Open Access
ARTICLE
Network pharmacology and molecular docking identify mechanisms of medicinal plant-derived 1,2,3,4,6-penta-O-galloyl-beta-D-glucose treating gastric cancer
1 The First Clinical Medical College, Lanzhou University, Lanzhou, 730000, China
2 Gansu Key Laboratory of Gastroenterology, Lanzhou University, Lanzhou, 730000, China
3 Department of Geriatrics Gerontology, The First Hospital of Lanzhou University, Lanzhou, 730000, China
4 Office of National Drug Clinical Trial Institutions, The First Hospital of Lanzhou University, Lanzhou, 730000, China
5 School of Pharmacy, Lanzhou University, Lanzhou, 730000, China
6 Department of Gastroenterology, The First Hospital of Lanzhou University, Lanzhou, 730000, China
* Corresponding Authors: YUPING WANG. Email: ; YONGNING ZHOU. Email:
BIOCELL 2023, 47(5), 977-989. https://doi.org/10.32604/biocell.2023.028402
Received 16 December 2022; Accepted 17 January 2023; Issue published 10 April 2023
Abstract
Background: 1,2,3,4,6-penta-O-galloyl-beta-D-glucose (PGG) is a natural polyphenolic compound derived from multiple medicinal plants with favorable anticancer activity. Methods: In this study, the mechanisms of PGG against gastric cancer were explored through network pharmacology and molecular docking. First, the targets of PGG were searched in the Herbal Ingredients’ Targets (HIT), Similarity Ensemble Approach (SEA), and Super-PRED databases. The potential targets related to gastric cancer were predicted from the Human Gene Database (GeneCards) and DisGeNET databases. The intersecting targets of PGG and gastric cancer were obtained by Venn diagram and then subjected to protein-protein interaction analysis to screen hub targets. Functional and pathway enrichment of hub targets were analyzed through Gene Ontology and Kyoto Encyclopedia of Genes and Genomes pathway databases. The differential expression and survival analysis of hub targets in gastric cancer were performed based on The Cancer Genome Atlas database. Finally, the affinity of PGG with hub targets was visualized by molecular docking. Results: Three hub targets were screened, including mitogen-activated protein kinase 14 (MAPK14), BCL2 like 1 (BCL2L1), and vascular endothelial growth factor A (VEGFA). MAPK14 had a higher expression, while BCL2L1 and VEGFA had lower expression in gastric cancer than in normal conditions. Enrichment analysis indicated enrichment of these hub targets in MAPK, neurotrophin, programmed death-ligand 1 (PD-L1) checkpoint, phosphatidylinositol 3-kinases/protein kinase B (PI3K-Akt), Ras, and hypoxia-inducible factor-1 (HIF-1) signaling pathways. Conclusion: Therefore, network pharmacology and molecular docking analyses revealed that PGG exerts a therapeutic efficacy on gastric cancer by multiple targets (MAPK14, BCL2L1, and VEGFA) and pathways (MAPK, PD-L1 checkpoint, PI3K-Akt, Ras, and HIF-1 pathways).Keywords
Supplementary Material
Supplementary Material FileGastric cancer is a malignancy, which leads to high motility, and is the third leading cause of cancer-related deaths globally (Ajani et al., 2022). More than 1 million people are newly diagnosed with gastric cancer each year worldwide (Thrift and El-Serag, 2020), and the overall survival for cancer of the stomach remains at 25% (Sexton et al., 2020). In 2020, the new cases of gastric cancer reached approximately 480,000 in China, accounting for nearly 44% of all global new gastric cancer cases (Sung et al., 2021). Risk factors for gastric cancer mainly include Helicobacter pylori infection, age, high salt intake, and diets low in fruit and vegetables (Smyth et al., 2020). The incidence rate of gastric cancer rises progressively with age, and the median age at diagnosis is 70 years (Machlowska et al., 2020). Most of the time, patients with gastric cancer are diagnosed at advanced stages, causing a poor prognosis (Ouyang et al., 2022). Conventional therapies, including chemotherapy and resection, have limited clinical benefit; the median overall survival for patients with advanced-stage gastric cancer is approximately 8 months (Li et al., 2021). The commonly used chemotherapeutic drugs, including fluorouracil, capecitabine, and platinum, exhibit high toxicity, resulting in low overall survival of gastric cancer (Li et al., 2021; Shitara, 2017; Solomon and Garrido-Laguna, 2018). Recently, due to their low toxicity, traditional Chinese herbal medicines have attracted accumulating attention to treat gastric cancer as an adjunctive therapy (Wang et al., 2021; Xu et al., 2022).
1,2,3,4,6-penta-O-galloyl-beta-D-glucose (PGG) is a natural polyphenolic compound derived from multiple medicinal plants, including Galla rhois, Schinus terebinthifolius, Rhus chinensis Mill., Paeonia lactiflora Pall., etc., (Kant et al., 2020). PGG is reportedly extracted from P. lactiflora Pall. Root and G. rhois, which showed favorable anti-inflammatory and antiviral activity (Kim et al., 2020; Lee et al., 2021). PGG possesses various biological and pharmacologic activities, including anti-cancer, anti-oxidative, anti-inflammatory, anti-coagulation, anti-angiogenesis, radio-protective, and neuroprotective properties (Zhang et al., 2009). PGG has shown enormous potential as an anti-cancer agent. For example, PGG confers cytoprotection against oxidative damage in human hepatoma cells (Pae et al., 2006). Oral administration of PGG can repress tumor growth and metastasis of triple-negative breast cancer (Lee et al., 2011). PGG exerts anti-colorectal cancer effects by inducing apoptosis and tumor suppression (Kawk et al., 2018). PGG also exerts anti-proliferative and anti-metastatic effects on colorectal cancer (Yang et al., 2022). Also, PGG might exert its anti-cancer activity through autophagy-mediated senescence (Dong et al., 2014). However, the potential therapeutic efficacy and pharmacological mechanisms of PGG on gastric cancer remain ambiguous.
