Open Access
ARTICLE
Nitric oxide alleviates cadmium-impeded growth by limiting ROS accumulation in pea seedlings
1 Department of Life Sciences, Central University of Jharkhand, Ranchi, 835205, India
2 Department of Biotechnology, Chaudhary Bansi Lal University, Bhiwani, 127021, India
3 Horticulture Research Institute (HRI), Agricultural Research Center (ARC), Giza, 12619, Egypt
4 School of Environment and Sustainable Development, Central University of Gujrat, Gandhinagar, 382030, India
5 School of Applied Sciences, Centurion University of Technology and Management, Bhubaneswar, 752050, India
6 Department of Agronomy, Faculty of Agriculture, Sher-e-Bangla Agricultural University, Dhaka, 1207, Bangladesh
* Corresponding Authors: EKHLAQUE A. KHAN. Email: ; MIRZA HASANUZZAMAN. Email:
(This article belongs to the Special Issue: Physiology and Molecular Biology of Plant Stress Tolerance)
BIOCELL 2022, 46(12), 2583-2593. https://doi.org/10.32604/biocell.2022.021732
Received 31 January 2022; Accepted 18 April 2022; Issue published 10 August 2022
Abstract
Cadmium (Cd) causes oxidative stress, which leads to the oxidation of various biomolecules by the production of reactive oxygen species (ROS) to facilitate programmed cell death (PCD). The antioxidant defense system fails to detoxify ROS when it is produced in excess. Nitric oxide (NO), a gaseous free radical and a phytohormone, regulates various physiological processes of plants. Therefore, this work was undertaken to study the effects of the application of exogenous sodium nitroprusside (SNP, a NO donor) on growth parameters, oxidative stress, accumulation of secondary metabolites, and activities of antioxidant enzymes under Cd stress. Mild (50 µM) and severe (200 µM) Cd stress were applied to hydroponically grown pea (Pisum sativum L.) plants with or without 50 µM SNP. Severe Cd stress had a substantial impact on the plants. The effectiveness of NO in reducing Cd-induced negative effects on plant height, fresh weight, dry weight, protein content, nitrite content, nitrate reductase (NR) activity, catalase activity, and peroxidase activity were investigated. Seedling development, protein content, nitrite content, nitrate reductase (NR) activity, antioxidant defense systems disruption, overproduction of reactive oxygen species, and oxidative damage were observed. The antioxidant defense system (catalase and peroxidase activities) was activated by NO, which resulted in lower lipid peroxidation and lower hydrogen peroxide (H2O2) levels in Cd-exposed plants. SNP treatment boosted endogenous NO levels and NR activity in Cd-stressed plants while also enhanced proline levels to preserve osmotic equilibrium. The presence of total phenols and flavonoids increased after SNP treatment, indicating that SNP enhanced stress recovery and boosted plant development in Cd-stressed plants.
Keywords
The toxic heavy metal like cadmium (Cd) reaches the soil mainly as Cd2+ via various natural and anthropogenic activities. Soil pollution occurs because Cd becomes a hazardous threat by inhibiting plant development by damaging various metabolic activities, reducing intake of water and nutrient, and modifying organ development. Furthermore, the availability of Cd in the soil causes a reduction in the market price of various edible plants, and as a result, Cd becomes a challenge for living beings (Zhang et al., 2020). Cd simply reaches the root from the soil either through channel or nutrients transporters or via aquaporins, and from there, it is transported to aerial parts of the plants and gets accumulated. Cd has a great affinity to phosphates, porphyrins, purines, pteridines, histidyl, and cysteinyl side chains of proteins; hence, it has the potential to damage nucleic acids, proteins, lipids, and enzymes (Cheng et al., 2016). Cd promotes severe stress by causing increased production of reactive oxygen species (ROS) (Kumar and Khan, 2021), which, if not detoxified, promptly lead to oxidation of proteins, lipids, and DNA, triggering programmed cell death (PCD), and dysregulation of a serious physiological and metabolic process of plant development leading to selective cell death.
Plants usually trigger defense strategies at every stage of their life to protect themselves from toxicity in the soil; they protect themselves from heavy metal contamination by controlling the free radicle ion produced in the cell by eliminating, immobilizing, chelating, attaching to thiol-peptides, and by vacuolar segregation (Zaid and Wani, 2019). However, when ROS is produced in excess, the antioxidant enzymes fail to reduce their level. In heavy metal-stressed plants, attempts have been made to increase the efficacy of components of the antioxidant defense system by supplying various compounds and phytohormones exogenously (Mohamed et al., 2019; Sharma et al., 2012). In the past few years, several results have shown that the reactive nitrogen species (RNS), specifically nitric oxide (NO), interacts with ROS to protect the plant from heavy metals stress, although the mechanism behind this process is still unknown. In fact, while the ROS produce oxidative stress and NO may produce nitrosative stress, both act as signaling molecules depending on their amounts in the cells in response to Cd stimuli (Liu et al., 2019).
Plants can change their NO metabolism when exposed to Cd toxicity; nevertheless, conflicting data are available showing the effect of Cd2+ on endogenously produced NO (Genchi et al., 2020). In fact, as reported in numerous plant species, Cd can either enhance or inhibit NO levels (Corpas and Barroso, 2014). Furthermore, exogenous NO donor treatments were demonstrated to defend plant tissues from oxidative stress that occurred due to Cd2+ through increasing ROS scavenging (Kopyra et al., 2006; Noriega et al., 2007).
NO, being a plant hormone has lately been recognized as a possible signaling molecule (Khan et al., 2017; Khan et al., 2022a; Khan et al., 2022b). It is a universal signal molecule that controls various activities of animals and plants at the physiological and molecular level and provides tolerance to plants from heavy metal stress by increasing the antioxidant defense system (Anjum et al., 2016; Nabi et al., 2019). Several findings have stated the involvement of NO in the regulation of plant Cd response (Kopyra et al., 2006). Despite the fact that NO is important for regulating the biological processes produced by plants against metalloid stress, the exact pathway of NO signaling is unknown to date. Sodium nitroprusside (SNP) is one among other extensively used NO donor substances for supplying NO to plants, regardless of whether they are growing in natural soil, synthetic or prepared media, or hydroponic (Soares et al., 2021). Its significance in physiological processes, however, is determined at the cellular level. Indeed, at very low levels, it serves as a signaling molecule, but at high levels, it acts as a stress-causing signaling molecule (Fancy et al., 2017).
