin’s signal transduction [47]. Guo et al. have studied the role of PbrSAUR72 in regulating the response of pear trees to ID and found the modulation of auxin signal transduction promoted iron uptake and reduced oxidative stress in those species [100].
In summary, both NO and CO act as signaling molecules to enhance FCR activity and facilitate Fe uptake during ID in plants. Auxin transmission from the shoot to the root triggers the biosynthesis of NO, which further upregulates FCR activity to enhance Fe uptake. During this process, ID also induces HY1 activity, which is responsible for CO biosynthesis [22,101]. It is possible that CO promotes basipetal polar auxin transport into the root tip by altering the asymmetric accumulation of PIN and AUX proteins. Further investigations are needed to gain a comprehensive understanding of the mechanisms behind the interaction between CO and the NO signal in the regulation of the asymmetric stability of PIN and AUX proteins, which are crucial for auxin transport during iron deprivation (Fig. 4).
Figure 4: CO-mediated signaling pathway model in plants under ID
3.4 The Connection between CO, NO, and Auxin in Plant Responses to ID Stress
Iron deficiency triggers an increase in HO activity, leading to the production of CO. CO, in turn, enhances the expression of key genes such as FRO2 and FIT, promoting iron reduction and uptake. In Arabidopsis, CO alters the asymmetric accumulation of PIN1 and PIN2, facilitating polar auxin transport in the root tip and elevating auxin levels. Consequently, this upregulates the expression of FIT1 and FRO2 genes, enhancing iron absorption [84].
Concurrently, the accumulation of NO also promotes an upregulation of FCR activity, a crucial step in iron absorption. The levels of NO significantly increase under iron-deficient conditions, which can be achieved through the enhanced activity of enzymes such as nitric oxide synthase (NOS) [102]. NO exerts a significant influence on Auxin signal transduction, possibly achieved by reducing the accumulation of PIN1 protein and stabilizing the AXR3/IAA17 protein (the latter being an inhibitor of the Auxin signal), contributing to an increased sensitivity of the roots to Auxin.
Auxin plays a crucial role in root elongation and branching, which is closely related to iron absorption in plants. In cucumbers, NO and Auxin can interact to induce the formation of adventitious roots [103]. Furthermore, Auxin can also induce the accumulation of NO, thereby enhancing FCR activity. Therefore, the synergistic interactions among CO, NO, and Auxin regulate a complex array of physiological processes under iron deficiency stress. These processes include iron reduction and uptake, root development, and Auxin signaling. CO, NO, and Auxin may interact by influencing different signaling pathways, thus co-regulating plant responses to iron deficiency. This may involve affecting plant hormone signaling pathways such as gibberellin, ethylene, and abscisic acid, as well as activating relevant signaling protein kinases (MAPK), among others [104]. CO and NO can also work together with Auxin to enhance the plant’s antioxidant defense system. This can be achieved by regulating the expression and activity of antioxidant enzymes, thereby alleviating the oxidative stress caused by iron deficiency.
4 The Use of CO, NO, and Auxins in Plants with Iron Deficiency
CO, NO, and Auxin are crucial signaling molecules that regulate iron absorption in plants, leading to a multitude of effects, enhancing plant resilience, and increasing crop yield [105]. Here are the multiple applications of these signaling molecules in plant iron metabolism:
Adaptation to various environmental stressors [106]: The application of CO, NO, and Auxin can bolster a plant’s ability to adapt to a wide range of environmental stressors, including drought, high temperatures, pests, and soil quality issues. By optimizing iron uptake and distribution, plants are better equipped to cope with these pressures, reducing crop losses, ensuring stable agricultural yields, and contributing to the protection of ecosystems.
Enhancement of nutrient utilization efficiency: CO and NO promote the absorption of iron by plants while simultaneously reducing the demand for other nutrients in crops [107]. Improvement of fruit quality [108]: CO, NO, and Auxin have a significant impact on the size, shape, and sugar content of fruits through their influence on iron absorption and distribution [109]. This is crucial for the quality and commercial value of fruits, vegetables, and nuts. Adjusting the levels of these signaling molecules can improve the overall quality of agricultural produce, making it more competitive in the market. Enhancement of the immune system: CO, NO, and Auxin not only affect iron uptake but also play a role in strengthening a plant’s immune system. They enhance resistance to pathogens [110], reducing the need for pesticides. This is essential for maintaining ecological balance and environmental protection.
