Open Access
ARTICLE
Mitochondria are an important target of photobiomodulation in cardiomyocytes
1 Department of Laboratory Medicine at the Fourth Affiliated Hospital, and Department of Pharmacy at the Second Affiliated Hospital, Harbin Medical University, Harbin, 150081, China
2 Department of Pharmacology (State-Province Key Laboratories of Biomedicine-Pharmaceutics of China, Key Laboratory of Cardiovascular Research, Ministry of Education) at College of Pharmacy, Harbin Medical University, Harbin, 150081, China
3 Northern Translational Medicine Research and Cooperation Center, Heilongjiang Academy of Medical Sciences, Harbin Medical University, Harbin, 150081, China
* Corresponding Author: YU LIU. Email:
(This article belongs to the Special Issue: Mitochondrial Form and Function)
BIOCELL 2022, 46(12), 2637-2644. https://doi.org/10.32604/biocell.2022.021033
Received 24 December 2021; Accepted 26 April 2022; Issue published 10 August 2022
Abstract
Photobiomodulation (PBM) has been shown to delay the pathological process of heart failure, but the exact mechanism of action is not clear. Mitochondria occupy one-third of the volume of mammalian cardiomyocytes (CMs) and are central transport stations for CM energy metabolism. Therefore, in this study, we explored the regulatory effects of 630 nm light-emitting diodes (LED-Red) on the mitochondria of CMs. The results show that LED-Red-based PBM promotes adenosine triphosphate (ATP) synthesis by upregulating the expression of glycolipid metabolizing enzymes. Correspondingly, there was an improvement in the activity of succinate dehydrogenase (SDH), a key enzyme in the mitochondrial electron transport chain, and the mitochondrial membrane potential. Meanwhile, LED-Red affected the state of mitochondrial oxidative stress and promoted the generation of reactive oxygen species (ROS), but the increased ROS production did not damage the CMs. In addition, mitochondrial division and fusion were also affected by the stimulation of LED-Red. Finally, PBM treatment led to a significant increase in transcript levels of mitochondrial transcription factor A (TFAM), which controls the stability of the mitochondrial genome. Collectively, irradiation with LEDs at 630 nm played a regulatory role in mitochondrial function, suggesting that mitochondria appear to be the recipients of PBM treatment. This study provides more insights into the mechanisms underlying PBM treatment in heart diseases.Keywords
Photobiomodulation has been shown to exert many regulatory effects on the physiological activities of a multitude of tissues and organs (Willis et al., 2018; Wang et al., 2019; Mussttaf et al., 2019; Vladimirov et al., 2004). Light sources of various wavelengths in the visible light range modulate the physiology differently depending on their photon specificity. Blue light has been shown to inhibit tumor tissue proliferation and invasion but has a proliferative effect on neural stem cells (Teixeira et al., 2018; Wang et al., 2019). A clinical trial study showed that green light has the same inhibitory effect on neonatal bilirubin as blue light (Kuboi et al., 2019). In addition to this, red light demonstrates more biological modulation in PBM. The light source at 630 nm has been shown to promote tissue damage repair, deep tissue anti-inflammatory regulation, and even cell proliferation and differentiation (Barolet et al., 2009; Chaves et al., 2014; Jenkins et al., 2010; Hentschke et al., 2013). Several studies have shown that the myocardium is also receptive to red light stimulation signals and is involved in myocardial electrophysiological activity, promoting myocardial endothelial revascularization and delaying the occurrence of heart failure (Hentschke et al., 2013; Burton et al., 2015; Kuboi et al., 2019). However, the mechanisms by which red light modulates the physiological activity of myocardial tissue are not clear.
Mitochondria are the “energy factories” of CMs and provide a constant supply of ATP for excitation-coupled contraction through oxidative phosphorylation of fatty acids and transmission of electron respiratory chains. CMs have the largest number of mitochondria, with approximately 5,000–8,000 mitochondria per cell. Thus, the homeostasis of mitochondria is important for the physiological and pathological activity of CMs. Ischemic cardiomyopathy is a precursor to the development of heart failure, and mitochondrial dysfunction plays a key role in the pathology of ischemic heart disease (Walters et al., 2012). The reperfusion phase of ischemia-reperfusion causes mitochondrial damage, leading to inhibition of the mitochondrial respiratory electron transport chain, impairment of mitochondrial energy metabolism, production of reactive oxygen species, loss of mitochondrial membrane potential, and increased mitochondrial necrosis and apoptosis (Ambrosio et al., 1993; Murphy and Steenbergen, 2008; Huss and Kelly, 2005). Therefore, the regulation of mitochondria as a potential therapeutic target in ischemic cardiomyopathy seems to be implementable.
