Open Access
REVIEW
Hypoxia-induced reactive oxygen species in organ and tissue fibrosis
1 West China School of Public Health and West China Fourth Hospital, Sichuan University, Chengdu, 610041, China
2 Institute of Biomedical Engineering, West China School of Basic Medical Sciences and Forensic Medicine, Sichuan University, Chengdu, 610041, China
* Corresponding Author: YE ZENG. Email:
BIOCELL 2023, 47(2), 261-267. https://doi.org/10.32604/biocell.2023.024738
Received 07 June 2022; Accepted 15 July 2022; Issue published 18 November 2022
Abstract
Fibrosis is the end-stage change of damaged tissues in various human diseases, which can lead to permanent scarring or organ malfunction. Hypoxia leads to oxidative stress, mitochondrial dysfunction, and inflammation in dysfunctional organs and tissues. Oxidative stress resulting from the overproduction of reactive oxygen species plays a central role in the fibrosis of injured organs. This review addresses the updated knowledge of the relationship between hypoxia and tissue fibrosis mediated by the reactive oxygen species pathway. Moreover, novel anti-fibrotic strategies are discussed, which may suppress reactive oxygen species and organ fibrosis.Keywords
Fibrosis is an unregulated tissue repair process in various human diseases (Xie and Zeng, 2020). It is characterized by the differentiation of fibroblast/myofibroblasts from epithelial cells and excessive extracellular matrix (ECM) accumulation, accompanied by inflammatory injury and tissue structure destruction. That is, the abnormal repair of damaged tissues leads to structural destruction. After tissue injury, perfusion is disrupted, and acute tissue hypoxia results. The high demand for oxygen in wounded tissues or inflammatory cells and mesenchymal cells that infiltrate the tissues sustain the hypoxic state of damaged tissues (Remensnyder and Majno, 1968). Prolonged or chronic hypoxia may help the pathological repair, such as angiogenesis and fibrosis (Xie and Zeng, 2020; Yao et al., 2021). Fibrosis can occur in various diseases involving nearly every tissue and organ (lung, liver, kidney, heart, skin, connective tissue, etc.). A large amount of evidence over the past several decades has identified many of the essential pathogenic mechanisms that promote fibrosis in hypoxia, yet the precise molecular mechanism involved and the crosstalk between the implicated pathways are not fully understood (Cheresh et al., 2013; Remensnyder and Majno, 1968).
Oxidative stress (OS) is one of the major components contributing to organ fibrosis, the common pathway leading to organ dysfunction and death. OS results from increased endogenous and exogenous reactive oxygen species (ROS) levels. ROS is an important pathogenic factor underlying fibrosis in a variety of organs. ROS increase in patients with idiopathic pulmonary fibrosis (IPF) or silicosis-induced lung fibrosis and silica or asbestos-induced fibrosis animal models (Cheresh et al., 2013). Additionally, diverse renal abnormalities trigger OS and accelerate the disorders of the kidney, such as acute kidney injury (AKI), chronic kidney disease, and nephrotic syndrome (Su et al., 2019). ROS can promote cell apoptosis and necrosis, elicit an inflammatory response, and trigger the overproduction of pro-inflammatory cytokines that may be important for forming and depositing ECM.
Recent studies have shown elevated levels of ROS biomarkers in plasma, urine, and exhaled breath in hypoxia patients (Prabhakar et al., 2007). Continuous positive airway pressure therapy could reverse hypoxia and thus decrease circulating ROS levels in elderly and obese patients (Chen et al., 2020). Lack of oxygen enhances reductive carboxylation through a hypoxia-inducible factor-1α (HIF-1α)-dependent mechanism, increasing ROS production (Jiang et al., 2016). Hypoxia also alters the complex structure and activity of the electron transport chain (ETC) and results in excessive ROS formation. Besides hypoxia, re-oxygenation seems to trigger ROS production (Diaz et al., 2012; Guarás et al., 2016). A study on a model of glaucoma showed that intraocular pressure elevation could reduce oxygen supply to the retina, subjecting the retina and optic nerve to hypoxic conditions (Chidlow et al., 2017; Kamel et al., 2020). Increased ROS concomitant with HIF-1α upregulation was observed in hypoxic cells, which led to reduced mitophagy (Jassim et al., 2021). Here, we reviewed the role of hypoxia-induced ROS in organ and tissue fibrosis and highlighted the complex interplays between hypoxia signaling and ROS production. This review will provide better insight into the pathological progress of fibrosis and help in developing new anti-fibrotic therapy strategies.
The key role of ROS in organ and tissue fibrosis
ROS is a double-edged sword. A Low level of ROS is necessary as potent signaling molecules are involved in the regulatory processes of various physiological events. At the same time, excessive ROS causes cell damage and organ and tissue injury. ROS has been identified to play an essential role in various organ and tissue fibrosis. ROS production was found in chronic renal abnormalities, which may result in tubulointerstitial fibrosis by myofibroblast activation as well as in glomerulosclerosis by mesangial sclerosis, podocyte abnormality, and parietal epithelial cell injury (Choi et al., 2014; Fassett et al., 2015). ROS is a key process in driving liver damage; the initiation of liver fibrosis by disrupting cellular lipids, proteins, and DNA, and leads to hepatocyte necrosis and apoptosis. Moreover, ROS could induce epigenetic changes in the hepatic stellate cells (HSCs), including chromatin remodeling by histone modification, DNA methylation, and gene degradation by microRNAs (Moran-Salvador and Mann, 2017; Roehlen et al., 2020). ROS was found to be associated with type-II epithelial-to-mesenchymal transition (EMT) in case of oral sub-mucous fibrosis (OSF), attributing to the development of sub-epithelial fibrosis and type-III EMT in case of oral squamous cell carcinoma (OSCC) favoring malignancy associated metastasis (Kawami et al., 2022). Alteration of Shh and Gli-1 expression patterns revealed the mechanistic association of hypoxia with the phenotypic plasticity and disease manifestation in the case of OSF and OSCC. Shh/Gli-1 signaling can also be correlated with survival in mediating the cytoprotective phenomenon under OS (Chatterjee et al., 2021). ROS is particularly linked to pulmonary fibrosis due to its exposure to higher oxygen tensions than other tissues. Besides the common molecular mechanism like mitochondrial DNA damage, pro-inflammation, and fibroblast proliferation, ROS is implicated in specific fibrotic processes such as macrophage polarization, alveolar epithelial cell apoptosis, myofibroblast differentiation and senescence, and alterations in the extracellular ECM underlying pulmonary fibrosis (Liu and Chen, 2017; Otoupalova et al., 2020).