In summary, the application of CO, NO, and Auxin in plant iron metabolism offers multiple benefits, including increased crop yields, improved food quality, reduced environmental stress, and a contribution to sustainable agriculture. In the future, research and application of these signaling molecules are expected to continue driving innovation and advancements in the field of agriculture.
Grasses (Poaceae) and non-grass plants employ different strategies when facing iron deficiency. Grasses have the ability to directly uptake trivalent iron ions, while non-grass plants need to employ FCR to convert trivalent iron ions in the soil into divalent iron ions for absorption. The interaction between CO, NO, and auxin enhances FCR activity to cope with iron deficiency stress in plants. ID triggers an increase in HO activity, leading to the production of CO, which, in turn, influences auxin transport by modulating the subcellular distribution of PIN1 proteins. Simultaneously, NO levels surge, enhancing FCR activity and upregulating the expression of crucial genes in plant roots, such as FIT and FRO2. Auxin promotes root growth and, in turn, iron absorption in plants while also stimulating NO accumulation, thereby boosting FCR activity. The process of iron uptake, facilitated by the interaction of CO, NO, and auxin, also relies on the expression of relevant genes, such as FER, FRO, and IRT, among others.
These recent discoveries collectively elucidate the interplay between CO, NO, and auxin, which collectively assist plants in adapting to ID stress. Beyond advancing our understanding of how plants respond to ID, these findings offer hope for potential applications in crop improvement, forestry production, and nutrient management strategies. The future holds exciting prospects for utilizing this knowledge to enhance plant productivity and resilience.
Acknowledgement: Not applicable.
Funding Statement: This research was funded by Open Project of Jiangsu Key Laboratory for Eco-Agricultural Biotechnology around Hongze Lake, Grant Number HZHLAB2201.
Author Contributions: K.H. and Y.L. confirmed the research topic and goals, searched and screened the literature, and wrote the manuscript. Y.R., P.L. and W.A. reviewed and edited the manuscript. P.L. and Y.L. sourced funding and supervised the progress of the manuscript.
Availability of Data and Materials: Data sharing is not applicable to this article as no new data were created or analyzed in this study.
Ethics Approval: Not applicable.
Conflicts of Interest: The authors declare that they have no conflicts of interest to report regarding the present study.
References
1. Rout GR, Sahoo S. Role of iron in plant growth and metabolism. Rev Agric Sci. 2015;3:1–24. [Google Scholar]
2. Li J, Cao X, Jia X, Liu L, Cao H, Qin W, et al. Iron deficiency leads to chlorosis through impacting chlorophyll synthesis and nitrogen metabolism in Areca catechu L. Front Plant Sci. 2021;12:710093. [Google Scholar] [PubMed]
3. Kato T, Kumazaki K, Wada M, Taniguchi R, Nakane T, Yamashita K, et al. Crystal structure of plant vacuolar iron transporter VIT1. Nat Plants. 2019;5(3):308–15. [Google Scholar] [PubMed]
4. Borlotti A, Vigani G, Zocchi G. Iron deficiency affects nitrogen metabolism in cucumber (Cucumis sativus L.) plants. BMC Plant Biol. 2012;12(1):1–15. [Google Scholar]
5. Moradbeygi H, Jamei R, Heidari R, Darvishzadeh R. Investigating the enzymatic and non-enzymatic antioxidant defense by applying iron oxide nanoparticles in Dracocephalum moldavica L. plant under salinity stress. Sci Hortic. 2020;272:109537. [Google Scholar]
6. El-Desouky HS, Islam KR, Bergefurd B, Gao G, Harker T, Abd-El-Dayem H, et al. Nano iron fertilization significantly increases tomato yield by increasing plants’ vegetable growth and photosynthetic efficiency. J Plant Nutr. 2021;44(11):1649–63. [Google Scholar]