Currently, the impact of PBM on the pathophysiology of the heart remains inadequate. This is not conducive to the development of PBM for cardiovascular applications. Therefore, this study aimed to investigate the effect of LEDs irradiation at 630 nm on the function of mammalian mitochondrial CMs and homeostasis.
This study was approved by the Medical Ethics Committee of the Second Affiliated Hospital of Harbin Medical University (No. KY2021-363). First, hearts of newborn Kunming mice were isolated and subjected to trypsin (T1300, Solarbio, Beijing, China) digestion. The digest was centrifuged (4°C, 1500 rpm, 5 min) after filtration, and the precipitate was collected. The precipitate was added to the complete culture solution, including Dulbecco’s modified Eagle’s medium (DMEM; D6429; Sigma, USA), 10% fetal bovine serum (Gibco, Grand Island, USA), and 1 × penicillin and streptomycin (Life Technologies, Grand Island, USA), spread evenly, transferred to cell bottles, and incubated at 37°C, and 5% CO2, in 95% humid air for 90 min. The upper layer of unadhered cells was collected, and the cell density was adjusted by adding the culture solution. This was then spread to a plate covered with gelatin wells and incubated in an incubator for 24 h before treatment.
A 630 nm light emitting diode with a spot area of about 10 cm2 was placed vertically above the cell well plate. Next, the power density of the light source was measured using a light source power density meter, and the vertical distance between the light source and the cell well plate was adjusted so that the final power density was maintained at 2.5 mW/cm2 and irradiated for 10 min. The Non-LED group was illuminated under the same environmental conditions as the LED-Red group but without turning on the switch of the light source.
Estimation of adenosine triphosphate (ATP) content
To analyze cellular ATP content, the ATP assay kit (S0027, Beyotime, Beijing, China) was used. The culture solution was aspirated from the cell well plate, and 200 µL of lysate were added to each well. After lysis, the mix was centrifuged at 4°C and 12000 g for 5 min, and the supernatant was removed for subsequent assays. An appropriate amount of ATP assay reagent was adjusted according to the manufacturer’s instructions. Then, 100 µL of ATP assay working solution was added to the assay wells and left at room temperature for 3–5 min. To the test wells, 20 µL of the sample was added, mixed rapidly, and the RLU value was measured with a luminometer after an interval of at least 2 s.
Lactate dehydrogenase activity (LDH) estimation
The working assay solution was prepared according to the SDH assay reagent instructions (BC0955, Solarbio, Beijing, China). After cell digestion and centrifugation, the supernatant was aspirated and used to detect SDH activity. The absorbance values were measured at 600 nm on a visible spectrophotometer, and the SDH activity was calculated and analyzed for each group.
Determination of mitochondrial membrane potential (MMP)
CMs were cultured in 12-well plates, and the mitochondrial membrane potential was analyzed using the JC-1 assay kit (C2003S, Beyotime, Beijing, China). After light stimulation, the culture solution in the well plates was discarded, and the cells were washed three times with PBS. Then, the JC-1 solution was added, incubated for 20 min at 37°C, washed again with PBS, and the changes in mitochondrial membrane potential were observed under fluorescence microscopy. The red fluorescence represents an increase, and the green fluorescence indicates a decrease in mitochondrial membrane potential. Then, the mitochondrial membrane potential was quantified by fluorescence analysis using the software image J. The red and green fluorescence values were measured separately for each group, and then the red fluorescence value/sum of red and green fluorescence was calculated as the relative increase of mitochondrial membrane potential for each group.
Reactive oxygen species estimation (ROS)
CMs were inoculated in 96-well plates and ROS were detected using the Reactive Oxygen Species Assay Kit (S0033S, Beyotime, Beijing, China). The assay was performed according to the manufacturer’s instructions. Then, the cells were washed three times with PBS, and DMEM and DCFH-DA probes were prepared in a ratio of 1:1000. Appropriate volumes of probes were added to the well plates and incubated for 20 min at 37°C. Excess unloaded probes were then removed, and the fluorescence of each group was detected using a fluorescent enzyme marker.
Terminal deoxynucleotidyl transferase-mediated dUTP nick-end labeling (TUNEL) staining
The TUNEL Apoptosis Assay Kit (C1089, Beyotime, Beijing, China) was used to analyze apoptosis. CMs were first washed once with PBS after LED-Red stimulation. The cells were fixed in 4% paraformaldehyde for 30 min and then washed once with PBS. Next, the cells were permeabilized with PBS containing 0.3% Triton X-100 for 90 min. 50 µL TUNEL assay solution was added to each well according to the manufacturer’s instructions and incubated for 60 min at 37°C in a dark environment. Finally, the cell nuclei were labeled with DAPI, and apoptosis was observed by fluorescence microscopy.