The peroxynitrite (ONOO-) is formed when NO reacts with superoxide anion (O2•−), which is considered one of the simplest and fastest reactions in living systems (Arasimowicz-Jelonek and Floryszak-Wieczorek, 2011; Corpas and Barroso, 2014). The peroxynitrite is a highly unstable anion and a member of the RNS family. Although it is generally established that ONOO- is not hazardous to plant cells at low concentrations, a rise in its cellular levels causes stress, as reported in Arabidopsis (Corpas and Barroso, 2014). NO has the ability to modify a variety of proteins, either directly through S-nitrosylation, nitration, and nitrosylation, or secondarily through monitoring the stress proteins genes transcription, which explains its role in plant physiological/metabolic processes (Fancy et al., 2017).
Pea (Pisum sativum L.) is the main nutritious legume crop, considered highly sensitive to Cd toxicity compared to cereals and grasses. Based on earlier studies, we hypothesized that NO plays an important role in providing tolerance to pea plants from Cd stress. Therefore, we subjected pea seedlings to low and high Cd concentrations to understand the response and physiology of pea plants growing under Cd stress. Our primary objective was to use this understanding to produce Cd tolerance in other plant varieties that currently suffer from severe Cd damage and crop losses. We investigated the effect of Cd stress on pea seedlings and the mechanism by which exogenously supplied NO ameliorates Cd toxicity in examined plants.
Plant material and stress conditions
The sodium hypochlorite (NaOCl) (v/v) solution of 0.5% was used for surface sterilization of pea (Pisum sativum L.) seeds which were then rinsed thrice with deionized water. The sterilized seeds were placed at random on two-layered filter paper moistened with distilled water and kept at 28 ± 2°C for 3 days for better germination. After germination, seedlings were moved to pots having Hoagland solution. The treatment solutions included two concentrations of CdCl2 (50 µM and 200 µM) with or without SNP (50 µM) added to the Hoagland solution. Growth media were continuously aerated and renewed on each third day. A growth chamber (ACMAS Technology Pvt., Ltd., India) was used for growing seedlings. The growth chamber was maintained with 12 h/12 h light/dark cycles, at 25/20°C, under a white fluorescent light intensity of 350 µmol photons m–2 s–1, and 70% relative humidity. After three weeks of growing, plants were harvested, washed completely, and plant parts were used for further estimations. All the experiment was carried out thrice.
Evaluation of growth and biomass
The shoots and roots length of pea seedlings were measured by a measuring scale. The length between root–shoot knot and the tip apex of the root was considered as root length, and shoot length was the difference between the base of the culm to the tip apex of the plants. The measurements were presented in centimeters. The seedlings were cleaned with double distilled water and blotted between filter papers before measuring their fresh weight (FW). A weighing balance was used to determine the fresh weight. Dry matter was obtained by drying the root and shoot samples at 70°C for 72 h in an oven.
Quantification of Nitric Oxide
By quantifying nitrite, NO was indirectly measured. Evans and Nason’s method was used to calculate the nitrite content (Evans and Nason, 1953). Briefly, plant root and shoot were homogenized in acetic acid buffer (50 mM, pH 3.6) in a chilled mortar pestle, followed by centrifugation (10,000 g, 15 min) and removal of the residue. Greiss reagent was mixed with the obtained supernatant. This reagent contained 1.0% sulfanilamide in 1N-HCl, and 0.025% N-(1-Napthyl)-ethylene diammonium dichloride (NEDD) and optical density (OD) was recorded at 540 nm.
Determination of Nitrate Reductase (NR) activity
To determine NR activity, the method by Hageman and his colleagues was followed (Hageman et al., 1980). Fresh root and shoot tissues (0.5 g) were crushed in potassium phosphate buffer (0.05 M, pH 7.8) and 0.4 M KNO3 solution. The obtained homogenate was incubated at 35°C for 75 min in the dark. After incubation, tubes were subjected to a hot treatment for 5 min to terminate the activity of the enzyme. A 200 µL aliquot of the resulted product was collected and mixed with 2 mL Greiss reagent (1.0% sulfanilamide in 1N HCl and 0.025% NEDD). Diazotization resulted in a pink color after 30 min of incubation. Finally, using distilled water, the volume was increased to 6 mL, and the OD was measured at 540 nm. The standard curve was made from sodium nitrite. The activity of the enzyme was measured in μmol NO2 min−1 g−1 FW.
Determination of Hydrogen Peroxide (H2O2)
The quantity of H2O2 was calculated using Cheeseman’s approach (Cheeseman, 2006). In a prechilled pestle and mortar, 0.5 g of root and shoot was crushed in 5 mL trichloroacetic acid (TCA) buffer (0.1%) followed by centrifugation (12,000 g, 15 min, 4°C). The obtained supernatant was used to calculate the amount of H2O2. One milliliter of supernatant was added to phosphate buffer (10 mM, pH 7.0), and KI (1 M), and the absorbance was measured at 390 nm. The concentration of H2O2 was measured in µmol H2O2 g−1 FW.
Estimation of Lipid Peroxidation
The method, using 2-thiobarbituric acid (TBA), developed by Heath and Packer (1968), was used for estimating the malondialdehyde (MDA) level, which is produced upon pre-oxidation of polyunsaturated fatty acid. Root and shoot were homogenized in 0.1% TCA buffer followed by centrifugation for 10 min. The supernatant obtained after centrifugation was incubated at 95°C with 4 mL of 0.5% TBA in 20% TCA for 30 min. To stop the process, the mixture was immediately placed on ice and centrifuged (8,000 g for 10 min). The MDA level was obtained by calculating the differences in absorbance at 600 nm and 532 nm using 155 mM−1 cm−1 as an extinction coefficient. The concentration of MDA was expressed as nmol MDA g−1 FW.