Another chronic lung fibrosis disease different from IPF is cystic fibrosis (CF). CF is a genetic fibrosis disease that commonly occurs due to mutations of the gene encoding the protein cystic fibrosis transmembrane conductance regulator (CFTR). In the CF airway, many activated neutrophils release ROS and proteases, thereby inducing additional ROS production in the lungs (Künzi et al., 2021). Both in vitro and in vivo experiments demonstrated that CFTR is involved in stabilizing HIF1-α via controlling intracellular ROS generation during hypoxia (Duranton et al., 2012). Phagocytic cells play a significant role in the host immune defenses by inducing the oxidative burst response and the secretion of ROS. The up-regulation of Scedosporium boydii catalase A1 gene (CATA1) and the constitutive expression of Cu, Zn-SOD gene (SODC) in response to oxidative stress in phagocytic cells may be helpful for the survival of the fungus during the inflammation in fibrosis process (Mina et al., 2015).
Both acute and chronic intermittent hypoxia exhibit an oxidative burst during re-oxygenation, which can cause cellular injury (Granger and Kvietys, 2015). The oxidative burst results from the production and accumulation of ROS, such as superoxide anion and hydrogen peroxide. The overproduction of ROS provokes oxidative DNA damage, lipid peroxidation, and protein denaturation. The cerebral hypoxia-ischemia can induce increased levels of ROS and inflammatory response and result in neurodegeneration (Merelli et al., 2021). The concentration of malondialdehyde (MDA), a type of ROS-induced product, increased significantly after hypoxia stress (Jie et al., 2021). A large body of evidence indicates that hyperglycemia-induced chronic hypoxia is one of the common pathogenic mechanisms driving the progression of diabetic nephropathy (DN) (Fu et al., 2016; Rosenberger et al., 2008). Further study confirmed that hyperglycemia induces cellular hypoxia and mitochondrial ROS generation, which may promote podocyte injury in DN (Sada et al., 2016). Tangeretin can inhibit podocyte injury and fibrosis by blocking EMT caused by ROS and hypoxia (Kang et al., 2020). Hypoxia leads to ATP depletion and ROS-induced damage to cellular components, which consequently cause age-related macular degeneration (Blasiak et al., 2014). In rheumatoid arthritis, hypoxia induces excessive production of ROS leading to oxidative damage (Quiñonez-Flores et al., 2016). A comparative metabolomics study of hypoxic rodent tissues revealed that hypoxic conditions lead to an accumulation of mitochondrial succinate due to the reversal of the succinate dehydrogenase enzyme acting on fumarate in the citric acid cycle. When reperfusion occurs, this accumulated succinate is rapidly re-oxidized, generating large amounts of superoxide radical and other free radical species (Chouchani et al., 2014). Intermittent hypoxia worsened the fibrosis in bleomycin (BLM)-treated mice. Hypoxia increases the levels of ROS and inflammation with the progress of pulmonary fibrosis, and there is a positive linear correlation between the HIF-1α expression and hydroxyproline content (a biomarker of fibrosis) (Xiong et al., 2021).
The central role of ROS in crosstalk between the pathways
The cellular reactions to hypoxia evoke a change in the expression of many genes. HIF-1 is the primary regulator of oxygen homeostasis and consists of HIF-1α and HIF-1β subunits. HIF-1α is uniquely associated with the transcription of the hypoxia-inducible genes. HIF-1α can regulate the expression of roughly 400 target genes to ensure cell survival under hypoxic stress conditions (Schodel et al., 2011). HIF-1α targets genes such as BNIP3L, FLT1, SIAH1, BHLHB2, and vascular endothelial growth factor (VEGF) were found to be significantly overexpressed in BLM-induced pulmonary inflammation and fibrosis. Both mRNA and protein levels of HIF-1α were found to be remarkably up-regulated as early as seven days post-BLM treatment, before any destruction of lung architecture and consequent gas exchange problems, indicating an early role of HIF-1α in the fibrosis process (Tzouvelekis et al., 2007). The enlargement and leakage of skin capillaries in active stages develop into an extensive vascular area associated with severe tissue hypoxia in systemic sclerosis. Hypoxia was found to up-regulate the expression of VEGF in all stages of the disease, accompanied by strongly reactive HIF-1α (Ioannou et al., 2013). Excessive production of ROS could impair VEGF-stimulated angiogenesis and wound healing (Liu et al., 2022). Other vascular bioactive factors, such as sphingosine 1-phosphate, might enhance the expression of HIF-1α (Hutami et al., 2022; Zeng et al., 2022), contributing to the regulation of wound healing and organ fibrosis. In vitro, hypoxia induces the expression of VEGF and type I collagen in activated HSCs in experimental hepatic fibrosis (Corpechot et al., 2002). HIF-1α enhanced EMT in vitro and induced epithelial cell migration through upregulation of lysyl oxidase (LOX) genes. In contrast, genetic ablation of epithelial HIF-1α inhibited the development of tubulointerstitial fibrosis in unilateral ureteral obstruction (UUO) kidneys, associated with decreased fibrogenesis (Higgins et al., 2007). Additionally, hypoxia serves as a stimulus for endothelial to mesenchymal transition (EndMT) via up-regulated HIF-1α target transcription factors Snail, Slug, and Twist (Xu et al., 2015). Hypoxia activates nuclear factor-activated T cell signaling via increased HIF-2α but not HIF-1α, then increases the proliferation of normal human pulmonary fibroblasts and IPF fibroblasts (Senavirathna et al., 2018).