7. Hilo A, Shahinnia F, Druege U, Franken P, Melzer M, Rutten T, et al. A specific role of iron in promoting meristematic cell division during adventitious root formation. J Exp Bot. 2017;68(15):4233–47. [Google Scholar] [PubMed]
8. Pontiggia A, de Nisi P, Zocchi G. Effect of iron deficiency on RNA and protein synthesis in cucumber roots. J Plant Nutr. 2003;26(10–11):2177–86. [Google Scholar]
9. Prity SA, El-Shehawi AM, Elseehy MM, Tahura S, Kabir AH. Early-stage iron deficiency alters physiological processes and iron transporter expression, along with photosynthetic and oxidative damage to sorghum. Saudi J Biol Sci. 2021;28(8):4770–7. [Google Scholar] [PubMed]
10. Slatni T, Krouma A, Aydi S, Chaiffi C, Gouia H, Abdelly C, et al. Growth, nitrogen fixation and ammonium assimilation in common bean (Phaseolus vulgaris L) subjected to iron deficiency. Plant Soil. 2008;312:49–57. [Google Scholar]
11. Ryter SW, Otterbein LE, Morse D, Choi AMK. Heme oxygenase/carbon monoxide signaling path-ways: regulation and functional significance. In: Vallyathan V, Shi X, Castranova V, editors. Oxygen/nitrogen radicals: cell injury and disease. Boston, MA: Springer; 2002. p. 249–63. doi:https://doi.org/10.1007/978-1-4615-1087-1_29. [Google Scholar] [CrossRef]
12. Li H, Chen H, Chen L, Wang C. The role of hydrogen sulfide in plant roots during development and in response to abiotic stress. Int J Mol Sci. 2022;23(3):1024. [Google Scholar] [PubMed]
13. Mathur P, Tripathi DK, Baluška F, Mukherjee S. Auxin-mediated molecular mechanisms of heavy metal and metalloid stress regulation in plants. Environ Exp Bot. 2022;196:104796. [Google Scholar]
14. Liu J, Wang J, Wang Z, Li M, Liang C, Yang Y, et al. Alleviation of iron deficiency in pear by ammonium nitrate and nitric oxide. BMC Plant Biol. 2022;22(1):1–11. [Google Scholar]
15. Filiz E, Kurt F. FIT (Fer-like iron deficiency-induced transcription factor) in plant iron homeostasis: genome-wide identification and bioinformatics analyses. J Plant Biochem. 2019;28:143–57. [Google Scholar]
16. Lei R, Li Y, Cai Y, Li C, Pu M, Lu C, et al. bHLH121 functions as a direct link that facilitates the activation of FIT by bHLH IVc transcription factors for maintaining Fe homeostasis in Arabidopsis. Mol Plant. 2020;13(4):634–49. [Google Scholar] [PubMed]
17. Feng L, Wei L, Liu Y, Ren J, Liao W. Carbon monoxide/heme oxygenase system in plant: roles in abiotic stress response and crosstalk with other signals molecules. Nato Sci S A Lif Sci. 2023;138–139:51–63. [Google Scholar]
18. Khan M, Ali S, Al Azzawi TNI, Yun BW. Nitric oxide acts as a key signaling molecule in plant development under stressful conditions. Int J Mol Sci. 2023;24(5):4782. [Google Scholar] [PubMed]
19. Du Z, Wang X, Zhang X, Gu Z, Fu X, Gan S, et al. X-ray-triggered carbon monoxide and manganese dioxide generation based on scintillating nanoparticles for cascade cancer radiosensitization. Angewandte Chem Int Ed. 2023;62(23):e202302525. [Google Scholar]
20. Lin M, Xian H, Chen Z, Wang S, Liu M, Liang W, et al. MCM8-mediated mitophagy protects vascular health in response to nitric oxide signaling in a mouse model of Kawasaki disease. Nat Cardiovasc Res. 2023;2(8):778–92. [Google Scholar]
21. Hussain A, Shah F, Ali F, Yun BW. Role of nitric oxide in plant senescence. Front Plant Sci. 2022;13:851631. [Google Scholar] [PubMed]
22. Singh N, Bhatla SC. Heme oxygenase-nitric oxide crosstalk-mediated iron homeostasis in plants under oxidative stress. Free Radic Bio Med. 2022;182:192–205. [Google Scholar] [PubMed]