Network pharmacology is a systemic approach that predicts the interactions between herbal components and targets for the treatment of diseases (Sharma et al., 2022a, 2022b). Through network pharmacology, the potential molecular mechanisms for herbal drugs treating diseases are explored, contributing to the improvement of therapeutic strategies and the success rate of clinical trials (Li and Zhang, 2013). In this study, the underlying action mechanisms of PGG in the treatment of gastric cancer were explored using network pharmacology combined with molecular docking. Network pharmacology was applied to predict hub targets and signaling pathways of PGG for the treatment of gastric cancer. Subsequently, molecular docking was used to validate the interaction between PGG and hub targets. This research lays a solid foundation for the investigation of PGG as an adjuvant therapeutic drug for gastric cancer.
Prediction of 1,2,3,4,6-penta-O-galloyl-beta-D-glucose and gastric cancer-related targets
Pharmacological targets of PGG were retrieved from online tools, including the Herbal Ingredients’ Targets (HIT) platform (v2.0; http://hit2.badd-cao.net/) (Yan et al., 2022), the Similarity ensemble approach (SEA) dataset (https://sea.bkslab.org/) (Keiser et al., 2007), and Super-PRED (https://prediction.charite.de/) (Nickel et al., 2014). The PubChem ID of PGG was searched as 65238 from HIT. Based on its SMILES structure, PGG-related targets were predicted using SEA and Super-PRED databases. Gastric cancer-related targets were searched from the Human Gene Database (GeneCards v3; https://www.genecards.org/) (Safran et al., 2010) and DisGeNET databases (http://www.disgenet.org/) (Piñero et al., 2020) with the key word of “gastric cancer.” Then, the PGG and cancer-related targets were mapped in the VennDiagram package (v1.7.3) (Chen and Boutros, 2011) to obtain overlapping targets. Finally, the interaction network between overlapping targets and PGG was visualized by Cytoscape 3.7.1 software.
Gene Ontology (GO) and Kyoto Encyclopedia of Genes and Genomes (KEGG) enrichment analyses
The clusterProfiler package (v4.4.2) (Yu et al., 2012) was utilized to analyze the GO function and KEGG pathway enrichment of overlapping targets of PGG and gastric cancer. GO functions include biological processes (BP), cellular components (CC), and 41 molecular functions (MF). The correlation between targets and GO/KEGG terms was evaluated by the hypergeometric distribution model as follows:
In this model, N represents the total number of genes, M represents the number of annotated genes in GO/KEGG, n represents the imported target genes, and k represents the number of shared genes. p-value < 0.01 represents the significant correlation between targets and GO/KEGG terms.
Protein-protein interaction (PPI) network
To obtain the hub targets of PGG treating gastric cancer, the overlapping targets were imported into the STRING platform (https://string-db.org/) (Szklarczyk et al., 2021) to construct the PPI network. The protein type was set as Homo sapiens, and the confidence was set to more than 0.4. Results from SRTING were imported into Cytoscape 3.7.1 software for visualization. The top 10 target proteins in this network were ranked by the maximum neighborhood component (MNC), maximal clique centrality (MCC), and edge percolated component (EPC) methods using the cytoHubba plug-in (Chin et al., 2014). VennDiagram package (v1.7.3) (Chen and Boutros, 2011) was used to obtain the intersection targets of MNC, MCC, and EPC analyses, and the intersection targets were considered hub targets.
The transcriptome data of The Cancer Genome Atlas (TCGA)-gastric cancer were downloaded from the UCSC Xena database (https://xenabrowser.net/hub/). Differential expression of hub target genes in normal and tumor samples were analyzed by t-test. The interaction network between differentially expressed genes (DEGs) and KEGG pathways was visualized in Cytoscape 3.7.1. In addition, to evaluate the prognostic effect of DEGs on gastric cancer, multivariate Cox regression analysis was performed to establish a prognostic model and calculate risk scores. From TCGA, patients with gastric cancer were divided into high- and low-risk groups, and survival analysis was carried out for the validation of the prognostic model.
Molecular docking was performed for PGG and hub target proteins. The 3D protein structures of hub targets were downloaded from the RCSB protein data bank (RCSB PDB, http://www.pdb.org/) and ZINC database (https://zinc.docking.org/). The structure of PGG (CID_65238) was downloaded from the PubChem database (https://pubchem.ncbi.nlm.nih.gov/#query=65238). The Autodock Vina (v1.5.6) and PyMOL (v2.3.0) were applied for molecular docking (Seeliger and de Groot, 2010). The binding activity was assessed according to the free bind energy <−5.0 kcal/mol and hydrogen bond formation between the acceptor ligands.
Identification of intersection targets of 1,2,3,4,6-penta-O-galloyl-beta-D-glucose against gastric cancer
Web-accessible databases, including HIT, SEA, and Super-PRED were used to predict the pharmacological targets of PGG. After the depletion of repetitive genes, 223 PGG-related targets were obtained (Suppl. Table S1). Meanwhile, 5627 gastric cancer-associated targets were collected from the GeneCards and DisGeNET databases (Suppl. Table S2). Venn diagram identified 141 intersection targets of PGG against gastric cancer (Fig. 1A). The network of PGG acting on 141 targets is presented in Fig. 1B.
Figure 1: The intersection targets of 1,2,3,4,6-penta-O-galloyl-beta-D-glucose (PGG) against gastric cancer. (A) The intersection targets of PGG and gastric cancer are shown by a Venn diagram. (B) Compound-target network of PGG and 141 intersection targets.
Gene ontology and kyoto encyclopedia of genes and genomes enrichment analyses
The 141 intersection targets of PGG against gastric cancer were subjected to GO and KEGG enrichment analyses. In GO analysis, these targets were enriched in 777 BPs, 26 CCs, and 41 MFs. Results indicated that PGG mainly affected the regulation of body fluid levels (GO-BP), cytoplasmic vesicle lumen (GO-CC), and serine/threonine/tyrosine kinase activity (GO-MF) (Fig. 2A; Table 1). KEGG enrichment analysis showed that these intersection targets were associated with epidermal growth factor receptor (EGFR) tyrosine kinase inhibitor resistance, chemical carcinogenesis-receptor activation, proteoglycans in cancer, neurotrophin, programmed death-ligand 1 (PD-L1) expression and PD-1 checkpoint, hypoxia-inducible factor-1 (HIF-1), mitogen-activated protein kinase (MAPK), sphingolipid, Ras, and calcium-related signaling pathways (Fig. 2B; Table 2).