Mitochondria are the significant consumers of oxygen in the cell and are severely affected by hypoxia consequently. Mitochondria have emerged as a powerful source of ROS under hypoxia conditions, and mitochondrial DNA (mtDNA) is more susceptible to oxidative damage than its nuclear counterpart (McElroy and Chandel, 2017). Ischemia-reperfusion initiates oxidative damage through the generation of mitochondrial ROS, and the repeated cycles of hypoxia-reoxygenation can lead to increased levels of ROS (Bugger and Pfeil, 2020). HIF-1α is recruited to the mitochondria in response to ROS, and clearance of the ROS thus protects against hydrogen peroxide (H2O2)-induced apoptosis (Li et al., 2019). Increased intracellular ROS levels enhance HIF-1α gene expression in OSCC cells under normoxia, which is initiated through transcriptional and translational regulation of HIF-1α via the extracellular regulated protein kinase (ERK) and phosphatidylinositol 3 kinase/serine-threonine kinase (PI3K/AKT) signaling pathway (Sasabe et al., 2010). Under hypoxia conditions, ROS can also increase the transcription of HIF-1α by induction of ERK and PI3K/AKT phosphorylation (Du et al., 2011; Koshikawa et al., 2009). ROS, especially H2O2, inhibits the activity of prolyl hydroxylases (PHD), thereby interfering with HIF-1α degradation and favoring the accumulation of HIF-α (Dehne and Brune, 2014). Several cell types respond to acute hypoxia with a transient increase in ROS production for about 10 min, accompanied by the stabilization of HIF-1α (Hernansanz-Agustin et al., 2014). Inhibition of the mitochondrial anti-oxidative system may increase the expression of HIF-1α. Suppression of THE gene associated with retinoid-IFN-induced mortality-19 (an essential subunit of mitochondrial complex I) by shRNA enhanced autophagy by activating ERK and HIF-1α in a ROS-mediated manner (Yue et al., 2016). Inhibition of mitochondrial complex II, either pharmacologically or via RNA interference of succinate dehydrogenase complex iron-sulfur subunit B, could increase ROS production and, subsequently, the ability to stabilize HIF-1α (Guzy et al., 2008). Deregulation of electron transfer chain complex III results in the release of ROS and thus stabilizes HIF-1α (Comito et al., 2011). In turn, HIF-1α suppresses metabolism through the tricarboxylic acid cycle by directly inducing pyruvate dehydrogenase kinase 1 and leads to hypoxic ROS generation (Kim et al., 2006). A recent study indicated that mitochondria can modulate HIF-1α stability via their effects as oxygen consumers and not as peroxide second messenger generators (Kumar et al., 2021).
Nuclear factor-κB (NF-κB) regulates the expression of genes involved in inflammation, cell proliferation, fibrosis, and apoptosis. ROS is considered a essential second messenger for NF-κB activation. Treating cells with ROS such as H2O2, superoxide anion (O2−), and hydroxyl radical (OH−) results in NF-κB activation. Conversely, antioxidants such as pyrrolidine-9-dithiocarbamate and N-acetyl-cysteine block NF-κB activation by a wide range of stimuli such as interleukin-1(IL-1) and tumor necrosing factor-α (TNF-α), suggesting that ROS act as signaling molecules in NF-κB activation during tissue damage (Bonizzi et al., 2000; Gloire et al., 2006; Panopoulos et al., 2005). Pieces of evidence from cellular experiments revealed the role of ROS in the development and progression of transforming growth factor (TGF)-β1-induced renal diseases. ROS-induced oxidation in latency-associated peptide-β1 triggers a conformational change that releases TGF--β1 (Jobling et al., 2006). Glucose oxidase can continuously generate H2O2 from glucose and thereby induce TGF-β1 synthesis and ECM gene expression in cultured human mesangial cells (Iglesias-de la Cruz et al., 2001). The overexpression of TGF-β1 is stimulated by exogenous as well as endogenous ROS, and decreased intracellular ROS by catalase addition can reduce the protein and mRNA expression of TGF-β1 (González-Ramos et al., 2012). Hypoxia enhances type-II arginase (Arg-II) expression through HIF1α or HIF2α and induces mitochondrial ROS generation, which mediates hypoxia-induced TGF-β1 production in the renal epithelial cells, suggesting that hypoxia participates in hypoxia-associated fibrosis by activating HIFs-Arg-II-ROS-TGFβ1-cascade (Liang et al., 2021).
ROS plays a central role in the pathways mediating organ fibrosis (Fig. 1).
Figure 1: ROS plays a central role in the pathways mediating the organ fibrosis.
Therapy strategies and perspective
It has been well known that glucocorticoids, colchicine, and cyclophosphamides are regularly used to treat organ fibrosis. Some new therapy have emerged recently. For example, baicalin attenuates ROS-associated damage in BLM-induced pulmonary fibrosis by reducing the MDA content, simultaneously increasing the levels of glutathione peroxidase (GSH-px), superoxide dismutase (SOD), and GSH (Zhao et al., 2020), and also in carbon tetrachloride-induced liver fibrosis (Li and Zheng, 2022).
As mitochondrial ROS accounts for most of the cellular ROS in crystalline silica-exposed macrophages, treatment with dioscin could decrease ROS content by promoting alveolar macrophage autophagy activation in vitro and in vivo (Du et al., 2019). Hippophae rhamnoide L and nanocurcumin decreased ROS production in rats exposed to hypobaric hypoxia (Nehra et al., 2017). ROS plays a pivotal role in promoting the activation of some kinases, such as p38 mitogen-activated protein kinase (MAPK), protein kinase C, and ERK 1/2 in hypoxia conditions (Bugger and Pfeil, 2020).
