Open Access
ARTICLE
Enhancing Hydrocarbon-Rich Bio-Oil Production via Catalytic Pyrolysis Fortified with Microorganism Pretreatment
1
Key Laboratory of Energy Thermal Conversion and Control of Ministry of Education, Southeast University, Nanjing, 210096, China
2
School of Energy and Power Engineering, Jiangsu University, Zhenjiang, 212013, China
* Corresponding Author: Bo Zhang. Email:
(This article belongs to the Special Issue: New Trends in Bio-oil Production, Conversion and Utilization )
Journal of Renewable Materials 2023, 11(10), 3595-3612. https://doi.org/10.32604/jrm.2023.030005
Received 18 March 2023; Accepted 30 May 2023; Issue published 10 August 2023
Abstract
A new method of pretreatment of corn straw with Phanerochaete chrysosporium combined with pyrolysis was proposed to improve the quality of bio-oil. The characterization results demonstrated that microbial pretreatment was an effective method to decrease the lignin, which can achieve a maximum removal rate of 44.19%. Due to the destruction of biomass structure, the content of alkali metal and alkaline earth metal is reduced. Meanwhile, the depolymerized biomass structure created better pyrolysis conditions to promote the pyrolysis efficiency, increase the average decomposition rate of pyrolysis and reduce the residue. In fast pyrolysis, because of the enrichment of cellulose and the removal of lignin, the contents of acids, linear carbonyls, furans, and sugars increased while the contents of phenols decreased. As for the catalytic pyrolysis, due to the hydrocarbon pool reaction and shape selection deoxidation of ZSM-5 catalyst, the total hydrocarbon and aromatics contents can significantly increase up to 34.37% and 30.59%, respectively, with 3 weeks of pretreatment. And the the low molecular content of bio-oil increased after pretreatment, which can significantly benefit the entry of primary pyrolysis steam into the catalyst pores to improve the catalytic efficiency and hydrocarbon contents. This method provides a new treatment idea for high-quality utilization of biomass.Graphical Abstract
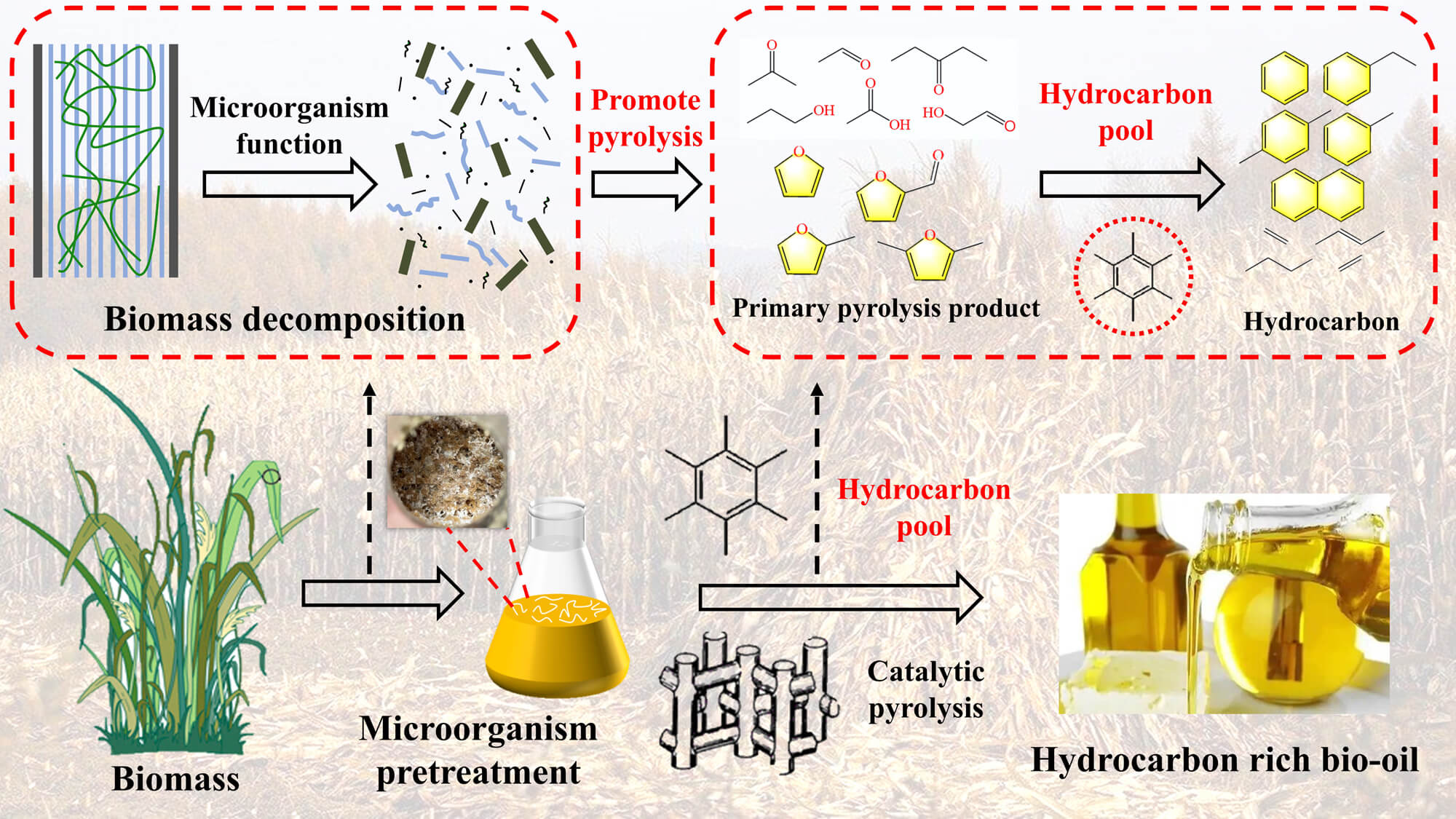