Root and shoot tissues (0.5 g) were homogenized in 10 mL of extraction buffer (3% sulfosalicylic acid). The resultant filtrate was used to calculate proline using the technique of Bates et al. (1973). In a test tube, 2 mL filtrate was added to 2 mL of acid ninhydrin followed by 2 mL of glacial acetic acid and incubated at 100°C for 1 h. The reaction was immediately transferred to the ice to stop the reaction. Toluene was used to extract the reaction mixture. A test tube stirrer was used to thoroughly mix the test tubes for 15–20 s. The toluene-containing chromophore was removed from the upper layer, and the OD was measured spectrophotometrically at 520 nm, considering toluene as a blank. L-proline (0.02 to 0.1 M) standard curve was used to measure the concentration of proline and expressed as mg proline g−1 FW.
Bradford’s test was performed to estimate the total amount of protein in root and shoot samples (Prasertsongskun et al., 2002). In test tubes, 100 μL plant extract was mixed with 5 mL of the diluted dye. The reaction mixture was incubated at room temperature for 5 min, and the color change was recorded spectrophotometrically at 595 nm. The standard curve was prepared using bovine serum albumin to calculate the protein concentrations.
Estimation of antioxidant enzyme activities
The plant tissues were homogenized in phosphate buffer (100 mM, pH 7.5) with 1 mM EDTA and 5% insoluble PVP in a 3:1 ratio, followed by centrifugation at 10,000 g for 30 min. Protein and enzyme activities were measured using the extracts. The activity of catalase (EC: 1.11.1.6) was estimated by measuring the decline in absorbance that occurred due to the disappearance of H2O2 at 240 nm (Aebi, 1984). In a cuvette, 100 μL of enzyme extract was quickly added to 3.0 mL H2O2-phosphate buffer prepared by mixing 0.067 mM phosphate buffer (pH 7) with 2 mM H2O2. The change in OD was measured in a spectrophotometer at 240 nm at intervals of 30 s up to 3 min. As a control, an enzyme solution containing H2O2-free phosphate buffer was used. The decreasing absorbance was measured at 240 nm for 3 min, and the enzyme unit mg−1 protein was calculated. One unit of the enzyme was defined as the quantity of enzyme essential to degrade 1 μM H2O2 min−1.
The activity of peroxidase (POD, EC: 1.11.1.7) was measured by using the procedure of Li et al. (2014). POD uses pyrogallol as a hydrogen donor and converts H2O2 to H2O and O2. When pyrogallol is oxidized, it generates purpurogalli, a colorful product that may be detected spectrophotometrically at 430 nm. A hundred microliters of enzyme extract was mixed thoroughly with 3.0 mL of 50 μM pyrogallol solution. Five hundred microliters of 1% H2O2 was mixed properly in the test cuvette. The change in OD at 430 nm was recorded for a gap of every 30 s till 3 min and presented as enzyme units mg−1 protein. One enzyme unit was defined as the quantity of enzyme needed to produce one µmole purpurogalli every min.
Determination of phenolics and flavonoids
An assay modified by Shetty and co-workers was used to measure total soluble phenolics (Shetty et al., 1995). The leaf tissue weighing 0.5 g was immersed in 95% ethanol and frozen for 48 h. The leaf sample was crushed and followed by centrifugation at 12,000 g for 10 min. The supernatant (0.5 mL) was diluted with 0.5 mL distilled water and then placed into a test tube having 1 mL 95% ethanol and 5 mL distilled water. Each sample was mixed thoroughly with 500 µL of 50% (v/v) Folin and Ciocalteu’s reagent. The sample mixture was incubated for 5 min, then 1 mL of 5% Na2CO3 was added to it and left for 60 min. As a blank, 0.5 mL of double distilled water was taken, and the absorbance was noted at 725 nm. The standard curves were prepared by taking various concentrations of gallic acid in 95% ethanol. The obtained absorbances were transformed to total phenol and represented in mg equal to gallic acid per g of FW.
The total flavonoid content was determined using the method of Jia and coworkers (Jia et al., 1999). At room temperature, the leaf sample was homogenized with ethanol. To each extract, methanol was added to 300 µL to make the final volume up to 2 mL followed by the addition of 0.1 mL of AlCl3 (10%) and 0.1 mL of 1 M sodium acetate (CH3COONa). The final volume was then adjusted to 5 mL using double distilled water. The sample was then incubated for 30 min and absorbance was measured at 415 nm using a spectrophotometer (Perkin Elmer UV-VIS 35). The standard curve was made using quercetin. The data were given in µg of quercetin per g of fresh weight.
One-way ANOVA was performed by Graph Pad PRISM version 5.01. The error bars on the figures represent the standard deviation (SE). Using Tukey’s honest significant difference (HSD) test at p ≤ 0.05, different letters show significant differences while the same letters represent no significant differences.
Cd concentration of 50 µM decreased the shoot length by 37%, root length by 36%, shoot fresh weight by 21%, shoot dry weight by 26%, root fresh weight by 37%, and root dry weight by 21% in comparison to those of the control (Figs. 1A, 1C and 1E). Cd concentration of 200 µM reduced the shoot length by 62%, root length by 47%, shoot fresh weight by 43%, shoot dry weight by 61%, root fresh weight by 60%, and root dry weight by 54% in comparison to those of the control. The use of 50 µM SNP with 50 and 200 µM Cd increased the shoot length by 46% and 77% and root length by 63% and 42%, respectively, compared to the treatment with 50 and 200 µM Cd alone (Figs. 1A and B). The shoot fresh weight and dry weight were also elevated by the supplementation of 50 µM SNP with 200 µM Cd, and the maximum increase in shoot fresh weight and dry weight was 42% and 65%, corresponding to 200 µM Cd treatment (Figs. 1C–1F). The root fresh weight and dry weight also increased when 50 µM SNP was used along with 200 µM Cd, and an increase in root fresh weight by 56% and root dry weight by 64% was observed compared to those after 200 µM Cd treatment (Figs. 1C–1F).
Figure 1: Effect of Cd, SNP, and their combinations on length (A,B), fresh weight (C,D), and dry weight (E,F) of shoot and root of pea seedlings. Different letters represent significant differences, and the same letters represent no significant difference by applying Tukey’s honest significant difference (HSD) test at p ≤ 0.05.