Figure 2: Gene Ontology (GO) function and the Kyoto Encyclopedia of Genes and Genomes (KEGG) pathway enrichment analyses of 141 intersection targets. (A) Top 15 GO enrichment terms of targets, including biological process (BP), cellular component (CC), and molecular function (MF). (B) Top 15 KEGG enrichment pathways of targets.
Protein-protein interaction network and hub targets for 1,2,3,4,6-penta-O-galloyl-beta-D-glucose against gastric cancer
PPI network for 141 intersection targets was established to identify hub targets of PGG against gastric cancer (Fig. 3A). In order to find hub targets from this PPI network, network scoring methods (MNC, MCC, and EPC) in cytoHubba were used to identify top 10 hub targets and construct sub-networks. In the MNC method, HIF1A, fibroblast growth factor 2 (FGF2), toll-like receptor 4 (TLR4), BCL2-like 1 (BCL2L1), tumor protein p53 (TP53), MAPK1, MAPK14, vascular endothelial growth factor A (VEGFA), sirtuin 1 (SIRT1), and heat shock protein 90 alpha family class A member 1 (HSP90AA1) were top 10 hub targets (Fig. 3B). In the MCC method, top 10 targets were BCL2L1, HSP90AA1, protein tyrosine phosphatase non-receptor type 11 (PTPN11), TP53, MAPK1, MAPK14, VEGFA, insulin like growth factor 1 receptor (IGF1R), signal transducer and activator of transcription 1 (STAT1), and growth factor receptor bound protein 2 (GRB2) (Fig. 3B). In the EPC method, the top 10 targets were MAPK1, MAPK14, VEGFA, SIRT1, HSP90AA1, STAT1, HIF1A, TLR4, BCL2L1, and TP53 (Fig. 3B). We finally obtained six hub targets from Venn diagram displaying the intersection targets from MNC, MCC, and EPC sub-networks: TP53, VEGFA, MAPK1, HSP90AA1, MAPK14, and BCL2L1 (Fig. 3C).
Figure 3: Identification of hub targets for 1,2,3,4,6-penta-O-galloyl-beta-D-glucose (PGG) against gastric cancer. (A) Protein-protein interaction (PPI) network of 141 intersection targets. (B) PPI networks of top 10 hub targets based on the maximum neighborhood component (MNC), maximal clique centrality (MCC), and edge percolated component (EPC) methods. (C) The intersection hub targets based on the MCC, MNC, and EPC methods were screened by a Venn diagram.
Identification of hub targets in gastric cancer
Six hub targets were subjected to UCSC Xena to identify their differential expression in normal and tumor groups based on the TCGA-gastric cancer database. Results showed significantly upregulated levels of VEGFA, HSP90AA1, and BCL2L1 expression in tumor tissues (p < 0.0001). In contrast, MAPK14 expression was lower in tumor tissues than that in normal (p < 0.05; Fig. 4A). According to KEGG enrichment analysis, these four hub targets were mainly enriched in chemical carcinogenesis-receptor activation, EGFR tyrosine kinase inhibitor resistance, proteoglycans in cancer, MAPK, neurotrophin, and PD-L1 expression and PD-1 checkpoint-related signaling pathway (Fig. 4B). Subsequently, a prognostic model of four hub targets in gastric cancer was obtained by multivariate Cox regression analysis. Risk scores were calculated according to the prognostic model as follows: risk score = 2.2643 * MAPK14 −0.4435 * BCL2L1 + 4.5958 * HSP90AA1 + 0.1674 * VEGFA. Patients from the TCGA-gastric cancer database were categorized into high- and low-risk groups. Survival analysis displayed a lower survival probability for the high-risk group than the low-risk group (p = 0.011; Fig. 4C).
Figure 4: Bioinformatic analysis of hub targets in gastric cancer. (A) Differential expression of hub targets (VEGFA, HSP90AA1, BCL2L1, and MAPK14) in gastric cancer based on the Cancer Genome Atlas (TCGA) dataset. (B) The network of four hub targets and corresponding Kyoto Encyclopedia of Genes and Genomes pathways. (C) Survival analysis related to four hub targets in patients with gastric cancer (VEGFA, vascular endothelial growth factor A; HSP90AA1, heat shock protein 90 alpha family class A member 1; BCL2L1, BCL2 like 1; MAPK14, mitogen-activated protein kinase).
Molecular docking of 1,2,3,4,6-penta-O-galloyl-beta-D-glucose with hub targets
theThe interaction between PGG and four hub targets (MAPK14, BCL2L1, HSP90AA1, and VEGFA) was identified by molecular docking. Results showed that PGG could bind to MAPK14, BCL2L1, and VEGFA with the free binding energy of −8.3, −7.6, and −6.7 kcal/mol, respectively (Fig. 5; Table 3).
Figure 5: Molecular docking between 1,2,3,4,6-penta-O-galloyl-beta-D-glucose (PGG) and three hub targets (MAPK14, BCL2L1, and VEGFA).
Gastric cancer is a disease of global concern with poor prognosis and high mortality (Smyth et al., 2020). Chemotherapy is a conventional therapy for advanced gastric cancer; however, the toxicity of chemotherapeutic drugs results in low median survival, often of less than one year (Smyth et al., 2020). Traditional Chinese medicines (TCM) can reportedly potentiate the sensitivity to chemotherapeutic agents and exert the tumor-suppressing effect (Zhang et al., 2020). In China, approximately 70% of patients with cancers were reported to benefit from TCM treatment (Zhang et al., 2020). PGG, a natural polyphenolic compound from various medicinal plants, possesses favorable anti-tumor effects in multiple cancers, such as colorectal, lung, and breast cancers (Kim et al., 2022; Xiang et al., 2019; Yang et al., 2022). However, the potential effects and molecular mechanisms of action of PGG in the treatment of gastric cancer remain to be determined.