Salubrinal, an inhibitor of p-eIF2α dephosphorylation, could inhibit ROS and contribute to attenuating pulmonary arterial hypertension (PAH) and right ventricular hypertrophy in rats exposed to hypobaric hypoxia (He et al., 2016). Likewise, Ganoderma lucidum could decrease cellular ROS levels in ultraviolet B-exposed fibroblasts (Zeng et al., 2017) and significantly hinder UUO-induced renal fibrosis via suppressing the TGF-β/Smad and MAPK signaling pathways (Geng et al., 2020). Chrysin could decrease local tissue hypoxia in a model of BLM-induced pulmonary fibrosis. Chrysin also demonstrated a potent antioxidant effect by decreasing lipid peroxidation, increasing antioxidant defense mechanisms by increasing SOD activity and endothelial nitric oxide synthase (eNOS) expression, and reducing GSH and inducible NOS (iNOS) expression (Kseibati et al., 2020). Kaempferol showed therapeutic effects in BLM-induced dermal fibrosis by inhibiting the expression of OS-associated factors and decreasing the accumulation of ROS-induced fibroblasts and apoptotic cells (Sekiguchi et al., 2019). Quercetin exerts anti-fibrogenic and anti-inflammatory effects on BLM-induced pulmonary damage in mice through modulation of the redox balance by inducing redox-sensitive transcription factor nuclear factor erythroid 2-related factor 2 (Nrf2) (Boots et al., 2020).
Cell therapy using mesenchymal stem cells (MSCs) represents a promising therapeutic approach to fibrosis diseases. Allogeneic human cells in patients with idiopathic pulmonary fibrosis via intravenous delivery (AETHER) was the first human trial designed to evaluate the safety of bone marrow–derived human MSCs in patients with mild to moderate IPF. Data from this trial supported the safety of a single infusion of human MSCs in patients (Glassberg et al., 2017). Further study confirmed that therapy with high doses of MSCs was a safe and promising method to reduce IPF progression (Averyanov et al., 2020; Fishman et al., 2019). In various murine alveolar injury models, MSCs exerted an overall protective effect, limiting lung inflammation and fibrosis. MSC inhibited HIF-1α protein expression and ROS accumulation (Bernard et al., 2018). Also, purified exosomes derived from MSCs effectively prevented and reverted core features of BLM-induced pulmonary fibrosis (Mansouri et al., 2019).
Several studies have suggested that MSCs have strong immunomodulatory properties. Activated innate immune cells (neutrophils, monocytes, macrophages, dendritic cells, etc.) contribute to fibrosis by secreting ROS and pro-inflammatory mediators such as TNF-α and IL-6 (Sun et al., 2020a). During immune responses, most immune cells undergo metabolic reprogramming, which produces high levels of cytosolic ROS and mitochondrial ROS (Sun et al., 2020b). Evaluating drug interactions with innate immune cells helps to broaden the understanding of anti-fibrotic strategy. A recent study demonstrated the anti-fibrotic potential of pirfenidone by decreasing neutrophil phagocytosis and ROS production (Evani et al., 2020).
In conclusion, the evidence supporting OS in human fibrotic disorders, particularly IPF, is substantial and incontrovertible. The precise role of ROS must be recognized when designing antioxidant therapies. Mitochondrial dysfunction, redox imbalance, and cellular senescence are closely associated with ROS production, which are new targets in organ fibrosis treatment (Otoupalova et al., 2020). Nevertheless, antioxidant therapy is a promising strategy for organ and tissue fibrosis. We hope that with a greater understanding of the pharmacokinetic and pharmacodynamic properties of the bioactive agents and their interactions in vivo, antioxidant-based therapeutics may be used in the clinic for the treatment of specific fibrotic diseases.
Author Contribution: The authors confirm contribution to the paper as follows: study conception and design: Y.Z., and LS.X. All authors draft manuscript preparation and approved the final version of the manuscript.
Ethics Approval: Not applicable.
Funding Statement: This work was supported by the Science and Technology Department of Sichuan Province (No. 2021YFS0182), Science and Technology Department of Tibet (No.XZ202201ZY0033G), and Sichuan Provincial Administration of Traditional Chinese Medicine (No. 2021MS088).
Conflicts of Interest: The authors declare that they have no conflicts of interest to report regarding the present study.
References
Averyanov A, Koroleva I, Konoplyannikov M, Revkova V, Lesnyak V et al. (2020). First-in-human high-cumulative-dose stem cell therapy in idiopathic pulmonary fibrosis with rapid lung function decline. Stem Cells Translational Medicine 9: 6–16. DOI 10.1002/sctm.19-0037. [Google Scholar] [CrossRef]
Bernard O, Jeny F, Uzunhan Y, Dondi E, Terfous R et al. (2018). Mesenchymal stem cells reduce hypoxia-induced apoptosis in alveolar epithelial cells by modulating HIF and ROS hypoxic signaling. American Journal of Physiology Lung Cellular and Molecular Physiology 314: L360–L371. DOI 10.1152/ajplung.00153.2017. [Google Scholar] [CrossRef]