23. Mir IR, Rather BA, Sehar Z, Masood A, Khan NA. Nitric oxide in co-ordination with nitrogen reverses cadmium-inhibited photosynthetic activity by interacting with ethylene synthesis, strengthening the antioxidant system, and nitrogen and sulfur assimilation in mustard (Brassica juncea L.). Sci Horticult. 2023;314(8):111958. doi:https://doi.org/10.1016/j.scienta.2023.111958. [Google Scholar] [CrossRef]
24. Mahawar L, Ramasamy KP, Pandey A, Prasad SM. Iron deficiency in plants: an update on homeostasis and its regulation by nitric oxide and phytohormones. Plant Growth Regul. 2023;100(2):283–99. [Google Scholar]
25. He H, He L. The role of carbon monoxide signaling in the responses of plants to abiotic stresses. Nitric Oxide. 2014;42:40–3. [Google Scholar] [PubMed]
26. Shang JX, Li X, Li C, Zhao L. The role of nitric oxide in plant responses to salt stress. Int J Mol Sci. 2022;23(11):6167. [Google Scholar] [PubMed]
27. Mariyam S, Bhardwaj R, Khan NA, Sahi SV, Seth CS. Review on nitric oxide at the forefront of rapid systemic signaling in mitigation of salinity stress in plants: crosstalk with calcium and hydrogen peroxide. Plant Sci. 2023;336:111835. [Google Scholar] [PubMed]
28. Roychoudhry S, Kepinski S. Auxin in root development. Cold Spring Harb Perspect Biol. 2022;14(4):a039933. [Google Scholar] [PubMed]
29. Gorelova V. Gateway to morphogenesis: TIR1 auxin receptor is essential for cellular differentiation and organ formation in Marchantia polymorpha. USA: Oxford University Press; 2023. [Google Scholar]
30. Zhang Y, Yu J, Xu X, Wang R, Liu Y, Huang S, et al. Molecular mechanisms of diverse auxin responses during plant growth and development. Int J Mol Sci. 2022;23(20):12495. [Google Scholar] [PubMed]
31. Sanchez-Corrionero A, Sánchez-Vicente I, Arteaga N, Manrique-Gil I, Gómez-Jiménez S, Torres-Quezada I, et al. Fine-tuned nitric oxide and hormone interface in plant root development and regeneration. J Exp Bot. 2023;74(19):6104–18. [Google Scholar] [PubMed]
32. Wang M, Liao W. Carbon monoxide as a signaling molecule in plants. Front Plant Sci. 2016;7(1665):572. [Google Scholar] [PubMed]
33. Ryter SW, Otterbein LE. Carbon monoxide in biology and medicine. Bioessays. 2004;26(3):270–80. [Google Scholar] [PubMed]
34. Ryter SW. Heme oxygenase-1: an anti-inflammatory effector in cardiovascular, lung, and related metabolic disorders. Antioxidants. 2022;11(3):555. [Google Scholar] [PubMed]
35. Xie YJ, Xu S, Han B, Wu MZ, Yuan XX, Han Y, et al. Evidence of Arabidopsis salt acclimation induced by up-regulation of HY1 and the regulatory role of RbohD-derived reactive oxygen species synthesis. Plant J. 2011;66(2):280–92. [Google Scholar] [PubMed]
36. Corpas FJ, González-Gordo S, Palma JM. NO source in higher plants: present and future of an unresolved question. Trends Plant Sci. 2022;27(2):116–9. [Google Scholar] [PubMed]
37. Chen L, Sun S, Song CP, Zhou JM, Li J, Zuo J, et al. Nitric oxide negatively regulates gibberellin signaling to coordinate growth and salt tolerance in Arabidopsis. J Genet Genomics. 2022;49(8):756–65. [Google Scholar] [PubMed]
38. Zhou Y, Wang L, Rubio MC, Pérez-Rontomé C, Zhou Y, Qi Y, et al. Heme catabolism mediated by heme oxygenase in uninfected interstitial cells enables efficient symbiotic nitrogen fixation in Lotus japonicus nodules. New Phytol. 2023;240(6):1989–2006. doi:https://doi.org/10.1111/nph.19074. [Google Scholar] [PubMed] [CrossRef]
39. Liu M, Wei J, Wei L, Biao G. S-nitrosylation of ACO homolog 4 improves ethylene synthesis and salt tolerance in tomato. New Phytol. 2023;239(1):159–73. doi:https://doi.org/10.1111/nph.18928. [Google Scholar] [PubMed] [CrossRef]
40. Jahnová J, Luhová L, Petřivalský M. S-nitrosoglutathione reductase—the master regulator of protein S-nitrosation in plant NO signaling. Plants. 2019;8(2):48. [Google Scholar] [PubMed]