In the current study, 141 potential targets of PGG were predicted for treating gastric cancer. These potential targets were analyzed by the PPI network, and six hub targets were obtained by MNC, MCC, and EPC methods. The six identified hub targets were TP53, VEGFA, MAPK1, HSP90AA1, MAPK14, and BCL2L1. TP53 is a tumor suppressor gene that regulates the expression of genes involved in the cell cycle, DNA repair, and metabolic regulation (Donehower et al., 2019). VEGFA is a major regulator of angiogenesis, implicated in tumor growth and metastasis in various cancers (Lu et al., 2020). MAPK pathway is a classic cellular phosphorylation cascade, controlling cell proliferation, differentiation, inflammation, and metabolism in cancers (Jiang et al., 2021). PGG reportedly alleviates inflammation and oxidative stress in diabetic nephropathy rats by inhibiting the MAPK/NF-κB signaling pathways (Wang et al., 2022). HSP90AA1 reportedly acts as a diagnostic marker and therapeutic target for liver metastasis of gastric cancer (Chang et al., 2009). BCL2L1 belongs to the BCL-2 family that regulates cancer cell apoptosis, tumor metastasis, and cell sensitivity to chemotherapeutic drugs (Warren et al., 2019). The inhibition of Bcl-2/Bcl-xl has been a promising treatment strategy for gastric carcinoma by inducing apoptosis (Yi et al., 2020). Summing up the above findings, PGG may inhibit cell proliferation and tumor metastasis of gastric cancer by targeting these hub genes.
Six hub targets were subjected to differential expression analysis in gastric cancer, and we found upregulated expressions of VEGFA, HSP90AA1, and BCL2L1, whereas MAPK14 expression was downregulated in gastric cancer. GO functional analysis showed that PGG treatment against gastric cancer mainly concentrated in response to peptide, cytoplasmic vesicle lumen, and protein serine/threonine/tyrosine kinase activity, positive regulation of MAPK. Molecular docking revealed that PGG has good binding activities with the hub targets MAPK14 (−8.3 kcal/mol), BCL2L1 (−7.6 kcal/mol), and VEGFA (−6.7 kcal/mol). KEGG analysis showed that MAPK14 is mainly enriched in MAPK, neurotrophin, and PD-L1 checkpoint signaling pathways, BCL2L1 is closely related to PI3K-Akt and Ras signaling pathways, and VEGFA is associated with HIF-1 and MAPK signaling pathways. Taken together, PGG may treat gastric cancer by acting on MAPK14, BCL2L1, and VEGFA to regulate MAPK, neurotrophin, PD-L1 checkpoint, PI3K-Akt, Ras, and HIF-1 signaling pathways.
MAPK signaling pathway is an essential ubiquitous signal transduction pathway, responsible for cellular communication and regulates cell functions, including proliferation, differentiation, and survival (Degirmenci et al., 2020). During cancer treatment, the MAPK pathway modulates drug sensitivity and resistance (Lee et al., 2020). In gastric cancer, the inactivation of the MAPK pathway inhibits the malignant biological behavior of cancer cells (Jiang et al., 2021). Previous studies have confirmed that PGG exerts a regulatory effect on the MAPK pathway. For example, PGG suppresses the MAPK/NF-κB signaling pathway to alleviate inflammation and oxidative stress in diabetic nephropathy (Wang et al., 2022). Also, PGG attenuates pro-inflammatory responses of microglial cells through MAPK signaling pathway (Mendonca et al., 2018). MAPK14 is a remember of the MAPK pathway; its inhibition impairs cell proliferation and migration of gastric cancer (Mesquita et al., 2020). MAPK14 could be a potential pharmacological target for gastric cancer. In addition, VEGFA is the most functional isoform of the VEGF family, which is overexpressed in various tumors, including gastric cancer, and correlates with poor prognosis from metastasis (Karaman et al., 2018; Yu et al., 2022). Consistently, we found that VEGF has higher expression in gastric tumor samples than in normal samples. Our prognostic model also showed that high expression of VEGFA has higher risk scores and lower survival probability in patients with gastric cancer. In this study, we found that the MAPK pathway is enriched by MAPK14 and VEGFA. Molecular docking showed that PGG has a good affinity with MAPK4 and VEGFA. Therefore, we speculated that PGG may target MAPK14 and VEGFA to regulate the MAPK pathway, thereby suppressing gastric cancer. VEGFA is also enriched in the HIF-1 pathway, a key signaling regulating tumor hypoxia (Li et al., 2019). Under hypoxia conditions, HIF-1α signaling is activated and regulates cell metabolism, inflammation, and tumorigenesis, which has been a promising therapeutic target for gastric cancer (Li et al., 2019). Of note, PGG reportedly alleviates pancreatic cancer cachexia by inhibiting HIF-1α (Yang et al., 2019a). Thus, PGG may act on VEGFA to regulate the HIF-1 pathway, thereby inhibiting gastric cancer.
Neurotrophins are nerve growth factors expressed in human cancers to trigger neurogenesis and stimulate tumor growth (Griffin et al., 2018). One previous study revealed the carcinogenic role of the brain-derived neurotrophic factor in gastric cancer (Esfandi et al., 2019). In addition, the immune checkpoint PD-1/PD-L1 signaling pathway is a critical target of immunotherapies for human cancers (Ai et al., 2020). PD-L1 represses anti-tumor immunity by binding to its receptor PD-1 and counteracting T cell-activating signals on activated T cells (Sun et al., 2018). Notably, PD-L1 expression positively correlates with tumor lymphocyte infiltration in gastric cancer (Wang et al., 2019). Our study found that PGG-targeted MAPK14 is related to neurotrophin and PD-L1 checkpoint pathways, implying the potential mechanisms of PGG against gastric cancer.
Another hub target BCL2L1 was found to be related to PI3K-Akt and Ras signaling pathways. The PI3K-Akt signaling pathway is an essential intracellular pathway that regulates cell proliferation, differentiation, metabolism, and response to extracellular stimulation (Noorolyai et al., 2019). Due to the key role of PI3K-Akt signaling in cellular processes, this pathway has emerged as a noteworthy target for cancer treatment (Yang et al., 2019b). Previous studies have reported the tumorigenic property of PI3K-Akt in gastric cancer (Rong et al., 2020; Wu et al., 2021). Our study revealed that PGG treating gastric cancer may be achieved by targeting BCL2L1 and regulating the PI3K-Akt signaling pathway. In addition, the Ras signaling pathway also plays a pivotal role in cellular processes, regulating cellular responses to growth factors and affecting cell proliferation, differentiation, migration, and apoptosis (Al Mahi and Ablain, 2022). Ras is the most frequently mutated gene in cancers; consequently, Ras-targeted therapy has been effectively developed for cancers (Moore et al., 2020). The inhibition of the Ras pathway contributes to stalling the development of gastric cancer and enhancing the efficacy of chemotherapy (Liao et al., 2021). Our study developed the potential therapeutic mechanism of PGG against gastric cancer, that is, the regulation of BCL2L1 and the Ras signaling pathway.