Cd concentrations of 50 and 200 µM reduced nitrite content by 53% and 60% in shoot and 50% and 68% in root compared to control. Supplementation of NO with Cd improved the nitrite content and NR activity in both root and shoot (Figs. 2A–2D). SNP concentration of 50 µM with 50 µM Cd enhanced the nitrite content by 23% and 27% in shoot and root, respectively, whereas 200 µM Cd enhanced the nitrite content by 24% and 59% in shoot and root, respectively (Figs. 2A and 2B). Cd treatment reduced the NR activity in shoot and root by 47% and 34%, respectively, at 50 µM concentration and by 100% and 61%, respectively, at 200 µM concentration compared to control. The use of NO to 50 µM Cd treated plants enhanced the NR content by 27% and 26% in shoot and root, respectively, whereas NO supplementation to 200 µM Cd stressed plants enhanced the NR content by 30% and 43% in shoot and root, respectively (Figs. 2C and 2D).
Figure 2: Effect of Cd, SNP and their combinations on nitrite content (A,B) and NR activity (C,D) in shoot and root of pea seedlings. Different letters represent significant differences, and the same letters represent no significant difference by applying Tukey’s honest significant difference (HSD) test at p ≤ 0.05.
In shoot and root, Cd treatment of 50 µM led to a 31% and 44% increase in H2O2 and 16% and 44% increase in MDA content, respectively (Figs. 3A–3D). Cd concentration of 200 µM caused 65% and 77% increase in H2O2 content and 44% and 120% increase in MDA content in the shoot and root, respectively, compared to those in control plants. The addition of 50 µM SNP to 50 µM Cd reduced H2O2 accumulation by 24% in the shoot and 24% in the root, whereas the addition of 50 µM SNP to 200 µM Cd caused 28% reduction in H2O2 content in the shoot and 25% reduction in the root (Figs. 3A and 3B). MDA content decreased by 22% in shoot and 31% in root when 50 µM SNP was used along with 50 µM Cd compared to 50 µM Cd treatment alone. Similarly, the use of 50 µM SNP along with 200 µM Cd reduced MDA content by 22% in the shoot and 22% in the root (Figs. 3C and 3D).
Figure 3: Effect of Cd, SNP, and their combinations on H2O2 (A,B) and MDA (C,D) content in shoot and root of pea seedlings. Different letters represent significant differences, and the same letters represent no significant difference by applying Tukey’s honest significant difference (HSD) test at p ≤ 0.05.
The amount of proline reduced by 22% in the shoot and 28% in the root, whereas protein content decreased by 25% in the shoot and 39% in the root at 50 µM Cd treatment (Figs. 4A–4D). At 200 µM Cd concentration, proline content reduced by 5% in the shoot and 16% in the root, whereas protein content decreased by 63% in the shoot and 68% in the root, respectively, relative to those in control. Supplementation of 50 µM SNP along with 50 µM Cd led to a 100% and 42% decrease of proline content in shoot and root, respectively, and a 50% and 72% increase in protein content in shoot and root respectively. Treatment with 50 µM SNP along with 200 µM Cd decreased proline content by 73% in shoot and 31% in root and enhanced protein content by 74% in shoot and 100% in root compared to 200 µM Cd treatment alone (Figs. 4A–4D).
Figure 4: Effect of Cd, SNP, and their combination on proline (A,B) and protein (C,D) content in shoot and root of pea seedlings. Different letters represent significant differences, and the same letters represent no significant difference by applying Tukey’s honest significant difference (HSD) test at p ≤ 0.05.
Total phenols and flavonoids content
The contents of total phenols and flavonoids decreased by 41% and 37% in the shoot at 50 µM Cd and by 61% and 59% in the shoot at 200 µM Cd treated plants in comparison to those in control plants (Figs. 5A and 5B). However, 50 µM SNP increased the phenols and flavonoids by 30% and 20% in the shoot, respectively, when supplied along with 50 µM Cd and by 40% and 36% in the shoot, respectively, when supplied with 200 µM Cd (Figs. 5A and 5B).
Figure 5: Effect of Cd, SNP and their combination on phenols (A) and flavonoids (B) content in the shoot of pea seedlings. Different letters represent significant differences, and the same letters represent no significant difference by applying Tukey’s honest significant difference (HSD) test at p ≤ 0.05.
Catalase (CAT) and peroxidase activities
In 50 µM and 200 µM Cd-treated stressed plants, CAT activity was reduced by 48% and 61%, respectively, in shoot and 34% and 62% in root as compared to control (Figs. 6A and 6B). POD activity was reduced by 10% and 19% in shoot and root, respectively, in 50 µM Cd treated plants, but it was decreased by 48% and 36% in shoot and root, respectively, in 200 µM Cd treated plants (Figs. 6C and 6D). NO supplementation along with Cd resulted in a significant enhancement in the activities of CAT and POD both in root and shoot compared to 50 µM and 200 µM Cd treatment (Figs. 6A–6D). Supplementation of 50 µM SNP with 50 µM Cd increased CAT activity by 61% in shoot and 36% in root compared to 50 µM Cd treatment, whereas supplementation with 200 µM Cd led to a 76% increase in shoot and 58% increase in root compared to 200 µM Cd. The POD activity increased by 19% in shoot and 41% in root when 50 µM SNP was supplied with 50 µM Cd and 34% in shoot and 44% in root when 50 µM SNP was supplied along with 200 µM Cd (Figs. 6C and 6D).
Figure 6: Effect of Cd, SNP, and their combination on catalase (A,B) and peroxidase (C,D) activity in shoot and root of pea seedlings. Different letters represent significant differences, and the same letters represent no significant difference by applying Tukey’s honest significant difference (HSD) test at p ≤ 0.05.