41. Badiani E, Pasqualini S, Ciaffi M, Paolacci AR, Sorgonà A, Badiani M. Nitric oxide in plants: a molecule with dual roles. New Jersey, USA: John Wiley & Sons; 2022. 118–47 p. doi:https://doi.org/10.1002/9781119800156.ch7. [Google Scholar] [CrossRef]
42. He NY, Chen LS, Sun AZ, Zhao Y, Yin SN, Guo FQ, et al. A nitric oxide burst at the shoot apex triggers a heat-responsive pathway in Arabidopsis. Nat Plants. 2022;8(4):434–50. [Google Scholar] [PubMed]
43. Kaya C, Higgs D, Ashraf M, Alyemeni MN, Ahmad P. Integrative roles of nitric oxide and hydrogen sulfide in melatonin-induced tolerance of pepper (Capsicum annuum L.) plants to iron deficiency and salt stress alone or in combination. Physiol Plantarum. 2020;168(2):256–77. [Google Scholar]
44. Stitz M, Kuster D, Reinert M, Schepetilnikov M, Berthet B, Reyes-Hernández J, et al. TOR acts as a metabolic gatekeeper for auxin-dependent lateral root initiation in Arabidopsis thaliana. Embo J. 2023;42(10):e111273. [Google Scholar] [PubMed]
45. Hu J, Li X, Sun TP. Four class A AUXIN RESPONSE FACTORs promote tomato fruit growth despite suppressing fruit set. Nat Plants. 2023;9(5):1–14. [Google Scholar]
46. Gomes G, Scortecci K. Auxin and its role in plant development: structure, signalling, regulation and response mechanisms. Plant Biol. 2021;23(6):894–904. [Google Scholar] [PubMed]
47. Xue C, Li W, Shen R, Lan P. Impacts of iron on phosphate starvation-induced root hair growth in Arabidopsis. Plant Cell Environ. 2023;46(1):215–38. [Google Scholar] [PubMed]
48. Cao X, Yang H, Shang C, Ma S, Liu L, Cheng J, et al. The roles of auxin biosynthesis YUCCA gene family in plants. Int J Mol Sci. 2019;20(24):6343. [Google Scholar] [PubMed]
49. Tivendale ND, Ross JJ, Cohen JD. The shifting paradigms of auxin biosynthesis. Trends Plant Sci. 2014;19(1):44–51. [Google Scholar] [PubMed]
50. Xu Z, Xue H. The role of plant hormones in the embryo and seed development. Netherlands: Elsevier; 2012. 382–395 p. [Google Scholar]
51. Dong L, Ma Y, Chen CY, Shen L, Sun W, Cui G, et al. Identification and characterization of auxin/IAA biosynthesis pathway in the rice blast fungus Magnaporthe oryzae. J Fungi. 2022;8(2):208. [Google Scholar]
52. Rajagopal R, Larsen P. Metabolism of indole-3-acetaldoxime in plants. Planta. 1972;103:45–54. [Google Scholar] [PubMed]
53. Duca D, Rose DR, Glick BR. Characterization of a nitrilase and a nitrile hydratase from Pseudomonas sp. Strain UW4 that converts indole-3-acetonitrile to indole-3-acetic acid. Appl Environ Microb. 2014;80(15):4640–9. [Google Scholar]
54. Zhao Y. Auxin biosynthesis and its role in plant development. Annu Rev Plant Biol. 2014;61:49–64. doi:https://doi.org/10.1146/annurev-arplant-042809-112308. [Google Scholar] [PubMed] [CrossRef]
55. Westfall CS, Herrmann J, Chen Q, Wang S, Jez JM. Modulating plant hormones by enzyme action: the GH3 family of acyl acid amido synthetases. Plant Signal Behav. 2010;5(12):1607–12. [Google Scholar] [PubMed]
56. Armstrong JI, Yuan S, Dale JM, Tanner VN, Theologis A. Identification of inhibitors of auxin transcriptional activation by means of chemical genetics in Arabidopsis. Proc Natl Acad Sci USA. 2004;101(41):14978–83. [Google Scholar] [PubMed]
57. Yang L, Ji J, Harris-Shultz KR, Wang H, Wang H, Abd-Allah EF, et al. The dynamic changes of the plasma membrane proteins and the protective roles of nitric oxide in rice subjected to heavy metal cadmium stress. Front Plant Sci. 2016;7:190. [Google Scholar] [PubMed]
58. Hongwei J, Yang X, Emenecker RJ. Nitric oxide-mediated S-nitrosylation of IAA17 protein in intrinsically disordered region represses auxin signaling. J Genet Genomics. 2023;50(7):473–85. doi:https://doi.org/10.1016/j.jgg.2023.05.001. [Google Scholar] [PubMed] [CrossRef]