Keywords
Developing sustainable energy is one of the great important ways to alleviate the global greenhouse effect and energy crisis. As the fourth largest energy in the world, biomass, and its derivatives, renewable energies will inevitably replace fossil hydrocarbons soon to promote carbon neutrality and develop advanced technologies [1]. Among many biomass utilization technologies, pyrolysis is a valuable technology converting biomass into bio-oil, gas, and biochar, which has the advantages of fast processing time, low cost, and good reduction effect [2,3]. However, several problems of biomass limit its use: (1) Biomass, composed of cellulose, hemicellulose, and lignin, is complex and cannot enrich a single component, which is difficult to effectively turn into the required chemical [4]. (2) The chemical bonds between biomass components form a dense polymerization structure, like a “barrier”, especially the lignin structure, which hinders the decomposition of biomass from making it difficult to be fully utilized [5].
Pretreatment is an excellent method to solve the above problems. Currently, biomass pretreatment technologies include microorganism, Fenton, and torrefaction pretreatment, which have been proven to realize biomass depolymerization and optimization of biomass products [6–9].
Titiri et al. [10] pretreated spent coffee grounds with NaOH (0.06% w/v) at 99.5°C for 60 min, which can achieve 35.7% lignin removal and promote fermentation. Wu et al. [4] pretreated corncob by Fenton pretreatment with 14 mL/g H2O2 and 16 mM FeSO4 at 55°C for 12 h. After pretreatment, compared to the feedstock, the lignin removal was 38.91%, and the pyrolysis products of levoglucosan yield can increase to more than 70 mg/L. However, the large number of agents and the extreme pretreatment conditions (such as high temperatures) make chemical pretreatment to biomass inappropriate, resulting the high cost and operation difficulty. Moreover, the recovery and disposal of chemical agents after the pretreatment is also difficult. So, it is necessary to find a suitable technology to pretreat biomass.