The current findings suggest that NO has a role in regulating pea responses to Cd toxicity. The exogenous supply of NO-donor SNP to the Cd-contaminated rice root increases intracellular NO and alleviates the morphological and anatomical damages caused by Cd by restoring the ROS/RNS balance in the cell (Sharma et al., 2020a). Plants absorb Cd easily through roots growing in Cd-rich environments. Only a little amount of Cd is transfered to the shoots from the roots (Moussa and El-Gamal, 2010). As Cd is a non-essential element, its accumulation inhibits the growth and development of the plant (Wang et al., 2021). NO acts as a key signaling molecule in plants, and its importance in alleviating abiotic and biotic stressors has prompted widespread interest (Khan et al., 2017). The endogenous NO level increased in Cd-affected pea plants developing in a stressed environment (Huang et al., 2020). This increase in NO suggests that it acts as a stress signaling molecule (Molassiotis et al., 2010). Treatment of Cd-stressed pea seedlings with SNP boosted endogenous NO levels even further, indicating effective uptake and storage of NO provided by SNP, which is consistent with earlier investigations that have found a similar role of SNP (Li et al., 2014). NO has been identified in recent studies as an important messenger molecule in plants and provides tolerance from various types of heavy metals like arsenic (Singh et al., 2009), Cd (Wang et al., 2013; Wang et al., 2013a), and copper (Zhang et al., 2009). In one previous study, we reported that a specific concentration of NO was needed to alleviate Cd stress in pea seedlings, and a low concentration of NO provides more tolerance against Cd toxicity (Khan et al., 2017; Mohamed et al., 2019). In a previous work, different concentrations of SNP were supplied exogenously to a Cd-treated growth medium to determine the optimal NO level for efficiently reducing Cd toxicity. Pea seedlings treated with a Cd cause a decrease in length and fresh and dry weight of root and shoot (Figs. 1A–1C). Cd toxicity reduces growth due to i) reduced absorption of water and nutrition by root (Wang et al., 2021), ii) damage to the photosynthetic membrane (Sharma et al., 2020b), iii), hindrance during cell division (Mondal et al., 2013), and iv) direct impedance of Cd with some hydrolytic enzymes, and changes in carbohydrates metabolism (Abdel Latef, 2013; Hussain et al., 2013). The exogenous use of SNP mitigates the inhibitory effect of Cd on length, and fresh and dry weight of root and shoot (Figs. 1A–1C), demonstrating that NO plays a direct or indirect function in reducing Cd toxicity in these plants. These findings are consistent with previous research on Typha latifolia and chickpea (Wang et al., 2016; Kumari et al., 2010).
The two probable enzymatic sources of NO synthesis are NR and NOS (Neill et al., 2008). In plants, NR is regarded as the primary enzymatic source for the production of endogenous NO (Li et al., 2016). Several studies have reported NOS activity in plants (Corpas and Barroso, 2013), despite the evidence showing that no NOS gene was reported in plants (Neill et al., 2008). Endogenous NO levels and NR activity were reduced in Cd-treated pea seedlings (Figs. 2A and 2B). Cd causes a reduction in endogenous NO concentration and NR activity which was reversed after an exogenous supply of SNP (Figs. 2A and 2B).
Cd stress causes ROS overproduction leading to oxidative burst and damage to various biomolecules in plants. The outcome of lipid peroxidation is MDA, and its concentration reflects lipid peroxidation and stress level (Abdel Latef, 2011; Ahmad et al., 2015). Enhanced MDA production and loss of membrane permeability have been reported in maize and pepper. In this study, we observed that Cd treatment caused the increase of H2O2 and MDA content in the root and shoot of pea (Figs. 3A and 3B). Similar to our studies, Cd has been reported to cause oxidative stress by enhancing H2O2 and MDA contents in Arabidopsis (Li et al., 2016). H2O2, on the one hand, act as a signaling molecule (Liu and He, 2016; Liu and He, 2017), on the other hand, it is very harmful to most biochemical reactions (Nahar et al., 2021). H2O2 hinders the Calvin cycle by lowering the photosynthetic rate (Hussain et al., 2013), and its high content is generally assumed as a stress condition in plants. When Cd-treated pea plants were supplied with 50 µM SNP, plants showed a decrease in H2O2 and MDA levels than those in plants treated with Cd alone (Fig. 3A and 3B). This could be because of NO-led modulation in antioxidant enzymes activity and proline accumulation, which can detoxify free radicals and decrease oxidative damage of membranes during Cd stress (Mourato et al., 2012; Li et al., 2016).
Heavy metal exposure has been linked to a decrease in the level of soluble proteins (Hasanuzzaman et al., 2014). Our findings showed the reduction of protein content in Cd-treated plants. This may be because of (i) an increase in ROS generation, which damages proteins (Gajewska and Skłodowska, 2007), (ii) binding of Cd to the sulfhydryl group of protein damages the protein structure (Hasanuzzaman et al., 2014), and (iii) active involvement of protease activity (Palma et al., 2002). Plants treated with Cd in combination with 50 µM SNP displayed more protein content related to Cd-treated plants (Fig. 4B). Parallel to our studies, SNP-mediated increase in protein content has been observed under Cd stress in peanuts (Dong et al., 2019) and seawater stress in canola plants (Abdel Latef, 2011). SNP improved the content of protein during abiotic stress, perhaps by increasing protein synthesis and lowering proteolysis and enzyme degradation (Kozlowski and Pallardy, 2002).
Phenolic compounds and flavonoids are well known for their protective role against various stresses in plants. Total phenols and flavonoids represent antioxidant properties because of their capacity to act as electron-donating agents (Salinitro et al., 2020). Flavonoids have also been implicated in metal chelation (Bai et al., 2004). With an increase in Cd concentration, phenols and flavonoid levels decreased in the roots and shoots of pea seedlings (Figs. 5A and 5B). Similar to our studies, the reduction of phenols and flavonoid levels due to Cd was also reported earlier (Kapoor et al., 2014). Cd toxicity causes the overproduction of ROS by inhibiting the enzymes involved in the production of phenols and flavonoid. Phenolic compounds are produced immediately through the signaling processes during stress (Bais et al., 2002). Exogenous supply of SNP along with Cd enhanced the total phenol and flavonoid level in roots and shoots of pea seedlings (Figs. 5A and 5B). This may be probably due to SNP-led (i) decrease in Cd uptake, (ii) enhancement in polyphenols biosynthesis genes expression (Xu et al., 2014), and (iii) stimulation of the activity of phenylalanine ammonia-lyase enzyme, a key enzyme in phenyl propanoid biosynthesis (Kuthanová et al., 2004).