Cells cultured in glass dishes received LED-Red stimulation. The culture medium was removed, and the cells were washed three times with Hanks buffer. Cells were incubated in 500× MitoTracker (22675, AAT Bioquest, California, USA) stain at 37°C with 5% CO2 for 40 min; then, the stain was discarded. Hanks buffer and Hoest3342 stain were added to re-stain and label the nuclei. Mitochondrial division and fusion were observed by a confocal laser scanning microscope (FV10i; Olympus, Japan). Labeled mitochondria appeared green, and the labeled nuclei appeared blue.
Quantitative real-time-polymerase chain reaction (qRT-PCR)
Total RNA of CMs was extracted using TRIzol reagent (Invitrogen, California, USA). The RNA samples were then reverse transcribed to cDNA. SYBR Green PCR Master Mix (Applied Biosystems, USA) was used for qRT-PCR. The sequences of the main primers are indicated in Table S1.
Cells were scraped by adding an appropriate volume (20–60 µL) of cell lysate depending on cell density and state. Protein concentration was determined using the BCA Protein Concentration Assay Kit (P0012, Beyotime, Beijing, China). An appropriate concentration of SDS-polyacrylamide gel was used for electrophoresis according to the corresponding molecular weight of the incubated antibody. Membranes were then blocked in 5% non-fat milk for 90 min at room temperature and incubated with antibody against Platelet glycoprotein 4 (CD36; 1:1000; YT5585, Immunoway, USA), Recombinant Carnitine Palmitoyltransferase 1A (CPT1A; 1:1000; DF12004, Affinity, USA), Phosphorylated Dynamin-related protein 1 (p-DRP1; 1:500; YP1318, Immunoway, USA), Peroxisome proliferator-activated receptor gamma coactivator 1-alp (PGC-1α; 1:1000; YM0519, Immunoway, USA), Solute carrier family 2 facilitated glucose transporter member 4 (Glut4; 1:1000; YT5523, Immunoway, USA), Transcription factor A mitochondrial (TFAM; 1:1000; YT2916, Immunoway, USA), and pyruvate dehydrogenase kinase isozyme 4 (PDK4; 1:1000; DF7169; Affinity, USA) 4°C overnight. The membrane was finally incubated with horseradish peroxidase (HRP)-conjugated secondary antibody for 1 h at room temperature and scanned by Odyssey (LI-COR Biosciences, Lincoln, NE, USA).
Data are expressed by means ± SEM. Comparisons between two groups were made using Student’s t-test, and comparisons between multiple groups were made using one-way ANOVA and Bonferroni-corrected t-test (version 8.0, GraphPad Software, Inc., USA). P values < 0.05 were considered statistically significant.
630 nm light emitting diodes regulates homeostatic activity within the mitochondria of cardiomyocytes
Mitochondria are subcellular organelles that serve as the main source of energy supply for CMs. The efficiency and amount of ATP synthesis are critical for the contractile activity of CMs. Therefore, we irradiated mammalian CMs using 630 nm light-emitting diodes. The results showed that low-energy LED-Red irradiation for 10 min led to an increase in intracellular ATP content (Fig. 1A). Among the key enzymes that regulate the process of the mitochondrial tricarboxylic acid cycle, SDH is the only multi-subunit enzyme integrated into the mitochondrial membrane, which is essential for regulating the ATP synthesis of the mitochondrial tricarboxylic acid cycle (Bezawork-Geleta et al., 2017). Consistent with the upregulation of ATP content by LED-Red, SDH activity in CMs also exhibited upregulation after stimulation (Fig. 1B). In addition, JC-1 staining showed a significant increase in mitochondrial membrane potential (MMP) in CMs stimulated by LED-Red, compared with the group without irradiation treatment (Fig. 1C). Thus, the above results showed that LED-Red elevated SDH enzyme activity in mitochondria of CMs increased MMP, and promoted ATP synthesis.
Figure 1: 630 nm light emitting diodes regulates homeostatic activity within the mitochondria of cardiomyocytes. CMs were assayed for (A) ATP content (B) succinate dehydrogenase (SDH) activity (C) mitochondrial membrane potential after LED-Red irradiation. 20× lens. n = 3. The data represent the mean ± SEM. **p < 0.01, ***p < 0.001 vs. Non-LED.