Microbial pretreatment has attracted extensive attention due to its advantages, such as environmental friendliness, low cost, and mild pretreatment condition [11,12]. He et al. [13] pretreated biomass with the mixed bacteria of Phanerochaete chrysosporium and Trichoderma longibrachiatum. The results showed that microbial inoculation promoted the decomposition of organic matter, and the degradation of cellulose, hemicellulose, and lignin increased by more than 5%, improving fermentation quality. García-Torreiro et al. [14] used white-rot fungus Irpex lacteus to pretreat four agricultural residues within 21 days (corn stover, barley straw, corncob, and wheat straw), which can achieve the best lignin removal (44.20%) and the lowest sugars consumption (11.5%) in corn straw. And Xu et al. [15] used white rot fungus Irpex lacteus CD2 to pretreated corn straw for 21 days. They found that microorganisms can effectively decompose the biomass, whose lignin removal rate reached 25.40%. Meanwhile, the subsequent saccharification was significantly enhanced, reaching 66.40%. In short, microbial pretreatment can improve the quality of biomass, especially the degradation of lignin and the optimization of the subsequent production process. In many microorganisms, Phanerochaete chrysosporium, as a typical white rot fungus, has a positive significance in biomass pretreatment with strong decomposition ability. Phanerochaete chrysosporium can produce cellulose and hemicellulose hydrolase, and lignin peroxidase, which can effectively pretreat biomass [16,17]. When lignin peroxidase decomposes the dense lignin structure on the biomass surface, cellulose and hemicellulose hydrolase can further depolymerize the internal structure of biomass, which can facilitate subsequent utilization.
However, the current microbial pretreatment researches, especially for Phanerochaete chrysosporium, focused on the depolymerization of biomass structure and the improvement of fermentation efficiency, which was fewer applications in pyrolysis, especially the hydrocarbon contents of catalytic pyrolysis. After microorganisms pretreat, the composition of biomass will be optimized and its structure will be destroyed, which can promote the pyrolysis reaction and increase the yield of pyrolysis products. Based on this, we hope that, on the one hand, this technology can provide relevant theoretical data supplement for biomass pretreatment by Phanerochaete chrysosporium. And on the other hand, providing a new processing idea for microbial pretreatment combined with fast catalytic pyrolysis. This paper proposed a new way to use Phanerochaete chrysosporium to pretreat corn straw coupled with catalytic pyrolysis to produce high-quality bio-oil.
In this paper, the changes in biomass properties before and after microbial pretreatment were analyzed by SEM (Scanning Electron Microscopy), XPS (X-ray photoelectron spectroscopy), and other characterization analyses to clarify the role of Phanerochaete chrysosporium in biomass pretreatment. Additionally, fast and catalytic pyrolysis were adopted to analyze the changes in bio-oil composition after pretreatment, and the changes in hydrocarbon content after catalytic pyrolysis are mainly discussed. Moreover, the feasible route for high-value utilization of biomass by Phanerochaete chrysosporium was discussed. By summing up the above studies, this paper provided a green pretreatment technology for biomass utilization.
Corn straw was collected in Lianyungang (China) and oven dried at 90°C to constant weight, and finally grinded into ≤0.425 mm. Phanerochaete chrysosporium SHBCC D22643 was purchased from Shanghai Bioresource Collection Center (China). The pyrolysis catalyst (ZSM-5) was purchased from the Catalyst Plant of Nankai University (China) with a Si/Al ratio of 38.
Phanerochaete chrysosporium was inoculated into 20 ml of Potato Dextrose Agar (PDA) and cultured at 28°C for 9 days. After culturing, the media was washed with sterile water to prepare 50 ml of a fungal spore. 20 g corn straw and 80 ml deionized water were put into a conical flask and autoclaved at 120°C for 20 min to sterilize, then inoculated with 10 ml fungal spores for pretreatment. After pretreatment, corn straw was washed and dry at 90°C for 24 h to collect. In the experiment, the pretreatment periods were 1, 2, and 3 weeks. The corn straw without pretreatment was named CS, whereas others were labeled with their pretreatment period. For example, the corn straw with 1-week pretreatment was labeled as CS-1.
2.3 Characterization Analysis of Raw and Pretreated Samples
The proportion of the three-components of the sample was measured by the paradigm washing method. The XRD (X-Ray Diffraction) (XRD-6100, Shimadzu, Japan) was used to test sample form 5°–90° by a step size 0.03°. And then cellulose crystallinity was calculated by the Segal method [18], as shown in Eq. (1).
where CI is the cellulose crystallinity (%), I002 reflects the intensity of diffraction peak (002) and Iam denotes the intensity of the non-crystalline background, which are located near 2θ = 22° and 18°, respectively.