Antioxidant enzymes, including CAT and POD play important roles in plants by avoiding oxidative damage (Lu et al., 2020). Both CAT and POD, decompose H2O2 to H2O and O2 (Ahmad et al., 2015). Compared to CAT, POD possesses a high affinity for H2O2. CAT is responsible for the elimination of excess H2O2, and POD is responsible for keeping H2O2 concentrations low (Mourato et al., 2012; Hasanuzzaman et al., 2020). Our results showed decreased CAT and POD activity in Cd stress pea seedlings (Figs. 6A and 6B). Excessive production of ROS during Cd stress may be the cause of the decrease in antioxidant enzyme activity (Mondal et al., 2013). The use of SNP increased antioxidant enzyme activity and lowered H2O2 and MDA content suggesting that SNP application can be effective in increasing Cd stress tolerance in the plant by enhancing antioxidant systems and protecting the structural and functional damage of cell membranes (Talukdar, 2012; Piacentini et al., 2020).
Our results suggest that the application of SNP along with Cd increases endogenous NO content and antioxidant activity, which might be responsible for the mitigation of Cd-impeded growth in pea seedlings.
Acknowledgement: The authors are thankful to the Department of Biotechnology, Government of India, for the financial support of BUILDER Project No. BT/PR-9028/INF/22/193/2013. EAK acknowledges the receipt of UGC-MANF for minority students, and PS is thankful to UGC-FRP during the period of this work.
Ethics Approval: Not applicable.
Author Contribution: Study conception and design: EAK, MM, PS, ANM; data collection: EAK; analysis and interpretation of results: EAK, PS, MH; draft manuscript preparation: EAK, HMIA, PS, MH. All authors reviewed the results and approved the final version of the manuscript.
Availability of Data and Materials: All data are available in this manuscript, and the materials can be received upon request.
Funding Statement: This work was funded by DBT BUILDER Project No. BT/PR-9028/INF/22/193/2013.
Conflicts of Interest: The authors declare that they have no conflicts of interest to report regarding the present study.
References
Abdel Latef AA (2013). Growth and some physiological activities of pepper (Capsicum annuum L.) in response to cadmium stress and mycorrhizal symbiosis. Journal of Agricultural Science and Technology 15: 1437–1448. [Google Scholar]
Abdel Latef AAH (2011). Ameliorative effect of calcium chloride on growth, antioxidant enzymes, protein patterns and some metabolic activities of canola (Bassica napus L.) under seawater stress. Journal of Plant Nutrition 34: 1303–1320. [Google Scholar]
Aebi H (1984). Catalase in Vitro. Methods in Enzymology 105: 121–126. [Google Scholar]
Ahmad P, Sarwat M, Bhat NA, Wani MR, Kazi AG et al. (2015). Alleviation of cadmium toxicity in, Brassica juncea L. (Czern. & Coss.) by calcium application involves various physiological and biochemical strategies. PLoS One 10: e0114571. [Google Scholar]
Anjum NA, Sharma P, Gill SS, Hasanuzzaman M, Khan EA et al. (2016). Catalase and ascorbate peroxidase—representative H2O2-detoxifying heme enzymes in plants. Environmental Science and Pollution Research 23: 19002–19029. [Google Scholar]
Arasimowicz-Jelonek M, Floryszak-Wieczorek J (2011). Understanding the fate of peroxynitrite in plant cells From physiology to pathophysiology. Phytochemistry 72: 681–688. [Google Scholar]
Bai Y, Song F, Chen M, Xing J, Liu Z et al. (2004). Characterization of the rutin-metal complex by electrospray ionization tandem mass spectrometry. Analytical Sciences 20: 1147–1151. [Google Scholar]
Bais HP, Walker TS, Schweizer HP, Vivanco JM (2002). Root specific elicitation and antimicrobial activity of rosmarinic acid in hairy root cultures of Ocimum basilicum. Plant Physiology and Biochemistry 40: 983–995. [Google Scholar]
Bates LS, Waldren RP, Teare ID (1973). Rapid determination of free proline for water-stress studies. Plant and Soil 39: 205–207. [Google Scholar]
Cheeseman JM (2006). Hydrogen peroxide concentrations in leaves under natural conditions. Journal of Experimental Botany 57: 2435–2444. [Google Scholar]
Cheng J, Qiu H, Chang Z, Jiang Z, Yin W (2016). The effect of cadmium on the growth and antioxidant response for freshwater algae Chlorella vulgaris. SpringerPlus 5: 1290. [Google Scholar]
Corpas FJ, Barroso JB (2013). Nitro-oxidative stress vs. oxidative or nitrosative stress in higher plants. New Phytologist 199: 633–635. [Google Scholar]
Corpas FJ, Barroso JB (2014). Peroxynitrite (ONOO-) is endogenously produced in Arabidopsis peroxisomes and is overproduced under cadmium stress. Annals of Botany 113: 87–96. [Google Scholar]
Dong Y, Chen W, Bai X, Liu F, Wan Y (2019). Effects of exogenous nitric oxide and 24-epibrassinolide on the physiological characteristics of peanut seedlings under cadmium stress. Pedosphere 29: 45–59. [Google Scholar]
Evans HJ, Nason A (1953). Pyridine nucleotide-nitrate reductase from extracts of higher plants. Plant Physiology 28: 233–254. [Google Scholar]
Fancy NN, Bahlmann AK, Loake GJ (2017). Nitric oxide function in plant abiotic stress. Plant Cell and Environment 40: 462–472. [Google Scholar]
Gajewska E, Skłodowska M (2007). Effect of nickel on ROS content and antioxidative enzyme activities in wheat leaves. BioMetals 20: 27–36. [Google Scholar]
Genchi G, Sinicropi MS, Lauria G, Carocci A, Catalano A (2020). The effects of cadmium toxicity. International Journal of Environmental Research and Public Health 17: 3782. [Google Scholar]