Visualization of the regulation of enzymes related to glycolipid metabolism using 630 nm light emitting diodes
Mammalian CMs synthesize ATP in the mitochondria, and aerobic respiration is predicated on the oxidative phosphorylation of fatty acids and glycolysis. The above-mentioned experiments revealed that LED-Red promotes ATP synthesis in CMs. Therefore, we next explored whether ATP production correlates with the uptake and consumption of energy substrates by CMs. In the subsequent experiments, we examined the expression of enzymes related to glycolipid metabolism in CMs. qRT-PCR results showed that lipid metabolism genes CD36 and PDK4 expression were significantly upregulated after LED-Red irradiation, compared with the Non-LED group. Among the glucose metabolism genes, CPT1A expression was up-regulated. In addition, the expression of PGC1-α, which regulates mitochondrial biosynthesis, and energy metabolism, also increased due to LED-Red stimulation (Fig. 2A). We examined the expression of proteins expressed by the above-mentioned genes. Interestingly, LED-Red promoted only CD36 and PDK4 protein expression and PGC1-α expression, although the increase was not statistically significant (Fig. 2B). These results suggest that LED-Red stimulation increased the expression of some glycolipid metabolizing RNAs and proteins in CMs and accelerated the transport of metabolic substrates in CMs, which is important for ATP production.
Figure 2: Visualization of the regulation of enzymes related to glycolipid metabolism by 630 nm light emitting diodes. CMs were irradiated with LED-Red and the expression levels of Platelet glycoprotein 4 (CD36), Recombinant Carnitine Palmitoyltransferase 1A (CPT1A), Solute carrier family 2 facilitated glucose transporter member 4 (Glut4), pyruvate dehydrogenase kinase isozyme 4 (PDK4) and Peroxisome proliferator-activated receptor gamma coactivator 1-alp (PGC-1α) were detected by (A) quantitative real-time-polymerase chain reaction (qRT-PCR) and (B) Western blotting. n = 3. The data represent the mean ± SEM. *p < 0.05, **p < 0.01, ***p < 0.001 vs. Non-LED.
Slight upregulation of reactive oxygen species in mitochondria of cardiomyocytes by 630 nm light emitting diodes
PBM alters the process of mitochondrial oxidative stress (Li et al., 2020; Feng et al., 2020). However, no study has verified the regulation of this process using LED-Red in CMs. A DCFH-DA probe showed that LED-Red increased ROS levels in CMs (Fig. 3A). An abnormal increase in ROS is often considered a marker of myocardial injury, although ROS can act as a signaling molecule for intercellular communication. Therefore, we examined the apoptosis of CMs after LED-Red stimulation. The results showed no significant difference in the number of TUNEL-positive CMs in the LED-Red group compared with the Non-LED group (Fig. 3B), suggesting that the slight rise in ROS induced by LED-Red may only function as a signaling molecule, acting as an intercellular communication transmitter, without causing damage to CMs.
Figure 3: 630 nm light emitting diodes slightly upregulates reactive oxygen species in mitochondria of cardiomyocytes. CMs were irradiated with LED-Red and assayed for (A) Reactive oxygen species (ROS) content (B) Terminal deoxynucleotidyl transferase-mediated dUTP nick-end labeling (TUNEL) staining to detect apoptosis. Red represents apoptotic cells, blue represents nuclei. 20x lens. n = 3. The data represent the mean ± SEM. **p < 0.01 vs. Non-LED.
630 nm light emitting diodes induces mitochondrial division in cardiomyocytes
Mitochondria are dynamic organelles that control the number and size of mitochondria through constant division and fusion (Chan, 2020). This has an important role in maintaining cellular activities. LED-Red also shows some involvement in this dynamic process of mitochondria. MitoTracker analysis of living cells showed that LED-Red stimulation-induced mitochondrial division. In addition, LED-Red also affected the distribution of mitochondria, shifting from the normal perinuclear distribution to diffusion into the cytoplasm (Fig. 4A). The dynamic balance of mitochondrial fusion is regulated by DRP1, which is considered the major pre-mitochondrial fission protein. Ser-616 phosphorylation of DRP1 is necessary to activate mitochondrial fission. Western blotting results showed that LED-Red stimulation promoted the phosphorylation of DRP1 compared to those in the unirradiated group (Fig. 4B). In conclusion, LED-Red was involved in mitochondrial kinetic processes, promoting DRP1 phosphorylation and accelerating mitochondrial division. It also changed the distribution of mitochondria in CMs, which facilitated the energy production and transport of CMs.