In the present study, the pharmacological mechanisms of PGG on gastric cancer were analyzed through network pharmacology and molecular docking. The potential anti-cancer targets and signaling pathways of PGG were predicted. We found that PGG may act on MAPK14, BCL2L1, and VEGFA to regulate MAPK, neurotrophin, PD-L1 checkpoint, PI3K-Akt, Ras, and HIF-1 signaling pathways, thereby restraining gastric cancer progression. However, in vitro and in vivo validation experiments were not included in this study. Therefore, in the follow-up study, we plan to design pharmacological experiments in vitro and in vivo to validate the mechanisms of PGG in treating gastric cancer. The present study provides an important foundation for the therapeutic application of PGG on gastric cancer.
Funding Statement: This research was supported by the Natural Science Foundation of Gansu Province [Grant Numbers 22JR5RA930, 22JR5RA894], the Talent Project of Lanzhou Science and Technology Bureau [Grant Number 2022-3-44], the projects managed by the Administration of Traditional Chinese Medicine [Grant Number GZKG-2022-54], and Intra Hospital Fund of the First Hospital of Lanzhou University [Grant Number ldyyyn2021101].
Author Contributions: The authors confirm contribution to the paper as follows: Study conception and design: Man Ren, Yuan Yang. Data collection: Nannan Zhao. Analysis and interpretation of results: Dan Li. Draft manuscript preparation: Man Ren. Manuscript review and edit: Yuping Wang, Yongning Zhou. Funding acquisition: Man Ren and Dan Li. All authors reviewed the results and approved the final version of the manuscript.
Availability of Data and Materials: The data used to support the findings of this study are included within the article and can be made available upon request to the corresponding author.
Ethics Approval: Not applicable.
Conflicts of Interest: The authors declare that they have no conflicts of interest to report regarding the present study.
Supplementary Materials: The supplementary material is available online at DOI 10.32604/biocell.2023.028402.
References
Ai L, Xu A, Xu J (2020). Roles of PD-1/PD-L1 pathway: Signaling, cancer, and beyond. Advances in Experimental Medicine and Biology 1248: 33–59. https://doi.org/10.1007/978-981-15-3266-5 [Google Scholar] [CrossRef]
Ajani JA, D’Amico TA, Bentrem DJ, Chao J, Cooke D et al. (2022). Gastric cancer, version 2, NCCN clinical practice guidelines in oncology. Journal of the National Comprehensive Cancer Network 20: 167–192. https://doi.org/10.6004/jnccn.2022.0008 [Google Scholar] [PubMed] [CrossRef]
Al Mahi A, Ablain J (2022). RAS pathway regulation in melanoma. Disease Models & Mechanisms 15: dmm049229. https://doi.org/10.1242/dmm.049229 [Google Scholar] [PubMed] [CrossRef]
Chang W, Ma L, Lin L, Gu L, Liu X et al. (2009). Identification of novel hub genes associated with liver metastasis of gastric cancer. International Journal of Cancer 125: 2844–2853. https://doi.org/10.1002/(ISSN)1097-0215 [Google Scholar] [CrossRef]
Chen H, Boutros PC (2011). VennDiagram: A package for the generation of highly-customizable Venn and Euler diagrams in R. BMC Bioinformatics 12: 35. https://doi.org/10.1186/1471-2105-12-35 [Google Scholar] [PubMed] [CrossRef]
Chin CH, Chen SH, Wu HH, Ho CW, Ko MT, Lin CY (2014). cytoHubba: Identifying hub objects and sub-networks from complex interactome. BMC Systems Biology 8: S11. https://doi.org/10.1186/1752-0509-8-S4-S11 [Google Scholar] [PubMed] [CrossRef]
Degirmenci U, Wang M, Hu J (2020). Targeting aberrant RAS/RAF/MEK/ERK signaling for cancer therapy. Cells 9: 198. https://doi.org/10.3390/cells9010198 [Google Scholar] [PubMed] [CrossRef]
Donehower LA, Soussi T, Korkut A, Liu Y, Schultz A et al. (2019). Integrated analysis of TP53 gene and pathway alterations in the cancer genome atlas. Cell Reports 28: 1370–1384.e1375. https://doi.org/10.1016/j.celrep.2019.07.001 [Google Scholar] [PubMed] [CrossRef]
Dong Y, Yin S, Jiang C, Luo X, Guo X et al. (2014). Involvement of autophagy induction in penta-1,2,3,4,6-O-galloyl-β-D-glucose-induced senescence-like growth arrest in human cancer cells. Autophagy 10: 296–310. https://doi.org/10.4161/auto.27210 [Google Scholar] [PubMed] [CrossRef]
Esfandi F, Bouraghi H, Glassy MC, Taheri M, Kahaei MS, Kholghi Oskooei V, Ghafouri-Fard S (2019). Brain-derived neurotrophic factor downregulation in gastric cancer. Journal of Cellular Biochemistry 120: 17831–17837. https://doi.org/10.1002/jcb.29050 [Google Scholar] [PubMed] [CrossRef]
Griffin N, Faulkner S, Jobling P, Hondermarck H (2018). Targeting neurotrophin signaling in cancer: The renaissance. Pharmacological Research 135: 12–17. https://doi.org/10.1016/j.phrs.2018.07.019 [Google Scholar] [PubMed] [CrossRef]
Jiang T, Xia Y, Lv J, Li B, Li Y et al. (2021). A novel protein encoded by circMAPK1 inhibits progression of gastric cancer by suppressing activation of MAPK signaling. Molecular Cancer 20: 66. https://doi.org/10.1186/s12943-021-01358-y [Google Scholar] [PubMed] [CrossRef]