59. Dharmasiri N, Estelle M. Auxin signaling and regulated protein degradation. Trends Plant Sci. 2023;9(6):302–8. doi:https://doi.org/10.1016/j.tplants.2004.04.003. [Google Scholar] [PubMed] [CrossRef]
60. Kim YX, Kim TJ, Lee Y, Lee S, Lee D, Oh TK, et al. Metabolite profiling and mineral nutrient analysis from the leaves and roots of bell pepper (Capsicum annuum L. var. angulosum) grown under macronutrient mineral deficiency. Appl Biol Chem. 2018;61:661–71. [Google Scholar]
61. Nath M, Tuteja N. NPKS uptake, sensing, and signaling and miRNAs in plant nutrient stress. Protoplasma. 2016;253:767–86. [Google Scholar] [PubMed]
62. Yiqin W, Zhenfeng T, Li H, Wei W, Fan X, Sun K, et al. An activated form of NB-ARC protein RLS1 functions with cysteine-rich receptor-like protein RMC to trigger cell death in rice. Plant Commun. 2023;4(2):100459. [Google Scholar]
63. Qi Y, Wang S, Shen C, Zhang S, Chen Y, Xu Y, et al. OsARF12, a transcription activator on auxin response gene, regulates root elongation and affects iron accumulation in rice (Oryza sativa). New Phytol. 2012;193(1):109–20. [Google Scholar] [PubMed]
64. Bacaicoa E, Mora V, Zamarreño Á. M, Fuentes M, Casanova E, García-Mina JM. Auxin: a major player in the shoot-to-root regulation of root Fe-stress physiological responses to Fe deficiency in cucumber plants. Plant Physiol Biochem. 2011;49(5):545–56. [Google Scholar] [PubMed]
65. García-Mina JM, Bacaicoa E, Fuentes M, Casanova E. Fine regulation of leaf iron use efficiency and iron root uptake under limited iron bioavailability. Plant Sci. 2013;198:39–45. [Google Scholar]
66. Jia Z, Giehl RF, von Wirén N. Local auxin biosynthesis acts downstream of brassinosteroids to trigger root foraging for nitrogen. Nat Commun. 2021;12(1):5437. [Google Scholar] [PubMed]
67. Jia H, Zhang S, Wang L, Yang Y, Zhang H, Cui H, et al. OsPht1;8, a phosphate transporter, is involved in auxin and phosphate starvation response in rice. J Exp Bot. 2017;68(18):5057–68. [Google Scholar] [PubMed]
68. Giri J, Bhosale R, Huang G, Pandey BK, Parker H, Zappala S, et al. Rice auxin influx carrier OsAUX1 facilitates root hair elongation in response to low external phosphate. Nat Commun. 2018;9(1):1408. [Google Scholar] [PubMed]
69. Sun H, Guo X, Xu F, Wu D, Zhang X, Lou M, et al. Overexpression of OsPIN2 regulates root growth and formation in response to phosphate deficiency in rice. Int J Mol Sci. 2019;20(20):5144. [Google Scholar] [PubMed]
70. Sun H, Tao J, Bi Y, Hou M, Lou J, Chen X, et al. OsPIN1b is involved in rice seminal root elongation by regulating root apical meristem activity in response to low nitrogen and phosphate. Sci Rep. 2018;8(1):13014. [Google Scholar] [PubMed]
71. Colombo C, Palumbo G, He JZ, Pinton R, Cesco S. Review on iron availability in soil: interaction of Fe minerals, plants, and microbes. J Soil Sediment. 2014;14(3):538–48. [Google Scholar]
72. Singh B, Gilkes R. Properties and distribution of iron oxides and their association with minor elements in the soils of South-Western Australia. J Soil Sci. 1992;43(1):77–98. [Google Scholar]
73. Lindsay W. Soil and plant relationships associated with iron deficiency with emphasis on nutrient interactions. J Plant Nutr. 1984;7(1–5):489–500. [Google Scholar]
74. Römheld V, Marschner H. Evidence for a specific uptake system for iron phytosiderophores in roots of grasses. Plant Physiol. 1986;80(1):175–80. [Google Scholar]
75. Li S, Song Z, Liu X, Zhou X, Yang W, Chen J, et al. Mediation of zinc and iron accumulation in maize by ZmIRT2, a novel iron-regulated transporter. Plant Cell Physiol. 2022;63(4):521–34. [Google Scholar] [PubMed]