Figure 4: 630 nm light emitting diodes induces mitochondrial division in cardiomyocytes. Cardiomyocytes were irradiated with LED-Red. (A) MitoTracker staining of mitochondrial morphology in live cells to observe the fusion of divided mitochondria. Green represents mitochondria, blue represents nuclei. n = 3. 120x lens. (B) The expression levels of phosphorylated-Dynamin-related protein 1 (p-DRP1), were detected by Western blotting. n = 3. The data represent the mean ± SEM. *p < 0.05 vs. Non-LED.
630 nm light emitting diodes promotes mitochondrial transcription factor A expression in mitochondria of cardiomyocytes
Mitochondria have a separate genome, and the integrity of the mitochondrial genome is critical for multicellular organisms. TFAM was originally cloned as a transcription factor and is essential for the maintenance of mitochondrial DNA (Zhang et al., 2018). In recent years, TFAM has been found to play a key role in several aspects of mitochondrial DNA transcription, replication, linkage formation, damage perception, and DNA repair (Kang and Hamasaki, 2005). Overexpression of TFAM in mice increased the amount of mitochondrial DNA and significantly improved cardiac dysfunction caused by myocardial infarction (Kang et al., 2007). Maintenance of mitochondrial DNA integrity is important for maintaining normal cellular function under both physiological and pathological conditions. Analysis of frontal TFAM in mitochondria of CMs after LED-Red treatment revealed that LED-Red stimulation caused a significant increase in TFAM expression in mitochondria (Fig. 5A). However, the protein level of TFAM was not altered (Fig. 5B).
Figure 5: 630 nm light emitting diodes promotes mitochondrial transcription factor A expression in mitochondria of cardiomyocytes. CMs were irradiated with LED-Red and the expression levels of Transcription factor A mitochondrial (TFAM) were detected by (A) quantitative real-time-polymerase chain reaction (qRT-PCR) and (B) Western blotting. n = 3. The data represent the mean ± SEM. ***p < 0.001 vs. Non-LED.
PBM is a non-genetic, non-invasive medical technology, and there is increasing evidence of the modulatory effects of PBM on organismal activity (Burton et al., 2015; Hu et al., 2007; Shen et al., 2021). Phototherapy is therapeutically specific, with different wavelengths of light sources exerting different physiological effects. Among these, 630 nm LEDs are mainly used for tissue damage repair, anti-inflammatory response, and cell proliferation and differentiation (Oron et al., 2001; Chaves et al., 2014; Hawkins et al., 2005) because their photons are more effective in penetrating tissues and can more likely accumulate in diseased tissue sites (Xuan et al., 2014). LED-Red has also shown superior performance in regulating myocardial function. An earlier study found that LED-Red could improve contractile function and delay the progression in mice with heart failure (Capalonga et al., 2016). The repair of this pathological process seems to be potentially linked to the mitochondria within the myocardium. Studies have shown that damage to the mitochondrial electron transport chain, generation of oxidative stress, reduction of mitochondrial functional regulatory proteins, and even alterations in mitochondrial transcriptional regulators occur after ischemic cardiomyopathy (Lin and Beal, 2006). This also suggests that improving mitochondrial function could be a potential target for the treatment of ischemic cardiomyopathy. However, the regulation of mitochondrial biophysiological activities within CMs by LED-Red has not been comprehensively investigated until now. Therefore, in this study, we analyzed various regulatory aspects of LED-Red on mitochondria in mammalian CMs and found that LED-Red contributes significantly to mitochondrial function.
The heart requires uninterrupted excitation-coupled contractions and, therefore, also results in permanently high energy demands. However, the heart has a limited capacity for energy storage, and therefore, CMs contain a large number of mitochondria, which account for about 30% of the CM volume and provide about 95% of the ATP for the heartbeat (Sack et al., 2017). Mitochondria are not only the center of cellular metabolism and energy production but also the metabolic center of the tricarboxylic acid cycle and fatty acid oxidative phosphorylation. Mitochondria also serve the role of maintenance of specific cellular processes, including ion transport, sarcoplasmic function, and intracellular Ca2+ homeostasis. Therefore, maintaining and better regulating mitochondrial energy production in CMs and ensuring the good mitochondrial function is important for the physiological activity of the heart. In this study, we found that LED-Red promoted RNA expression of the glycolipid metabolism genes CD36, PDK4, CPT1A, and Glut4 in CMs. However, LED-Red stimulation only altered CD36 and PDK4 protein levels and did not affect the protein expression of other genes. This interesting phenomenon deserves to be explored in-depth in the future.