Blasiak J, Petrovski G, Veréb Z, Facskó A, Kaarniranta K (2014). Oxidative stress, hypoxia, and autophagy in the neovascular processes of age-related macular degeneration. BioMed Research International 2014: 768026. DOI 10.1155/2014/768026. [Google Scholar] [CrossRef]
Bonizzi G, Piette J, Merville MP, Bours V (2000). Cell type-specific role for reactive oxygen species in nuclear factor-kappaB activation by interleukin-1. Biochemical Pharmacology 59: 7–11. DOI 10.1016/S0006-2952(99)00290-7. [Google Scholar] [CrossRef]
Boots AW, Veith C, Albrecht C, Bartholome R, Drittij MJ et al. (2020). The dietary antioxidant quercetin reduces hallmarks of bleomycin-induced lung fibrogenesis in mice. BMC Pulmonary Medicine 20: 112. DOI 10.1186/s12890-020-1142-x. [Google Scholar] [CrossRef]
Bugger H, Pfeil K (2020). Mitochondrial ROS in myocardial ischemia reperfusion and remodeling. Biochimica et Biophysica Acta-Molecular Basis of Disease 1866: 165768. DOI 10.1016/j.bbadis.2020.165768. [Google Scholar] [CrossRef]
Chatterjee R, Ghosh B, Mandal M, Nawn D, Banerjee S et al. (2021). Pathophysiological relationship between hypoxia associated oxidative stress, Epithelial-mesenchymal transition, stemness acquisition and alteration of Shh/Gli-1 axis during oral sub-mucous fibrosis and oral squamous cell carcinoma. European Journal of Cell Biology 100: 151146. DOI 10.1016/j.ejcb.2020.151146. [Google Scholar] [CrossRef]
Chen Q, Chen LD, Chen MX, Wu YH, Zeng HX et al. (2020). The effect of continuous positive airway pressure on circulating malondialdehyde among obstructive sleep apnea patients: A meta-analysis. Sleep and Breathing 24: 1407–1415. DOI 10.1007/s11325-019-01998-x. [Google Scholar] [CrossRef]
Cheresh P, Kim SJ, Tulasiram S, Kamp DW (2013). Oxidative stress and pulmonary fibrosis. Biochimca et Biophysica Acta 1832: 1028–1040. DOI 10.1016/j.bbadis.2012.11.021. [Google Scholar] [CrossRef]
Chidlow G, Wood JPM, Casson RJ (2017). Investigations into hypoxia and oxidative stress at the optic nerve head in a rat model of glaucoma. Frontiers in Neuroscience 11: 478. DOI 10.3389/fnins.2017.00478. [Google Scholar] [CrossRef]
Choi BH, Kang KS, Kwak MK (2014). Effect of redox modulating NRF2 activators on chronic kidney disease. Molecules 19: 12727–12759. DOI 10.3390/molecules190812727. [Google Scholar] [CrossRef]
Chouchani ET, Pell VR, Gaude E, Aksentijević D, Sundier SY et al. (2014). Ischaemic accumulation of succinate controls reperfusion injury through mitochondrial ROS. Nature 515: 431–435. DOI 10.1038/nature13909. [Google Scholar] [CrossRef]
Comito G, Calvani M, Giannoni E, Bianchini F, Calorini L et al. (2011). HIF-1alpha stabilization by mitochondrial ROS promotes Met-dependent invasive growth and vasculogenic mimicry in melanoma cells. Free Radical Biology & Medicine 51: 893–904. DOI 10.1016/j.freeradbiomed.2011.05.042. [Google Scholar] [CrossRef]
Corpechot C, Barbu V, Wendum D, Kinnman N, Rey C et al. (2002). Hypoxia-induced VEGF and collagen I expressions are associated with angiogenesis and fibrogenesis in experimental cirrhosis. Hepatology 35: 1010–1021. DOI 10.1053/jhep.2002.32524. [Google Scholar] [CrossRef]
Dehne N, Brune B (2014). Sensors, transmitters, and targets in mitochondrial oxygen shortage–a hypoxia-inducible factor relay story. Antioxid Redox Signal 20: 339–352. DOI 10.1089/ars.2012.4776. [Google Scholar] [CrossRef]
Diaz F, Enríquez JA, Moraes CT (2012). Cells lacking Rieske iron-sulfur protein have a reactive oxygen species-associated decrease in respiratory complexes I and IV. Molecular and Cellular Biology 32: 415–429. DOI 10.1128/MCB.06051-11. [Google Scholar] [CrossRef]
Du J, Xu R, Hu Z, Tian Y, Zhu Y et al. (2011). PI3K and ERK-induced Rac1 activation mediates hypoxia-induced HIF-1alpha expression in MCF-7 breast cancer cells. PLoS One 6: e25213. DOI 10.1371/journal.pone.0025213. [Google Scholar] [CrossRef]
Du S, Li C, Lu Y, Lei X, Zhang Y et al. (2019). Dioscin alleviates crystalline silica-induced pulmonary inflammation and fibrosis through promoting alveolar macrophage autophagy. Theranostics 9: 1878–1892. DOI 10.7150/thno.29682. [Google Scholar] [CrossRef]
Duranton C, Rubera I, Cougnon M, Melis N, Chargui A et al. (2012). CFTR is involved in the fine tuning of intracellular redox status: Physiological implications in cystic fibrosis. The American Journal of Pathology 181: 1367–1377. DOI 10.1016/j.ajpath.2012.06.017. [Google Scholar] [CrossRef]
Evani SJ, Karna SLR, Seshu J, Leung KP (2020). Pirfenidone regulates LPS mediated activation of neutrophils. Scientific Reports 10: 19936. DOI 10.1038/s41598-020-76271-3. [Google Scholar] [CrossRef]
Fassett RG, Robertson IK, Ball MJ, Geraghty DP, Coombes JS (2015). Effects of atorvastatin on oxidative stress in chronic kidney disease. Nephrology 20: 697–705. DOI 10.1111/nep.12502. [Google Scholar] [CrossRef]
Fishman JE, Kim GJ, Kyeong NY, Goldin JG, Glassberg MK (2019). Intravenous stem cell dose and changes in quantitative lung fibrosis and DLCO in the AETHER trial: A pilot study. European Review for Medical and Pharmacological Sciences 23: 7568–7572. DOI 10.26355/eurrev_201909_18877. [Google Scholar] [CrossRef]
Fu Q, Colgan SP, Shelley CS (2016). Hypoxia: The force that drives chronic kidney disease. Clinical Medicine & Research 14: 15–39. DOI 10.3121/cmr.2015.1282. [Google Scholar] [CrossRef]