76. Liang G. Iron uptake, signaling, and sensing in plants. Plant Commun. 2022;3(5):100349. doi:https://doi.org/10.1016/j.xplc.2022.100349. [Google Scholar] [PubMed] [CrossRef]
77. Zhang X, Luo H, Xu Z, Zhu Y, Ji A, Song J, et al. Genome-wide characterisation and analysis of bHLH transcription factors related to tanshinone biosynthesis in Salvia miltiorrhiza. Sci Rep-UK. 2015;5(1):11244. [Google Scholar]
78. Akmakjian GZ, Riaz N, Guerinot ML. Photoprotection during iron deficiency is mediated by the bHLH transcription factors PYE and ILR3. Proc Natl Acad Sci USA. 2021;118(40):e2024918118. [Google Scholar] [PubMed]
79. Graziano M, Lamattina L. Nitric oxide accumulation is required for molecular and physiological responses to iron deficiency in tomato roots. Plant J. 2007;52(5):949–60. [Google Scholar] [PubMed]
80. Chen YH, Chao YY, Hsu YY, Hong CY, Kao CH. Heme oxygenase is involved in nitric oxide-and auxin-induced lateral root formation in rice. Plant Cell Rep. 2012;31(6):1085–91. [Google Scholar] [PubMed]
81. Xuan W, Xu S, Li M, Han B, Zhang B, Zhang J, et al. Nitric oxide is involved in hemin-induced cucumber adventitious rooting process. J Plant Physiol. 2012;169(11):1032–9. [Google Scholar] [PubMed]
82. Corpas FJ, Rodríguez-Ruiz M, Muñoz-Vargas MA, González-Gordo S, Reiter RJ, Palma JM. Interactions of melatonin, reactive oxygen species, and nitric oxide during fruit ripening: an update and prospective view. J Exp Bot. 2022;73(17):5947–60. [Google Scholar] [PubMed]
83. Sanz L, Albertos P, Mateos I, Sánchez-Vicente I, Lechón T, Fernández-Marcos M, et al. Nitric oxide (NO) and phytohormones crosstalk during early plant development. J Exp Bot. 2015;66(10):2857–68. [Google Scholar] [PubMed]
84. Yang L, Ji J, Wang H, Harris-Shultz KR, Abd_Allah EF, Luo Y, et al. Carbon monoxide interacts with auxin and nitric oxide to cope with iron deficiency in Arabidopsis. Front Plant Sci. 2016;7:112. [Google Scholar] [PubMed]
85. Huang D, Dai W. Two iron-regulated transporter (IRT) genes showed differential expression in poplar trees under iron or zinc deficiency. J Plant Physiol. 2015;186:59–67. [Google Scholar] [PubMed]
86. Ding H, Duan L, Li J, Yan H, Zhao M, Zhang F, et al. Cloning and functional analysis of the peanut iron transporter AhIRT1 during iron deficiency stress and intercropping with maize. J Plant Physiol. 2010;167(12):996–1002. [Google Scholar] [PubMed]
87. Chen WW, Yang JL, Qin C, Jin CW, Mo JH, Ye T, et al. Nitric oxide acts downstream of auxin to trigger root ferric-chelate reductase activity in response to iron deficiency in Arabidopsis. Plant Physiol. 2010;154(2):810–9. [Google Scholar] [PubMed]
88. Meiser J, Lingam S, Bauer P. Posttranslational regulation of the iron deficiency basic helix-loop-helix transcription factor FIT is affected by iron and nitric oxide. Plant Physiol. 2011;157(4):2154–66. [Google Scholar] [PubMed]
89. Lin A, Wang Y, Tang J, Xue P, Li C, Liu L, et al. Nitric oxide and protein S-nitrosylation are integral to hydrogen peroxide-induced leaf cell death in rice. Plant Physiol. 2012;158(1):451–64. [Google Scholar] [PubMed]
90. Wang P, Du Y, Hou YJ, Zhao Y, Hsu CC, Yuan F, et al. Nitric oxide negatively regulates abscisic acid signaling in guard cells by S-nitrosylation of OST1. Proc Natl Acad Sci USA. 2015;112(2):613–8. [Google Scholar] [PubMed]
91. Bai XG, Chen JH, Kong XX, Todd CD, Yang YP, Hu XY, et al. Carbon monoxide enhances the chilling tolerance of recalcitrant Baccaurea ramiflora seeds via nitric oxide-mediated glutathione homeostasis. Free Radical Bio Med. 2012;53(4):710–20. [Google Scholar]
92. Liping Z, Hongbo S, Xiaohua L, Zhaopu L. Gene regulation of iron-deficiency responses is associated with carbon monoxide and heme oxydase 1 in Chlamydomonas reinhardtii. PLoS One. 2013;8(1):e53835. [Google Scholar] [PubMed]