PGC-1α was first identified in brown adipose tissue, and it interacts with PPARγ to participate in the regulation of mitochondrial metabolism and biogenesis (Puigserver and Spiegelman, 2003). PGC-1α can be induced by physiological stimuli that increase ATP demand and stimulate mitochondrial oxidation, including cold exposure, fasting, and exercise (Goto et al., 2000). Activation of the PGC-1α cascade regulates the increase in cardiac mitochondrial oxidative capacity (Goto et al., 2000; Haemmerle et al., 2011). In cultured CMs, PGC-1α increases the number of mitochondria, upregulates the expression of mitochondrial enzymes, and increases the rate of Fatty acids oxidation and coupled respiration (Lehman et al., 2000). Similarly, in our study LED-Red had a pro-expression effect on PGC1-α in mitochondria of CMs. Mitochondrial ROS have a twofold role in cells; on the one hand, they act as intracellular signaling molecules to ensure normal intercellular signaling, and on the other hand, ROS overload generated by oxidative stress injury significantly impairs myocardial structural function (Tsutsui et al., 2011; Chistiakov et al., 2018; Fukai and Ushio-Fukai, 2020). Although LED-Red can cause mitochondrial ROS production, the amount produced is not sufficient to contribute to myocardial injury. It has also been confirmed that specific deletion of the mitochondrial nuclear transcription factor TFAM in CMs can cause CM cycle arrest and inhibit embryonic heart growth and development, indicating that TFAM is important for CM mitochondria and even cardiomyocyte vital activity (Zhang et al., 2018). In this study, LED-Red irradiation clearly promoted the transcription of TFAM, resulting in the upregulation of TFAM RNA levels, but LED-Red did not alter the translational regulation of TFAM. This process still needs to be further explored, and a negative feedback regulation in CMs may affect the protein translation of TFAM.
In conclusion, we investigated the effects of LED-Red on mitochondrial biological functions in CMs. We found that LED-Red could promote ATP synthesis, mitochondrial division, and mitochondrial TFAM expression in CMs. This is of great scientific significance for the future investigation of PBM for the treatment of ischemic cardiomyopathy and understanding the molecular mechanism of the treatment. However, this research is still limited, as we did not validate the effect of LED-Red on CM mitochondria under pathological conditions, and elucidating this mechanism is important for future studies on the function of PBM in cardiovascular diseases.
Author Contribution: XLG designed the experiments, analyzed data, and wrote the manuscript. XXW, WWZ, and HJL conducted the main experiments and assisted in the data analysis. FY and WYM helped to edit the manuscript. YL designed the project, managed the experiments, and prepared the manuscript.
Availability of Data and Materials: The datasets used and/or analyzed during the present study are available from the corresponding author on reasonable request.
Ethics Approval: This study was approved by the Medical Ethics Committee of the Second Affiliated Hospital of Harbin Medical University (No. KY2021-363).
Funding Statement: This work was supported by grants from the National Key Research and Development Program of China (2017YFB0403802).
Conflicts of Interest: The authors declare that they have no conflicts of interest to report regarding the present study.
References
- Ambrosio, G., Zweier, JL., Duilio, C., Kuppusamy, P., & Santoro, G. (1993). Evidence that mitochondrial respiration is a source of potentially toxic oxygen free radicals in intact rabbit hearts subjected to ischemia and reflow. The Journal of Biological Chemistry, 268, 18532-18541. [Google Scholar] [CrossRef]
- Barolet, D., Roberge, CJ., Auger, FA., Boucher, A., & Germain, L. (2009). Regulation of skin collagen metabolism using a pulsed 660 nm LED light source: Clinical correlation with a single-blinded study. The Journal of Investigative Dermatology, 129, 2751-2759. [Google Scholar] [CrossRef]