Hageman RH, Reed AJ, Femmer RA, Sherrard JH, Dalling MJ (1980). Some new aspects of the in vivo assay for nitrate reductase in wheat (Triticum aestivum L.) leaves. Plant Physiology 65: 27–32. [Google Scholar]
Hasanuzzaman M, Alam MM, Rahman A, Hasanuzzaman M, Nahar K et al. (2014). Exogenous proline and glycine betaine mediated upregulation of antioxidant defense and glyoxalase systems provides better protection against salt-induced oxidative stress in two rice (Oryza sativa L.) varieties. BioMed Research International 2014: 757219. [Google Scholar]
Hasanuzzaman M, Bhuyan MHMB, Parvin K, Bhuiyan TF, Anee TI et al. (2020). Regulation of ROS metabolism in plants under environmental stress: A review of recent experimental evidence. International Journal of Molecular Sciences 21: 1–44. [Google Scholar]
Heath RL, Packer L (1968). Photoperoxidation in isolated chloroplasts: I Kinetics and stoichiometry of fatty acid peroxidation. Archives of Biochemistry and Biophysics 125: 189–198. [Google Scholar]
Huang J, Zhu C, Hussain S, Huang J, Liang Q et al. (2020). Effects of nitric oxide on nitrogen metabolism and the salt resistance of rice (Oryza sativa L.) seedlings with different salt tolerances. Plant Physiology and Biochemistry 155: 374–383. [Google Scholar]
Hussain I, Akhtar S, Ashraf MA, Rasheed R, Siddiqi EH et al. (2013). Response of maize seedlings to cadmium application after different time intervals. ISRN Agronomy 2013: 1–9. [Google Scholar]
Jia ZS, Tang MC, Wu JM (1999). The determination of flavonoid contents in mulberry and their scavenging effects on superoxide radicals. Food Chemistry 64: 555–559. [Google Scholar]
Kapoor D, Kaur S, Bhardwaj R (2014). Physiological and biochemical changes in Brassica juncea plants under Cd-induced stress. BioMed Research International 2014: 726070. [Google Scholar]
Khan EA, Ahmed HMI, Misra M, Sharma P, Misra AN (2022a). Nitric oxide alleviates photochemical damage induced by cadmium stress in Pea seedlings. Phyton-International Journal of Experimental Botany 91: 959–973. DOI 10.32604/phyton.2022.018708. [Google Scholar] [CrossRef]
Khan EA, Misra M, Sharma P, Misra AN (2017). Effects of exogenous nitric oxide on protein, proline, MDA contents and antioxidative system in pea seedling (Pisum sativum L.). Research Journal of Pharmacy and Technology 10: 3137–3142. [Google Scholar]
Khan EA, Upadhyay TK, Prajapat RK, Mathur M (2022b). Chapter 2–Revisiting brassinosteroids signaling in plants. Brassinosteroids in Plant Developmental Biology and Stress Tolerance, 15–41. DOI 10.1016/B978-0-12-813227-2.00010-2. [Google Scholar] [CrossRef]
Kopyra M, Stachoń-Wilk M, Gwóźdź EA (2006). Effects of exogenous nitric oxide on the antioxidant capacity of cadmium-treated soybean cell suspension. Acta Physiologiae Plantarum 28: 525–536. [Google Scholar]
Kozlowski TT, Pallardy SG (2002). Acclimation and adaptive responses of woody plants to environmental stresses. Botanical Review 68: 270–334. [Google Scholar]
Kumar D, Khan EA (2021). Remediation and detection techniques for heavy metals in the environment. In: Heavy Metals in the Environment, pp. 205–222. Springer. [Google Scholar]
Kumari A, Sheokand S, Swaraj K (2010). Nitric oxide induced alleviation of toxic effects of short term and long term Cd stress on growth, oxidative metabolism and Cd accumulation in chickpea. Brazilian Journal of Plant Physiology 22: 271–284. [Google Scholar]
Kuthanová A, Gemperlová L, Zelenková S, Eder J, Macháčková I et al. (2004). Cytological changes and alterations in polyamine contents induced by cadmium in tobacco BY-2 cells. Plant Physiology and Biochemistry 42: 149–156. [Google Scholar]
Li P, Zhao C, Zhang Y, Wang X, Wang X et al. (2016). Calcium alleviates cadmium-induced inhibition on root growth by maintaining auxin homeostasis in Arabidopsis seedlings. Protoplasma 253: 185–200. [Google Scholar]
Li X, Gong B, Xu K (2014). Interaction of nitric oxide and polyamines involves antioxidants and physiological strategies against chilling-induced oxidative damage in Zingiber officinale Roscoe. Scientia Horticulturae 170: 237–248. [Google Scholar]
Liu Y, He C (2016). Regulation of plant reactive oxygen species (ROS) in stress responses: Learning from AtRBOHD. Plant Cell Reports 35: 995–1007. [Google Scholar]
Liu Y, He C (2017). A review of redox signaling and the control of MAP kinase pathway in plants. Redox Biology 11: 192–204. [Google Scholar]
Liu Y, Liu L, Qi J, Dang P, Xia T (2019). Cadmium activates ZmMPK3-1 and ZmMPK6-1 via induction of reactive oxygen species in maize roots. Biochemical and Biophysical Research Communications 516: 747–752. [Google Scholar]
Lu L, Huang M, Huang Y, Corvini PFX, Ji R et al. (2020). Mn3O4 nanozymes boost endogenous antioxidant metabolites in cucumber (Cucumis sativus L) plant and enhance resistance to salinity stress. Environmental Science: Nano 7: 1692–1703. [Google Scholar]
Mohamed AA, Khan EA, Misra AN (2019). Mitigation effect of exogenous nitric oxide (NO) on some metabolic compounds of maize seedling grown under salt stress. Journal of Physics: Conference Series 1294: 52008. [Google Scholar]
Molassiotis A, Tanou G, Diamantidis G (2010). No says more than “YES” to salt tolerance salt priming and systemic nitric oxide signaling in plants. Plant Signaling and Behavior 5: 209–212. [Google Scholar]