The surface of biomass before and after pretreatment was analyzed by SEM (Regulus8100, Hitachi, Japan). XPS (Nexsa, Thermo Fisher, USA) and FTIR (Fourier Transform Infrared Spectroscopy) (IS20-IN5, Thermo Fisher, USA) were used to analyze the functional group changes of feedstocks before and after pretreatment. The FTIR is conducted at 0.5 cm−1 with resolutions over 20 scans from 500 to 4000 cm−1. The samples were digested by the solution which was prepared by HCI, HNO3, and HF (the ratio was 6:2:2). And then the metal contents of raw biomass and pretreated feedstocks were analyzed by an atomic absorption spectrometer (AAS, WA 2081, China). The content of AAEMs (alkali and alkaline earth metals) was calculated by Eq. (2).
TG (Thermogravimetric analyzer) (STA-8000, PerkinElmer, USA) was used to the test thermodynamic properties of samples at the heating rates of 10°C/min from 30°C to 800°C. The pyrolysis products of samples were tested by the Py-GC/MS (Pyrolysis Gas Chromatography-Mass Spectrometer) (Agilent, WA2081, USA) at 550°C for 60 s. Other detailed Py-GC/MS parameters can be seen from previous research work [19].
3.1 The Changes in Yield, Three-Composition, and Cellulose Crystallinity of the Raw and Pretreated Feedstock
The change of biomass yield, three components, and cellulose crystallinity are shown in Fig. 1. After microbial pretreatment, the yields of all pretreated samples were less than 70% compared with the original sample, which indicated that the biomass components were depolymerized by microorganism to reduce their weight. Under the 3 weeks pretreatment time, the yield of the sample reached the minimum value (63.55%), which illustrated that with the increase of pretreatment time, the structure of corn straw can be further depolymerized. By determining three components of biomass, it was found that hemicellulose and lignin of corn straw decreased with the increase of pretreatment time. At the 3 weeks pretreatment time, they reached the lowest value, 16.92%, and 6.44%, respectively. By calculating the lignin removal and comparing it with other researches (Table S1), it was found that the lignin removal in this paper reached 44.19%. Among various pretreatment methods, the lignin removal efficiency in this paper was excellent, which indicated that it was feasible to pretreat biomass with Phanerochaete chrysosporium to realize the depolymerization of lignin and structural destruction of biomass.
Figure 1: The yield and compositions analyses of raw and pretreated feedstocks
Since the cellulose in the amorphous zone and hemicellulose were more vulnerable to degrade than cellulose in the crystalline region, the cellulose decomposed incompletely in the first week, resulting in the content of cellulose first increased in the first week and then decreased [20,21]. Therefore, in the sample of CS-1, cellulose content slightly increased, reaching 52.68%, which was about 1.45% higher than the CS (51.23%). With the increase of pretreatment time, microorganism produced a large amount of enzyme substances, which decomposed the dense structure of the surface of biomass and promoted the depolymerization of biomass structure, reducing cellulose content. The content of cellulose reached 45.33% in the CS-3. To confirm this opinion, the cellulose crystallinity of the sample was tested by XRD. The XRD spectrum can see supplementary material (Fig. S1). The cellulose crystallinity of CS was 48.97%. Under the first week of pretreatment, the cellulose crystallinity increased to 56.08%. Then, with the increase of pretreatment time, the cellulose crystallinity gradually decreased, which can reach 35.11% on the CS-3. The cellulose crystallinity is defined as the ratio of crystalline cellulose to the sum of crystalline cellulose and amorphous cellulose [21], implying that the microorganism treats amorphized cellulose before degrading microcrystalline cellulose during the pretreatment process [17]. To clarify the changing trend of cellulose content in the three components, the ratio of cellulose to the sum of the three components was calculated. The calculated results showed that the ratios of cellulose were 57.80% (CS), 63.92% (CS-1), 64.66% (CS-2), and 65.99% (CS-3), respectively. This proved that cellulose content was relatively enriched in the three components after pretreatment.