- Bezawork-Geleta, A., Rohlena, J., Dong, LF., Pacak, K., & Neuzil, J. (2017). Mitochondrial complex II: At the crossroads. Trends in Biochemical Sciences, 42, 312-325. [Google Scholar] [CrossRef]
- Burton, RAB., Klimas, A., Ambrosi, CM., Tomek, J., Corbett, A., Entcheva, E., & Bub, G. (2015). Optical control of excitation waves in cardiac tissue. Nature Photonics, 9, 813-816. [Google Scholar] [CrossRef]
- Capalonga, L., Karsten, M., Hentschke, VS., Rossato, DD., Dornelles, MP., Sonza, A., Bagnato, VS., Ferraresi, C., Parizotto, NA., & Lago, PD. (2016). Light-emitting diode therapy (LEDT) improves functional capacity in rats with heart failure. Lasers in Medical Science, 31, 937-944. [Google Scholar] [CrossRef]
- Chan, DC. (2020). Mitochondrial dynamics and its involvement in disease. Annual Review of Pathology, 15, 235-259. [Google Scholar] [CrossRef]
- Chaves, MEDA., Araújo, ARD., Piancastelli, ACC., & Pinotti, M. (2014). Effects of low-power light therapy on wound healing: LASER × LED. Anais Brasileiros de Dermatologia, 89, 616-623. [Google Scholar] [CrossRef]
- Chistiakov, DA., Shkurat, TP., Melnichenko, AA., Grechko, AV., & Orekhov, AN. (2018). The role of mitochondrial dysfunction in cardiovascular disease: A brief review. Annals of Medicine, 50, 121-127. [Google Scholar] [CrossRef]
- Feng, J., Li, XY., Zhu, SY., Xie, YM., Du, J., Ge, HB., Bai, YX., Liu, Y., & Guo, LJ. (2020). Photobiomodulation with 808-nm diode laser enhances gingival wound healing by promoting migration of human gingival mesenchymal stem cells via ROS/JNK/NF-κB/MMP-1 pathway. Lasers in Medical Science, 35, 1831-1839. [Google Scholar] [CrossRef]
- Fukai, T., & Ushio-Fukai, M. (2020). Cross-Talk between NADPH oxidase and mitochondria: Role in ROS signaling and angiogenesis. Cells, 9, 1849. [Google Scholar] [CrossRef]
- Goto, M., Terada, S., Kato, M., Katoh, M., Yokozeki, T., Tabata, I., & Shimokawa, T. (2000). cDNA Cloning and mRNA analysis of PGC-1 in epitrochlearis muscle in swimming-exercised rats. Biochemical and Biophysical Research Communications, 274, 350-354. [Google Scholar] [CrossRef]
- Haemmerle, G., Moustafa, T., Woelkart, G., Büttner, S., & Schmidt, A. (2011). ATGL-mediated fat catabolism regulates cardiac mitochondrial function via PPAR-α and PGC-1. Nature Medicine, 17, 1076-1085. [Google Scholar] [CrossRef]
- Hawkins, D., Houreld, N., & Abrahamse, H. (2005). Low level laser therapy (LLLT) as an effective therapeutic modality for delayed wound healing. Annals of the New York Academy of Sciences, 1056, 486-493. [Google Scholar] [CrossRef]
- Hentschke, SV., Jaenisch, RB., Schmeing, LA., Cavinato, PR., Xavier, LL., & Lago, PD. (2013). Low-level laser therapy improves the inflammatory profile of rats with heart failure. Lasers in Medical Science, 28, 1007-1016. [Google Scholar] [CrossRef]
- Hu, WP., Wang, JJ., Yu, CL., Lan, CCE., Chen, GS., & Yu, HS. (2007). Helium-neon laser irradiation stimulates cell proliferation through photostimulatory effects in mitochondria. The Journal of Investigative Dermatology, 127, 2048-2057. [Google Scholar] [CrossRef]
- Huss, JM., & Kelly, DP. (2005). Mitochondrial energy metabolism in heart failure: A question of balance. The Journal of Clinical Investigation, 115, 547-555. [Google Scholar] [CrossRef]
- Jenkins, MW., Duke, AR., Gu, S., Chiel, HJ., Fujioka, H., Watanabe, M., Jansen, ED., & Rollins, AM. (2010). Optical pacing of the embryonic heart. Nature Photonics, 4, 623-626. [Google Scholar] [CrossRef]
- Kang, DC., & Hamasaki, N. (2005). Mitochondrial transcription factor a in the maintenance of mitochondrial DNA: Overview of its multiple roles. Annals of the New York Academy of Sciences, 1042, 101-108. [Google Scholar] [CrossRef]
- Kang, DC., Kim, SH., & Hamasaki, N. (2007). Mitochondrial transcription factor A (TFAM): Roles in maintenance of mtDNA and cellular functions. Mitochondrion, 7, 39-44. [Google Scholar] [CrossRef]
- Kuboi, T., Kusaka, T., Okada, H., Arioka, M., & Nii, K. (2019). Green light-emitting diode phototherapy for neonatal hyperbilirubinemia: Randomized controlled trial. Pediatrics International: Official Journal of the Japan Pediatric Society, 61, 465-470. [Google Scholar] [CrossRef]