93. Horst BG, Stewart EM, Nazarian AA, Marletta MA. Characterization of a carbon monoxide-activated soluble guanylate cyclase from Chlamydomonas reinhardtii. Biochem. 2019;58(17):2250–9. [Google Scholar]
94. Li H, Song JB, Zhao WT, Yang ZM. AtHO1 is involved in iron homeostasis in an NO-dependent manner. Plant Cell Physiol. 2013;54(7):1105–17. [Google Scholar] [PubMed]
95. Kong WW, Zhang LP, Guo K, Liu ZP, Yang ZM. Carbon monoxide improves adaptation of Arabidopsis to iron deficiency. Plant Biotechnol J. 2010;8(1):88–99. [Google Scholar] [PubMed]
96. Briat JF, Curie C, Gaymard F. Iron utilization and metabolism in plants. Curr Opin Plant Biol. 2007;10(3):276–82. [Google Scholar] [PubMed]
97. Tan X, Yang X, Xie Y, Xiao H, Liu M, Wu L, et al. Effects of different foliar iron applications on activity of ferric chelate reductase and concentration of iron in sweet potato (Ipomoea batatas). Crop Pasture Sci. 2019;70(4):359–66. [Google Scholar]
98. Lin XY, Ye YQ, Fan SK, Jin CW, Zheng SJ. Increased sucrose accumulation regulates iron-deficiency responses by promoting auxin signaling in Arabidopsis plants. Plant Physiol. 2016;170(2):907–20. [Google Scholar] [PubMed]
99. Liu Y, von Wirén N. Integration of nutrient and water availabilities via auxin into the root developmental program. Curr Opin Plant Biol. 2022;65:102117. [Google Scholar] [PubMed]
100. Guo G, Yu T, Zhang H, Chen M, Dong W, Zhang S, et al. Evidence that PbrSAUR72 contributes to iron deficiency tolerance in pears by facilitating iron absorption. Plants. 2023;12(11):2173. [Google Scholar] [PubMed]
101. Weng X, Zhu L, Yu S, Liu Y, Ru Y, Zhang Z, et al. Carbon monoxide promotes stomatal initiation by regulating the expression of two EPF genes in Arabidopsis cotyledons. Front Plant Sci. 2022;13:1029703. [Google Scholar] [PubMed]
102. Buet A, Simontacchi M. Nitric oxide and plant iron homeostasis. Ann N Y Acad Sci. 2015;1340(1):39–46. [Google Scholar] [PubMed]
103. Lanteri ML, Pagnussat GC, Lamattina L. Calcium and calcium-dependent protein kinases are involved in nitric oxide-and auxin-induced adventitious root formation in cucumber. J Exp Bot. 2006;57(6):1341–51. [Google Scholar] [PubMed]
104. Pagnussat GC, Lanteri Ma L, Lombardo Ma C, Lamattina L. Nitric oxide mediates the indole acetic acid induction activation of a mitogen-activated protein kinase cascade involved in adventitious root development. Plant Physiol. 2004;135(1):279–86. [Google Scholar] [PubMed]
105. Zaman M, Kurepin LV, Catto W, Pharis RP. Enhancing crop yield with the use of N-based fertilizers co-applied with plant hormones or growth regulators. J Sci Food Agr. 2015;95(9):1777–85. [Google Scholar]
106. Ganguly R, Sarkar A, Acharya K, Keswani C, Minkina T, Mandzhieva S, et al. The role of no in the amelioration of heavy metal stress in plants by individual application or in combination with phytohormones, especially auxin. Sustain. 2022;14(14):8400. [Google Scholar]
107. Guo K, Xia K, Yang ZM. Regulation of tomato lateral root development by carbon monoxide and involvement in auxin and nitric oxide. J Exp Bot. 2008;59(12):3443–52. [Google Scholar] [PubMed]
108. Roussos PA, Tassis A. Effects of girdling, nitrogen, zinc and auxin foliar spray applications on mandarin fruit “Nova” quality characteristics. Emir J Food Agric. 2011;23(5):431–9. [Google Scholar]
109. Dhal S, Pal H. Role of nitric oxide in fruit ripening. In Nitric oxide in plant biology. Netherlands: Elsevier; 2022. p. 707–752. [Google Scholar]
110. Agurla S, Gayatri G, Raghavendra AS. Nitric oxide as a secondary messenger during stomatal closure as a part of plant immunity response against pathogens. Nitric Oxide. 2014;43:89–96. [Google Scholar] [PubMed]
Cite This Article
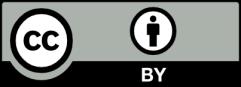