Geng XQ, Ma A, He JZ, Wang L, Jia YL et al. (2020). Ganoderic acid hinders renal fibrosis via suppressing the TGF-β/Smad and MAPK signaling pathways. Acta Pharmacologica Sinica 41: 670–677. DOI 10.1038/s41401-019-0324-7. [Google Scholar] [CrossRef]
Glassberg MK, Minkiewicz J, Toonkel RL, Simonet ES, Rubio GA et al. (2017). Allogeneic human mesenchymal stem cells in patients with idiopathic pulmonary fibrosis via intravenous delivery (AETHERa phase I safety clinical trial. Chest 151: 971–981. DOI 10.1016/j.chest.2016.10.061. [Google Scholar] [CrossRef]
Gloire G, Legrand-Poels S, Piette J (2006). NF-κB activation by reactive oxygen species: Fifteen years later. Biochemical Pharmacology 72: 1493–1505. DOI 10.1016/j.bcp.2006.04.011. [Google Scholar] [CrossRef]
González-Ramos M, Mora I, de Frutos S, Garesse R, Rodríguez-Puyol M et al. (2012). Intracellular redox equilibrium is essential for the constitutive expression of AP-1 dependent genes in resting cells: Studies on TGF-β1 regulation. The International Journal of Biochemistry & Cell Biology 44: 963–971. DOI 10.1016/j.biocel.2012.03.003. [Google Scholar] [CrossRef]
Granger DN, Kvietys PR (2015). Reperfusion injury and reactive oxygen species: The evolution of a concept. Redox Biology 6: 524–551. DOI 10.1016/j.redox.2015.08.020. [Google Scholar] [CrossRef]
Guarás A, Perales-Clemente E, Calvo E, Acín-Pérez R, Loureiro-Lopez M et al. (2016). The CoQH2/CoQ ratio serves as a sensor of respiratory chain efficiency. Cell Reports 15: 197–209. DOI 10.1016/j.celrep.2016.03.009. [Google Scholar] [CrossRef]
Guzy RD, Sharma B, Bell E, Chandel NS, Schumacker PT (2008). Loss of the SdhB, but not the SdhA, subunit of complex II triggers reactive oxygen species-dependent hypoxia-inducible factor activation and tumorigenesis. Molecular and Cellular Biology 28: 718–731. DOI 10.1128/MCB.01338-07. [Google Scholar] [CrossRef]
He YY, Liu CL, Li X, Li RJ, Wang LL et al. (2016). Salubrinal attenuates right ventricular hypertrophy and dysfunction in hypoxic pulmonary hypertension of rats. Vascular Pharmacology 87: 190–198. DOI 10.1016/j.vph.2016.09.009. [Google Scholar] [CrossRef]
Hernansanz-Agustin P, Izquierdo-Alvarez A, Sanchez-Gomez FJ, Ramos E, Villa-Pina T et al. (2014). Acute hypoxia produces a superoxide burst in cells. Free Radical Biology & Medicine 71: 146–156. DOI 10.1016/j.freeradbiomed.2014.03.011. [Google Scholar] [CrossRef]
Higgins DF, Kimura K, Bernhardt WM, Shrimanker N, Akai Y et al. (2007). Hypoxia promotes fibrogenesis in vivo via HIF-1 stimulation of epithelial-to-mesenchymal transition. The Journal of Clinical Investigation 117: 3810–3820. DOI 10.1172/JCI30487. [Google Scholar] [CrossRef]
Hutami IR, Izawa T, Khurel-Ochir T, Sakamaki T, Iwasa A et al. (2022). HIF-1α controls palatal wound healing by regulating macrophage motility via S1P/S1P(1). Signaling axis. Oral Diseases 28: 1157–1169. DOI 10.1111/odi.13856. [Google Scholar] [CrossRef]
Iglesias-de la Cruz MC, Ruiz-Torres P, Alcamí J, Díez-Marqués L, Ortega-Velázquez R et al. (2001). Hydrogen peroxide increases extracellular matrix mRNA through TGF-β in human mesangial cells. Kidney International 59: 87–95. DOI 10.1046/j.1523-1755.2001.00469.x. [Google Scholar] [CrossRef]
Ioannou M, Pyrpasopoulou A, Simos G, Paraskeva E, Nikolaidou C et al. (2013). Upregulation of VEGF expression is associated with accumulation of HIF-1α in the skin of naïve scleroderma patients. Modern Rheumatology 23: 1245–1248. DOI 10.3109/s10165-012-0787-6. [Google Scholar] [CrossRef]
Jassim AH, Fan Y, Pappenhagen N, Nsiah NY, Inman DM (2021). Oxidative stress and hypoxia modify mitochondrial homeostasis during glaucoma. Antioxid & Redox Signalling 35: 1341–1357. DOI 10.1089/ars.2020.8180. [Google Scholar] [CrossRef]
Jiang L, Shestov AA, Swain P, Yang C, Parker SJ et al. (2016). Reductive carboxylation supports redox homeostasis during anchorage-independent growth. Nature 532: 255–258. DOI 10.1038/nature17393. [Google Scholar] [CrossRef]
Jie YK, Cheng CH, Wang LC, Ma HL, Deng YQ et al. (2021). Hypoxia-induced oxidative stress and transcriptome changes in the mud crab (Scylla paramamosain). Comparative Biochemistry and Physiology. Toxicology & Pharmacology 245: 109039. DOI 10.1016/j.cbpc.2021.109039. [Google Scholar] [CrossRef]
Jobling MF, Mott JD, Finnegan MT, Jurukovski V, Erickson AC et al. (2006). Isoform-specific activation of latent transforming growth factor β (LTGF-β). By reactive oxygen species. Radiation Research 166: 839–848. DOI 10.1667/RR0695.1. [Google Scholar] [CrossRef]
Kamel K, O’Brien CJ, Zhdanov AV, Papkovsky DB, Clark AF et al. (2020). Reduced oxidative phosphorylation and increased glycolysis in human glaucoma lamina cribrosa cells. Investigative Ophthalmology & Visual Science 61: 4. DOI 10.1167/iovs.61.13.4. [Google Scholar] [CrossRef]
Kang MK, Kim SI, Oh SY, Na W, Kang YH (2020). Tangeretin ameliorates glucose-induced podocyte injury through blocking epithelial to mesenchymal transition caused by oxidative stress and hypoxia. International Journal of Molecular Sciences 21: 8577. DOI 10.3390/ijms21228577. [Google Scholar] [CrossRef]