- Lehman, JJ., Barger, PM., Kovacs, A., Saffitz, JE., Medeiros, DM., & Kelly, DP. (2000). Peroxisome proliferator-activated receptor gamma coactivator-1 promotes cardiac mitochondrial biogenesis. The Journal of Clinical Investigation, 106, 847-856. [Google Scholar] [CrossRef]
- Li, H., Sun, T., Liu, C., Cao, Y., & Liu, X. (2020). Photobiomodulation (450 nm) alters the infection of periodontitis bacteria via the ROS/MAPK/mTOR signaling pathway. Free Radical Biology & Medicine, 152, 838-853. [Google Scholar] [CrossRef]
- Lin, MT., & Beal, MF. (2006). Mitochondrial dysfunction and oxidative stress in neurodegenerative diseases. Nature, 443, 787-795. [Google Scholar] [CrossRef]
- Murphy, E., & Steenbergen, C. (2008). Mechanisms underlying acute protection from cardiac ischemia-reperfusion injury. Physiological Reviews, 88, 581-609. [Google Scholar] [CrossRef]
- Mussttaf, RA., Jenkins, DFL., & Jha, AN. (2019). Assessing the impact of low level laser therapy (LLLT) on biological systems: A review. International Journal of Radiation Biology, 95, 120-143. [Google Scholar] [CrossRef]
- Oron, U., Yaakobi, T., Oron, A., Mordechovitz, D., & Shofti, R. (2001). Low-energy laser irradiation reduces formation of scar tissue after myocardial infarction in rats and dogs. Circulation, 103, 296-301. [Google Scholar] [CrossRef]
- Puigserver, P., & Spiegelman, BM. (2003). Peroxisome proliferator-activated receptor-gamma coactivator 1 alpha (PGC-1 alpha): Transcriptional coactivator and metabolic regulator. Endocrine Reviews, 24, 78-90. [Google Scholar] [CrossRef]
- Sack, MN., Fyhrquist, FY., Saijonmaa, OJ., Fuster, V., & Kovacic, JC. (2017). Basic biology of oxidative stress and the cardiovascular system: Part 1 of a 3-part series. Journal of the American College of Cardiology, 70, 196-211. [Google Scholar] [CrossRef]
- Shen, Q., Liu, L., Gu, XT., & Xing, D. (2021). Photobiomodulation suppresses JNK3 by activation of ERK/MKP7 to attenuate AMPA receptor endocytosis in Alzheimer’s disease. Aging Cell, 20, e13289. [Google Scholar] [CrossRef]
- Teixeira, AF., Alves, JR., Fonseca, ADSD., & Mencalha, AL. (2018). Low power blue LED exposure increases effects of doxorubicin on MDA-MB-231 breast cancer cells. Photodiagnosis and Photodynamic Therapy, 24, 250-255. [Google Scholar] [CrossRef]
- Tsutsui, H., Kinugawa, S., & Matsushima, S. (2011). Oxidative stress and heart failure. American Journal of Physiology-Heart and Circulatory Physiology, 301, H2181-H2190. [Google Scholar] [CrossRef]
- Vladimirov, YA., Osipov, AN., & Klebanov, GI. (2004). Photobiological principles of therapeutic applications of laser radiation. Biochemistry. Biokhimiia, 69, 81-90. [Google Scholar] [CrossRef]
- Walters, AM., Porter, GA., & Brookes, PS. (2012). Mitochondria as a drug target in ischemic heart disease and cardiomyopathy. Circulation Research, 111, 1222-1236. [Google Scholar] [CrossRef]
- Wang, MT., Xu, ZL., Liu, Q., Sun, WJ., & Jiang, BC. (2019). Nongenetic optical modulation of neural stem cell proliferation and neuronal/glial differentiation. Biomaterials, 225, 119539. [Google Scholar] [CrossRef]
- Willis, GL., Boda, J., & Freelance, CB. (2018). Polychromatic light exposure as a therapeutic in the treatment and management of Parkinson’s disease: A controlled exploratory trial. Frontiers in Neurology, 9, 741. [Google Scholar] [CrossRef]
- Xuan, WJ., Vatansever, F., Huang, LY., & Hamblin, MR. (2014). Transcranial low-level laser therapy enhances learning, memory, and neuroprogenitor cells after traumatic brain injury in mice. Journal of Biomedical Optics, 19, 108003. [Google Scholar] [CrossRef]
- Zhang, DH., Li, YF., Waldron, DH., Bezzerides, V., & Guatimosim, S. (2018). Mitochondrial cardiomyopathy caused by elevated reactive oxygen species and impaired cardiomyocyte proliferation. Circulation Research, 122, 74-87. [Google Scholar] [CrossRef]
Cite This Article
GAO, X., WANG, X., ZHANG, W., LI, H., YANG, F. et al. (2022). Mitochondria are an important target of photobiomodulation in cardiomyocytes. BIOCELL, 46(12), 2637–2644. https://doi.org/10.32604/biocell.2022.021033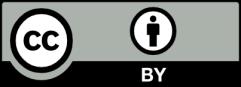