Kant R, Lu CK, Nguyen HM, Hsiao HH, Chen CJ, Hsiao HP, Lin KJ, Fang CC, Yen CH (2020). 1,2,3,4,6 penta-O-galloyl-β-D-glucose ameliorates high-fat diet-induced nonalcoholic fatty liver disease and maintains the expression of genes involved in lipid homeostasis in mice. Biomedicine & Pharmacotherapy 129: 110348. https://doi.org/10.1016/j.biopha.2020.110348 [Google Scholar] [PubMed] [CrossRef]
Karaman S, Leppänen VM, Alitalo K (2018). Vascular endothelial growth factor signaling in development and disease. Development 145: dev151019. https://doi.org/10.1242/dev.151019 [Google Scholar] [PubMed] [CrossRef]
Kawk SH, Kang YR, Kim YH (2018). 1,2,3,4,6-Penta-O-galloyl-β-d-glucose suppresses colon cancer through induction of tumor suppressor. Bioorganic & Medicinal Chemistry Letters 28: 2117–2123. https://doi.org/10.1016/j.bmcl.2018.05.028 [Google Scholar] [PubMed] [CrossRef]
Keiser MJ, Roth BL, Armbruster BN, Ernsberger P, Irwin JJ, Shoichet BK (2007). Relating protein pharmacology by ligand chemistry. Nature Biotechnology 25: 197–206. https://doi.org/10.1038/nbt1284 [Google Scholar] [PubMed] [CrossRef]
Kim JH, Im E, Lee J, Lee HJ, Sim DY et al. (2022). Apoptotic and DNA damage effect of 1,2,3,4,6-Penta-O-galloyl-beta-D-glucose in cisplatin-resistant non-small lung cancer cells via phosphorylation of H(2)AX, CHK2 and p53. Cells 11: 1343. https://doi.org/10.3390/cells11081343 [Google Scholar] [PubMed] [CrossRef]
Kim KH, Shim JS, Kim HJ, Son ED (2020). Penta-O-galloyl-β-D-glucose from Paeonia lactiflora Pall. root extract enhances the expression of skin barrier genes via EGR3. Journal of Ethnopharmacology 248: 112337. https://doi.org/10.1016/j.jep.2019.112337 [Google Scholar] [PubMed] [CrossRef]
Lee HJ, Seo NJ, Jeong SJ, Park Y, Jung DB et al. (2011). Oral administration of penta-O-galloyl-β-D-glucose suppresses triple-negative breast cancer xenograft growth and metastasis in strong association with JAK1-STAT3 inhibition. Carcinogenesis 32: 804–811. https://doi.org/10.1093/carcin/bgr015 [Google Scholar] [PubMed] [CrossRef]
Lee S, Rauch J, Kolch W (2020). Targeting MAPK signaling in cancer: Mechanisms of drug resistance and sensitivity. International Journal of Molecular Sciences 21: 1102. https://doi.org/10.3390/ijms21031102 [Google Scholar] [PubMed] [CrossRef]
Lee YG, Kang KW, Hong W, Kim YH, Oh JT et al. (2021). Potent antiviral activity of Agrimonia pilosa, Galla rhois, and their components against SARS-CoV-2. Bioorganic & Medicinal Chemistry 45: 116329. https://doi.org/10.1016/j.bmc.2021.116329 [Google Scholar] [PubMed] [CrossRef]
Li H, Jia Y, Wang Y (2019). Targeting HIF-1α signaling pathway for gastric cancer treatment. Pharmazie 74: 3–7. https://doi.org/10.1691/ph.2019.8674 [Google Scholar] [PubMed] [CrossRef]
Li K, Zhang A, Li X, Zhang H, Zhao L (2021). Advances in clinical immunotherapy for gastric cancer. Biochimica et Biophysica Acta-Reviews on Cancer 1876: 188615. https://doi.org/10.1016/j.bbcan.2021.188615 [Google Scholar] [PubMed] [CrossRef]
Li S, Zhang B (2013). Traditional Chinese medicine network pharmacology: Theory, methodology and application. Chinese Journal of Natural Medicines 11: 110–120. https://doi.org/10.1016/S1875-5364(13)60037-0 [Google Scholar] [PubMed] [CrossRef]
Liao Y, Chen W, Shi W, Zha H (2021). Targeting cPLA2α inhibits gastric cancer and augments chemotherapy efficacy via suppressing Ras/MEK/ERK and Akt/β-catenin pathways. Cancer Chemotherapy and Pharmacology 88: 689–697. https://doi.org/10.1007/s00280-021-04322-1 [Google Scholar] [PubMed] [CrossRef]
Lu J, Wang YH, Yoon C, Huang XY, Xu Y et al. (2020). Circular RNA circ-RanGAP1 regulates VEGFA expression by targeting miR-877-3p to facilitate gastric cancer invasion and metastasis. Cancer Letters 471: 38–48. https://doi.org/10.1016/j.canlet.2019.11.038 [Google Scholar] [PubMed] [CrossRef]
Machlowska J, Baj J, Sitarz M, Maciejewski R, Sitarz R (2020). Gastric cancer: Epidemiology, risk factors, classification, genomic characteristics and treatment strategies. International Journal of Molecular Sciences 21: 4012. https://doi.org/10.3390/ijms21114012 [Google Scholar] [PubMed] [CrossRef]
Mendonca P, Taka E, Bauer D, Reams RR, Soliman KFA (2018). The attenuating effects of 1,2,3,4,6 penta-O-galloyl-β-d-glucose on pro-inflammatory responses of LPS/IFNγ-activated BV-2 microglial cells through NF-κB and MAPK signaling pathways. Journal of Neuroimmunology 324: 43–53. https://doi.org/10.1016/j.jneuroim.2018.09.004 [Google Scholar] [PubMed] [CrossRef]
Mesquita FP, Moreira-Nunes CA, da Silva EL, Lima LB, Daniel JP, Zuerker WJ, Brayner M, de Moraes MEA, Montenegro RC (2020). MAPK14 (p38α) inhibition effects against metastatic gastric cancer cells: A potential biomarker and pharmacological target. Toxicology in Vitro 66: 104839. https://doi.org/10.1016/j.tiv.2020.104839 [Google Scholar] [PubMed] [CrossRef]