Kawami M, Yumoto R, Takano M (2022). Preventive approach against drug-induced pulmonary fibrosis through the suppression of epithelial-mesenchymal transition. BIOCELL 46: 1861–1865. DOI 10.32604/biocell.2022.019667. [Google Scholar] [CrossRef]
Kim JW, Tchernyshyov I, Semenza GL, Dang CV (2006). HIF-1-mediated expression of pyruvate dehydrogenase kinase: A metabolic switch required for cellular adaptation to hypoxia. Cell Metabolism 3: 177–185. DOI 10.1016/j.cmet.2006.02.002. [Google Scholar] [CrossRef]
Koshikawa N, Hayashi J, Nakagawara A, Takenaga K (2009). Reactive oxygen species-generating mitochondrial DNA mutation up-regulates hypoxia-inducible factor-1alpha gene transcription via phosphatidylinositol 3-kinase-Akt/protein kinase C/histone deacetylase pathway. The Journal of Biological Chemistry 284: 33185–33194. DOI 10.1074/jbc.M109.054221. [Google Scholar] [CrossRef]
Kseibati MO, Sharawy MH, Salem HA (2020). Chrysin mitigates bleomycin-induced pulmonary fibrosis in rats through regulating inflammation, oxidative stress, and hypoxia. International Immunopharmacology 89: 107011. DOI 10.1016/j.intimp.2020.107011. [Google Scholar] [CrossRef]
Kumar A, Vaish M, Karuppagounder SS, Gazaryan I, Cave JW et al. (2021). HIF1α stabilization in hypoxia is not oxidant-initiated. elife 10: e72873. DOI 10.7554/eLife.72873. [Google Scholar] [CrossRef]
Künzi L, Easter M, Hirsch MJ, Krick S (2021). Cystic fibrosis lung disease in the aging population. Frontiers in Pharmacology 12: 601438. DOI 10.3389/fphar.2021.601438. [Google Scholar] [CrossRef]
Li HS, Zhou YN, Li L, Li SF, Long D et al. (2019). HIF-1α protects against oxidative stress by directly targeting mitochondria. Redox Biology 25: 101109. DOI 10.1016/j.redox.2019.101109. [Google Scholar] [CrossRef]
Li M, Qiu YE, Zheng K (2022). The role of baicalin on carbon tetrachloride induced liver fibrosis. BIOCELL 46: 1089–1096. DOI 10.32604/biocell.2022.013432. [Google Scholar] [CrossRef]
Liang X, Potenza DM, Brenna A, Ma Y, Ren Z et al. (2021). Hypoxia induces renal epithelial injury and activates fibrotic signaling through up-regulation of arginase-II. Frontiers in Physiology 12: 773719. DOI 10.3389/fphys.2021.773719. [Google Scholar] [CrossRef]
Liu X, Chen Z (2017). The pathophysiological role of mitochondrial oxidative stress in lung diseases. Journal of Translational Medicine 15: 207. DOI 10.1186/s12967-017-1306-5. [Google Scholar] [CrossRef]
Liu Z, Zhang Y, Youn JY, Zhang Y, Makino A et al. (2022). Flavored and Nicotine-containing E-Cigarettes induce impaired angiogenesis and diabetic wound healing via increased endothelial oxidative stress and reduced NO bioavailability. Antioxidants 11: 904. DOI 10.3390/antiox11050904. [Google Scholar] [CrossRef]
Mansouri N, Willis GR, Fernandez-Gonzalez A, Reis M, Nassiri S et al. (2019). Mesenchymal stromal cell exosomes prevent and revert experimental pulmonary fibrosis through modulation of monocyte phenotypes. Journal of Clinical Investigation Insight 4: e128060. DOI 10.1183/23120541.lungscienceconference-2019.OP03. [Google Scholar] [CrossRef]
McElroy GS, Chandel NS (2017). Mitochondria control acute and chronic responses to hypoxia. Experimental Cell Research 356: 217–222. DOI 10.1016/j.yexcr.2017.03.034. [Google Scholar] [CrossRef]
Merelli A, Repetto M, Lazarowski A, Auzmendi J (2021). Hypoxia, oxidative stress, and inflammation: Three faces of neurodegenerative diseases. Journal of Alzheimer’s Disease 82: S109–S126. DOI 10.3233/JAD-201074. [Google Scholar] [CrossRef]
Mina S, Staerck C, d’Almeida SM, Marot A, Delneste Y et al. (2015). Identification of Scedosporium boydii catalase A1 gene, a reactive oxygen species detoxification factor highly expressed in response to oxidative stress and phagocytic cells. Fungal Biology 119: 1322–1333. DOI 10.1016/j.funbio.2015.09.007. [Google Scholar] [CrossRef]
Moran-Salvador E, Mann J (2017). Epigenetics and liver fibrosis. Cellular and Molecular Gastroenterology and Hepatology 4: 125–134. DOI 10.1016/j.jcmgh.2017.04.007. [Google Scholar] [CrossRef]
Nehra S, Bhardwaj V, Bansal A, Saraswat D (2017). Combinatorial therapy of exercise-preconditioning and nanocurcumin formulation supplementation improves cardiac adaptation under hypobaric hypoxia. Journal of Basic and Clinical Physiology and Pharmacology 28: 443–453. DOI 10.1515/jbcpp-2016-0134. [Google Scholar] [CrossRef]
Otoupalova E, Smith S, Cheng G, Thannickal VJ (2020). Oxidative stress in pulmonary fibrosis. Comprehensive Physiology 10: 509–547. DOI 10.1002/cphy. [Google Scholar] [CrossRef]
Panopoulos A, Harraz M, Engelhardt JF, Zandi E (2005). Iron-mediated H2O2 production as a mechanism for cell type-specific inhibition of tumor necrosis factor α-induced but not interleukin-1β-induced IκB kinase complex/nuclear factor-κB activation. The Journal of Biological Chemistry 280: 2912–2923. DOI 10.1074/jbc.M409524200. [Google Scholar] [CrossRef]
Prabhakar NR, Kumar GK, Nanduri J, Semenza GL (2007). ROS signaling in systemic and cellular responses to chronic intermittent hypoxia. Antioxidants & Redox Signaling 9: 1397–1403. DOI 10.1089/ars.2007.1732. [Google Scholar] [CrossRef]
Quiñonez-Flores CM, González-Chávez SA, Pacheco-Tena C (2016). Hypoxia and its implications in rheumatoid arthritis. Journal of Biomedical Science 23: 62. DOI 10.1186/s12929-016-0281-0. [Google Scholar] [CrossRef]