Mondal NK, Das C, Roy S, Datta JK (2013). Effect of varying cadmium stress on chickpea (Cicer arietinum L.) seedlings: An ultrastructural study. Annals of Environmental Science 7: 59–70. [Google Scholar]
Moussa HR, El-Gamal SM (2010). Effect of salicylic acid pretreatment on cadmium toxicity in wheat. Biologia Plantarum 54: 315–320. [Google Scholar]
Nabi RBS, Tayade R, Hussain A, Kulkarni KP, Imran QM et al. (2019). Nitric oxide regulates plant responses to drought, salinity, and heavy metal stress. Environmental and Experimental Botany 161: 120–133. [Google Scholar]
Nahar K, Hasanuzzaman M, Alam MU, Anee TI, Bhuiyan TF et al. (2021). Exogenous arginine enhances antioxidant defense system and regulates the physiology of lentil (Lens culinaris) under salt stress. Proceedings 4: 8879. [Google Scholar]
Neill S, Bright J, Desikan R, Hancock J, Harrison J et al. (2008). Nitric oxide evolution and perception. Journal of Experimental Botany 59: 25–35. [Google Scholar]
Noriega GO, Yannarelli GG, Balestrasse KB, Batlle A, Tomaro ML (2007). The effect of nitric oxide on heme oxygenase gene expression in soybean leaves. Planta 226: 1155–1163. [Google Scholar]
Palma JM, Sandalio LM, Javier Corpas F, Romero-Puertas MC, McCarthy I et al. (2002). Plant proteases, protein degradation, and oxidative stress: Role of peroxisomes. Plant Physiology and Biochemistry 40: 521–530. [Google Scholar]
Piacentini D, Ronzan M, Fattorini L, Della Rovere F, Massimi L et al. (2020). Nitric oxide alleviates cadmium- but not arsenic-induced damages in rice roots. Plant Physiology and Biochemistry 151: 729–742. [Google Scholar]
Prasertsongskun S, Sangduen N, Suwanwong S, Santisopasri V, Matsumoto H (2002). A rapid and sensitive method for the quantitation of microgram quantities of protein utilizing the principle of protein-dye binding. Weed Biology and Management 2: 248–254. [Google Scholar]
Salinitro M, Hoogerwerf S, Casolari S, Zappi A, Melucci D, Tassoni A (2020). Production of antioxidant molecules in Polygonum aviculare (L.) and senecio vulgaris (L.) under metal stress: A possible tool in the evaluation of plant metal tolerance. International Journal of Molecular Sciences 21: 1–17. [Google Scholar]
Sharma A, Kumar V, Kumar R, Kohli SK, Yadav P et al. (2020a). Role of plant growth regulators in ameliorating heavy metal caused oxidative stress in plants: An update. In: Metal Toxicity in Higher Plants, pp. 117–135. Elsevier. [Google Scholar]
Sharma A, Kumar V, Shahzad B, Ramakrishnan M, Singh Sidhu GP et al. (2020b). Photosynthetic response of plants under different abiotic stresses: A review. Journal of Plant Growth Regulation 39: 509–531. [Google Scholar]
Sharma P, Jha AB, Dubey RS, Pessarakli M (2012). Reactive oxygen species, oxidative damage, and antioxidative defense mechanism in plants under stressful conditions. Journal of Botany 2012: 1–26. [Google Scholar]
Shetty K, Curtis OF, Levin RE, Witkowsky R, Ang W (1995). Prevention of vitrification asociated with in vitro shoot culture of Oregano (Origanum vulgare L.) by Pseudomonas spp. Journal of Plant Physiology 147: 447–451. [Google Scholar]
Singh HP, Kaur S, Batish DR, Sharma VP, Sharma N et al. (2009). Nitric oxide alleviates arsenic toxicity by reducing oxidative damage in the roots of Oryza sativa (rice). Nitric Oxide 20: 289–297. [Google Scholar]
Soares C, Rodrigues F, Sousa B, Pinto E, Ferreira IMPLVO et al. (2021). Foliar application of sodium nitroprusside boosts solanum lycopersicum l. Tolerance to glyphosate by preventing redox disorders and stimulating herbicide detoxification pathways. Plants 10: 1862. [Google Scholar]
Talukdar D (2012). Exogenous calcium alleviates the impact of cadmium-induced oxidative stress in Lens culinaris medic. Seedlings through modulation of antioxidant enzyme activities. Journal of Crop Science and Biotechnology 15: 325–334. [Google Scholar]
Wang F, Tan H, Huang L, Cai C, Ding Y et al. (2021). Application of exogenous salicylic acid reduces Cd toxicity and Cd accumulation in rice. Ecotoxicology and Environmental Safety 207: 111198. [Google Scholar]
Wang Q, Liang X, Dong Y, Xu L, Zhang X et al. (2013). Effects of exogenous nitric oxide on cadmium toxicity, element contents and antioxidative system in perennial ryegrass. Plant Growth Regulation 69: 11–20. [Google Scholar]
Wang YJ, Dong YX, Wang J, Cui XM (2016). Alleviating effects of exogenous NO on tomato seedlings under combined Cu and Cd stress. Environmental Science and Pollution Research 23: 4826–4836. [Google Scholar]
Xu W, Peng H, Yang T, Whitaker B, Huang L et al. (2014). Effect of calcium on strawberry fruit flavonoid pathway gene expression and anthocyanin accumulation. Plant Physiology and Biochemistry 82: 289–298. [Google Scholar]
Zaid A, Wani SH (2019). Reactive oxygen species generation, scavenging and signaling in plant defense responses. In: Bioactive Molecules in Plant Defense: Signaling in Growth and Stress, pp. 111–132. Cham: Springer. [Google Scholar]
Zhang Q, Hou Q, Huang G, Fan Q (2020). Removal of heavy metals in aquatic environment by graphene oxide composites: A review. Environmental Science and Pollution Research 27: 190–209. DOI 10.1007/s11356-019-06683-w. [Google Scholar] [CrossRef]
Zhang Y, Han X, Chen X, Jin H, Cui X (2009). Exogenous nitric oxide on antioxidative system and ATPase activities from tomato seedlings under copper stress. Scientia Horticulturae 123: 217–223. DOI 10.1016/j.scienta.2009.08.015. [Google Scholar] [CrossRef]
Cite This Article
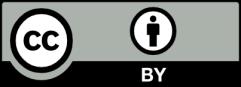