Moore AR, Rosenberg SC, McCormick F, Malek S (2020). RAS-targeted therapies: Is the undruggable drugged? Nature Reviews Drug Discovery 19: 533–552. https://doi.org/10.1038/s41573-020-0068-6 [Google Scholar] [PubMed] [CrossRef]
Nickel J, Gohlke BO, Erehman J, Banerjee P, Rong WW, Goede A, Dunkel M, Preissner R (2014). SuperPred: Update on drug classification and target prediction. Nucleic Acids Research 42: W26–W31. https://doi.org/10.1093/nar/gku477 [Google Scholar] [PubMed] [CrossRef]
Noorolyai S, Shajari N, Baghbani E, Sadreddini S, Baradaran B (2019). The relation between PI3K/AKT signalling pathway and cancer. Gene 698: 120–128. https://doi.org/10.1016/j.gene.2019.02.076 [Google Scholar] [PubMed] [CrossRef]
Ouyang S, Li H, Lou L, Huang Q, Zhang Z et al. (2022). Inhibition of STAT3-ferroptosis negative regulatory axis suppresses tumor growth and alleviates chemoresistance in gastric cancer. Redox Biology 52: 102317. https://doi.org/10.1016/j.redox.2022.102317 [Google Scholar] [PubMed] [CrossRef]
Pae HO, Oh GS, Jeong SO, Jeong GS, Lee BS, Choi BM, Lee HS, Chung HT (2006). 1,2,3,4,6-penta-O-galloyl-beta-D-glucose up-regulates heme oxygenase-1 expression by stimulating Nrf2 nuclear translocation in an extracellular signal-regulated kinase-dependent manner in HepG2 cells. World Journal of Gastroenterology 12: 214–221. https://doi.org/10.3748/wjg.v12.i2.214 [Google Scholar] [PubMed] [CrossRef]
Piñero J, Ramírez-Anguita JM, Saüch-Pitarch J, Ronzano F, Centeno E, Sanz F, Furlong LI (2020). The DisGeNET knowledge platform for disease genomics: 2019 update. Nucleic Acids Research 48: D845–D855. https://doi.org/10.1093/nar/gkz1021 [Google Scholar] [PubMed] [CrossRef]
Rong L, Li Z, Leng X, Li H, Ma Y, Chen Y, Song F (2020). Salidroside induces apoptosis and protective autophagy in human gastric cancer AGS cells through the PI3K/Akt/mTOR pathway. Biomedicine & Pharmacotherapy 122: 109726. https://doi.org/10.1016/j.biopha.2019.109726 [Google Scholar] [PubMed] [CrossRef]
Safran M, Dalah I, Alexander J, Rosen N, Iny Stein T et al. (2010). GeneCards version 3: The human gene integrator. Database, 2010: baq020. https://doi.org/10.1093/database/baq020 [Google Scholar] [PubMed] [CrossRef]
Seeliger D, de Groot BL (2010). Ligand docking and binding site analysis with PyMOL and Autodock/Vina. Journal of Computer-Aided Molecular Design 24: 417–422. https://doi.org/10.1007/s10822-010-9352-6 [Google Scholar] [PubMed] [CrossRef]
Sexton RE, Al Hallak MN, Diab M, Azmi AS (2020). Gastric cancer: A comprehensive review of current and future treatment strategies. Cancer Metastasis Reviews 39: 1179–1203. https://doi.org/10.1007/s10555-020-09925-3 [Google Scholar] [PubMed] [CrossRef]
Sharma R, Jadhav M, Choudhary N, Kumar A, Rauf A et al. (2022a). Deciphering the impact and mechanism of Trikatu, a spices-based formulation on alcoholic liver disease employing network pharmacology analysis and in vivo validation. Frontiers in Nutrition 9: 1063118. https://doi.org/10.3389/fnut.2022.1063118 [Google Scholar] [PubMed] [CrossRef]
Sharma R, Singla RK, Banerjee S, Sinha B, Shen B, Sharma R (2022b). Role of Shankhpushpi (Convolvulus pluricaulis) in neurological disorders: An umbrella review covering evidence from ethnopharmacology to clinical studies. Neuroscience and Biobehavioral Reviews 140: 104795. https://doi.org/10.1016/j.neubiorev.2022.104795 [Google Scholar] [PubMed] [CrossRef]
Shitara K (2017). Chemotherapy for advanced gastric cancer: Future perspective in Japan. Gastric Cancer 20: 102–110. https://doi.org/10.1007/s10120-016-0648-7 [Google Scholar] [PubMed] [CrossRef]
Smyth EC, Nilsson M, Grabsch HI, van Grieken NC, Lordick F (2020). Gastric cancer. Lancet 396: 635–648. https://doi.org/10.1016/S0140-6736(20)31288-5 [Google Scholar] [PubMed] [CrossRef]
Solomon BL, Garrido-Laguna I (2018). Upper gastrointestinal malignancies in 2017: Current perspectives and future approaches. Future Oncology 14: 947–962. https://doi.org/10.2217/fon-2017-0597 [Google Scholar] [PubMed] [CrossRef]
Sun C, Mezzadra R, Schumacher TN (2018). Regulation and function of the PD-L1 checkpoint. Immunity 48: 434–452. https://doi.org/10.1016/j.immuni.2018.03.014 [Google Scholar] [PubMed] [CrossRef]
Sung H, Ferlay J, Siegel RL, Laversanne M, Soerjomataram I, Jemal A, Bray F (2021). Global cancer statistics 2020: GLOBOCAN estimates of incidence and mortality worldwide for 36 cancers in 185 countries. CA: A Cancer Journal for Clinicians 71: 209–249. https://doi.org/10.3322/caac.21660 [Google Scholar] [PubMed] [CrossRef]
Szklarczyk D, Gable AL, Nastou KC, Lyon D, Kirsch R et al. (2021). The STRING database in 2021: Customizable protein-protein networks, and functional characterization of user-uploaded gene/measurement sets. Nucleic Acids Research 49: D605–D612. https://doi.org/10.1093/nar/gkaa1074 [Google Scholar] [PubMed] [CrossRef]