Remensnyder JP, Majno G (1968). Oxygen gradients in healing wounds. The American Journal of Pathology 52: 301–323. DOI 10.1097/00006534-196810000-00042. [Google Scholar] [CrossRef]
Roehlen N, Crouchet E, Baumert TF (2020). Liver fibrosis: Mechanistic concepts and therapeutic perspectives. Cells 9: 875. DOI 10.3390/cells9040875. [Google Scholar] [CrossRef]
Rosenberger C, Khamaisi M, Abassi Z, Shilo V, Weksler-Zangen S et al. (2008). Adaptation to hypoxia in the diabetic rat kidney. Kidney International 73: 34–42. DOI 10.1038/sj.ki.5002567. [Google Scholar] [CrossRef]
Sada K, Nishikawa T, Kukidome D, Yoshinaga T, Kajihara N et al. (2016). Hyperglycemia induces cellular hypoxia through production of mitochondrial ROS followed by suppression of aquaporin-1. PLoS One 11: e0158619. DOI 10.1371/journal.pone.0158619. [Google Scholar] [CrossRef]
Sasabe E, Yang Z, Ohno S, Yamamoto T (2010). Reactive oxygen species produced by the knockdown of manganese-superoxide dismutase up-regulate hypoxia-inducible factor-1alpha expression in oral squamous cell carcinoma cells. Free Radical Biology & Medicine 48: 1321–1329. DOI 10.1016/j.freeradbiomed.2010.02.013. [Google Scholar] [CrossRef]
Schodel J, Oikonomopoulos S, Ragoussis J, Pugh CW, Ratcliffe PJ et al. (2011). High-resolution genome-wide mapping of HIF-binding sites by ChIP-seq. Blood 117: e207–e217. DOI 10.1182/blood-2010-10-314427. [Google Scholar] [CrossRef]
Sekiguchi A, Motegi SI, Fujiwara C, Yamazaki S, Inoue Y et al. (2019). Inhibitory effect of kaempferol on skin fibrosis in systemic sclerosis by the suppression of oxidative stress. Journal of Dermatological Science 96: 8–17. DOI 10.1016/j.jdermsci.2019.08.004. [Google Scholar] [CrossRef]
Senavirathna LK, Huang C, Yang X, Munteanu MC, Sathiaseelan R et al. (2018). Hypoxia induces pulmonary fibroblast proliferation through NFAT signaling. Scientific Reports 8: 2709. DOI 10.1038/s41598-018-21073-x. [Google Scholar] [CrossRef]
Su H, Wan C, Song A, Qiu Y, Xiong W et al. (2019). Oxidative stress and renal fibrosis: Mechanisms and therapies. Advances in Experimental Medicine and Biology 1165: 585–604. DOI 10.1007/978-981-13-8871-2. [Google Scholar] [CrossRef]
Sun L, Wang X, Saredy J, Yuan Z, Yang X et al. (2020a). Innate-adaptive immunity interplay and redox regulation in immune response. Redox Biology 37: 101759. DOI 10.1016/j.redox.2020.101759. [Google Scholar] [CrossRef]
Sun L, Yang X, Yuan Z, Wang H (2020b). Metabolic reprogramming in immune response and tissue inflammation. Arteriosclerosis, Thrombosis, and Vascular Biology 40: 1990–2001. DOI 10.1161/ATVBAHA.120.314037. [Google Scholar] [CrossRef]
Tzouvelekis A, Harokopos V, Paparountas T, Oikonomou N, Chatziioannou A et al. (2007). Comparative expression profiling in pulmonary fibrosis suggests a role of hypoxia-inducible factor-1alpha in disease pathogenesis. American Journal of Respiratory and Critical Care Medicine 176: 1108–1119. DOI 10.1164/rccm.200705-683OC. [Google Scholar] [CrossRef]
Xie L, Zeng Y (2020). Therapeutic potential of exosomes in pulmonary fibrosis. Frontiers in Pharmacology 11: 590972. DOI 10.3389/fphar.2020.590972. [Google Scholar] [CrossRef]
Xiong M, Zhao Y, Mo H, Yang H, Yue F et al. (2021). Intermittent hypoxia increases ROS/HIF-1α ‘related oxidative stress and inflammation and worsens bleomycin-induced pulmonary fibrosis in adult male C57BL/6J mice. International Immunopharmacology 100: 108165. DOI 10.1016/j.intimp.2021.108165. [Google Scholar] [CrossRef]
Xu X, Tan X, Tampe B, Sanchez E, Zeisberg M et al. (2015). Snail is a direct target of hypoxia-inducible factor 1α (HIF1α). In hypoxia-induced endothelial to mesenchymal transition of human coronary endothelial cells. The Journal of Biological Chemistry 290: 16653–16664. DOI 10.1074/jbc.M115.636944. [Google Scholar] [CrossRef]
Yao X, Lu C, Shen J, Jiang W, Qiu Y et al. (2021). A novel nine gene signature integrates stemness characteristics associated with prognosis in hepatocellular carcinoma. BIOCELL 45: 1425–1448. DOI 10.32604/biocell.2021.017289. [Google Scholar] [CrossRef]
Yue X, Zhao P, Wu K, Huang J, Zhang W et al. (2016). GRIM-19 inhibition induced autophagy through activation of ERK and HIF-1α not STAT3 in Hela cells. Tumour Biology 37: 9789–9796. DOI 10.1007/s13277-016-4877-5. [Google Scholar] [CrossRef]
Zeng Q, Zhou F, Lei L, Chen J, Lu J et al. (2017). Ganoderma lucidum polysaccharides protect fibroblasts against UVB-induced photoaging. Molecular Medicine Reports 15: 111–116. DOI 10.3892/mmr.2016.6026. [Google Scholar] [CrossRef]
Zeng Y, Du X, Yao X, Qiu Y, Jiang W et al. (2022). Mechanism of cell death of endothelial cells regulated by mechanical forces. Journal of Biomechanics 131: 110917. DOI 10.1016/j.jbiomech.2021.110917. [Google Scholar] [CrossRef]
Zhao H, Li C, Li L, Liu J, Gao Y et al. (2020). Baicalin alleviates bleomycin‐induced pulmonary fibrosis and fibroblast proliferation in rats via the PI3K/AKT signaling pathway. Molecular Medicine Reports 21: 2321–2334. DOI 10.3892/mmr.2020.11046. [Google Scholar] [CrossRef]
Cite This Article
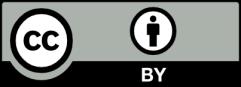