Thrift AP, El-Serag HB (2020). Burden of gastric cancer. Clinical Gastroenterology and Hepatology 18: 534–542. https://doi.org/10.1016/j.cgh.2019.07.045 [Google Scholar] [PubMed] [CrossRef]
Wang D, Li Y, Dai L, Wang Y, Zhao C, Wang W, Zhang Y, Zhao Y, Yu T (2022). 1,2,3,4,6‐penta‐O‐galloyl‐β‐D‐glucose alleviates inflammation and oxidative stress in diabetic nephropathy rats through MAPK/NF‐κB and ERK/Nrf2/HO‐1 signaling pathways. Experimental and Therapeutic Medicine 24: 639. https://doi.org/10.3892/etm.2022.11576 [Google Scholar] [PubMed] [CrossRef]
Wang K, Chen Q, Shao Y, Yin S, Liu C, Liu Y, Wang R, Wang T, Qiu Y, Yu H (2021). Anticancer activities of TCM and their active components against tumor metastasis. Biomedicine & Pharmacotherapy 133: 111044. https://doi.org/10.1016/j.biopha.2020.111044 [Google Scholar] [PubMed] [CrossRef]
Wang X, Wu W.K. K, Gao J, Li Z, Dong B et al. (2019). Autophagy inhibition enhances PD-L1 expression in gastric cancer. Journal of Experimental & Clinical Cancer Research 38: 140. https://doi.org/10.1186/s13046-019-1148-5 [Google Scholar] [PubMed] [CrossRef]
Warren CFA, Wong-Brown MW, Bowden NA (2019). BCL-2 family isoforms in apoptosis and cancer. Cell Death & Disease 10: 177. https://doi.org/10.1038/s41419-019-1407-6 [Google Scholar] [PubMed] [CrossRef]
Wu S, Chen M, Huang J, Zhang F, Lv Z et al. (2021). ORAI2 promotes gastric cancer tumorigenicity and metastasis through PI3K/Akt signaling and MAPK-dependent focal adhesion disassembly. Cancer Research 81: 986–1000. https://doi.org/10.1158/0008-5472.CAN-20-0049 [Google Scholar] [PubMed] [CrossRef]
Xiang Q, Tang J, Luo Q, Xue J, Tao Y, Jiang H, Tian J, Fan C (2019). In vitro study of anti-ER positive breast cancer effect and mechanism of 1,2,3,4-6-pentyl-O-galloyl-beta-d-glucose (PGG). Biomedicine & Pharmacotherapy 111: 813–820. https://doi.org/10.1016/j.biopha.2018.12.062 [Google Scholar] [PubMed] [CrossRef]
Xu W, Li B, Xu M, Yang T, Hao X (2022). Traditional Chinese medicine for precancerous lesions of gastric cancer: A review. Biomedicine & Pharmacotherapy 146: 112542. https://doi.org/10.1016/j.biopha.2021.112542 [Google Scholar] [PubMed] [CrossRef]
Yan D, Zheng G, Wang C, Chen Z, Mao T et al. (2022). HIT 2.0: An enhanced platform for herbal ingredients’ targets. Nucleic Acids Research 50: D1238–D1243. https://doi.org/10.1093/nar/gkab1011 [Google Scholar] [PubMed] [CrossRef]
Yang H, Yue GG, Leung PC, Wong CK, Zhang YJ, Lau CB (2022). Anti-metastatic effects of 1,2,3,4,6-Penta-O-galloyl-β-D-glucose in colorectal cancer: Regulation of cathepsin B-mediated extracellular matrix dynamics and epithelial-to-mesenchymal transition. Pharmacological Research 184: 106457. https://doi.org/10.1016/j.phrs.2022.106457 [Google Scholar] [PubMed] [CrossRef]
Yang J, Wang F, Chen X, Qiu S, Cui L, Hu L (2019a). β-Pentagalloyl-glucose sabotages pancreatic cancer cells and ameliorates cachexia in tumor-bearing mice. The American Journal of Chinese Medicine 47: 675–689. https://doi.org/10.1142/S0192415X19500356 [Google Scholar] [PubMed] [CrossRef]
Yang Q, Jiang W, Hou P (2019b). Emerging role of PI3K/AKT in tumor-related epigenetic regulation. Seminars in Cancer Biology 59: 112–124. https://doi.org/10.1016/j.semcancer.2019.04.001 [Google Scholar] [PubMed] [CrossRef]
Yi H, Qiu MZ, Yuan L, Luo Q, Pan W, Zhou S, Zhang L, Yan X, Yang DJ (2020). Bcl-2/Bcl-xl inhibitor APG-1252-M1 is a promising therapeutic strategy for gastric carcinoma. Cancer Medicine 9: 4197–4206. https://doi.org/10.1002/cam4.3090 [Google Scholar] [PubMed] [CrossRef]
Yu B, Zhu N, Fan Z, Li J, Kang Y, Liu B (2022). miR-29c inhibits metastasis of gastric cancer cells by targeting VEGFA. Journal of Cancer 13: 3566–3574. https://doi.org/10.7150/jca.77727 [Google Scholar] [PubMed] [CrossRef]
Yu G, Wang LG, Han Y, He QY (2012). clusterProfiler: An R package for comparing biological themes among gene clusters. Omics: A Journal of Integrative Biology 16: 284–287. https://doi.org/10.1089/omi.2011.0118 [Google Scholar] [PubMed] [CrossRef]
Zhang J, Li L, Kim SH, Hagerman AE, Lü J (2009). Anti-cancer, anti-diabetic and other pharmacologic and biological activities of penta-galloyl-glucose. Pharmaceutical Research 26: 2066–2080. https://doi.org/10.1007/s11095-009-9932-0 [Google Scholar] [PubMed] [CrossRef]
Zhang Y, Lou Y, Wang J, Yu C, Shen W (2020). Research status and molecular mechanism of the traditional chinese medicine and antitumor therapy combined strategy based on tumor microenvironment. Frontiers in Immunology 11: 609705. https://doi.org/10.3389/fimmu.2020.609705 [Google Scholar] [PubMed] [CrossRef]
Supplementary Materials
TABLE S1: The 223 targets related to 1,2,3,4,6-penta-O-galloyl-beta-D-glucose
TABLE S2: The 5627 gastric cancer-associated targets were collected from the GeneCards and DisGeNET databases
Cite This Article
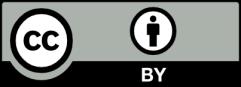