3.2 The FTIR and XPS Results of Raw and Pretreated Feedstocks
FTIR was used to analyze the functional groups of the samples before and after microbial pretreatment, and the signal peaks with differences were labeled (As shown in Fig. 2). 3420 cm−1 is the stretching vibration peak of -OH [22]. After pretreatment, the signal intensity decreased, indicating that microorganism can destroy the -OH structure of biomass. 2920 and 2840 cm−1 represent the stretching vibrations of -CH3 or -CH2, which are from the methylation of ester and ether in lignin [23]. While 1640 and 1510 cm−1 are aromatic skeletal vibrations in lignin [5]. After pretreatment, the signal intensity of 2920 and 2840 cm−1 enhanced while that of 1640 and 1510 cm−1 decreased, indicating that corn straw’s lignin structure was destroyed. 1250 cm−1 is the C-O breathing vibration of xylan [22], which was enhanced after pretreatment. Xylan is the basic unit structure of hemicellulose, which indicates that the hemicellulose can be decomposed after pretreatment. Meanwhile, 1050 and 899 cm−1 are the C-O-C stretching and C-H stretching vibrations of cellulose [24]. The enhanced signal intensity illustrated that the cellulose was enriched, which was consistent with the trend of the three components.
Figure 2: The chemical structure analysis of raw and pretreated feedstocks (a) FTIR analysis; (b–i) XPS analysis
XPS was used for further analysis of functional groups of samples, and the relative contents of each functional group were shown in Table 1. The fitted fine spectrum can be seen in Figs. 2b–2i. In the C1s spectrum, 284.8 eV, 286.0 ± 0.9 eV, and 287.9 ± 0.6 eV correspond to the functional groups -C-C-/-C=C-, -C-OR and -C=O, respectively [25]. It can be seen from the results that after pretreatment, the change of -C=O was not obvious. While the functional groups of -C-C-/-C=C- decreased and the functional groups of -C-OR increased. This trend became more obvious with the increase in pretreatment time. Under the 3 weeks of pretreatment, the relative content of -C-C-/-C=C- decreased to 58.64%, while that of -C-OR increased to 30.95%, which was 2.68 times that of CS (11.53%). The structure of -C-C-/-C=C- is mainly provided by the aromatic structure in lignin. After pretreatment, the decrease of -C-C-/-C=C- proved that the structure of lignin was decomposed, which was consistent with the results of FTIR and three components. The -C-OR may be composed of -C-O-C- and -C-OH from alcohol, phenol, and ether groups [25].
Combining the results in the O1s spectrum, 533.3 ± 0.5 eV and 532.5 ± 0.3 eV are -C-O-C- and -O-C=O/-C-OH functional groups, respectively [24]. After pretreatment, the content of -C-O-C- increased, while O-C=O/C-OH decreased. This trend was significant for the CS-3. While the relative content of -C=O had little change in the C1s spectrum, which meant that in the C-O functional groups, the relative content of -C-O-C- was obviously increased, and the -C-OH was removed. This result may be due to the enrichment of cellulose, which contained considerable C-O-C functional groups, and reduction of C-OH. The decrease of C-OH can reduce the hydrogen bond grid formed by a large number of -OH, which may be conducive to the destruction of the dense structure formed by microcrystalline cellulose with high crystallinity [26].
3.3 The AAEMs Result of Raw and Pretreated Feedstocks
The AAEMs result of before and after pretreatment feedstocks were analyzed (Table 2). The results showed that after pretreatment, the AAEMs were reduced, and the maximum removal rates of Ca, K, Mg, and Na reached 22.00%, 44.56%, 28.50%, and 39.56%, respectively. AAEMs are located in cross-linked structures of biomass. When these structures are broken by the microorganism, the AAEMs can release and then remove [27]. The destruction of biomass surface structure can be confirmed by the SEM result (Fig. S2). Interestingly, with the extension of pretreatment time, the content of AAEMs in biomass was slightly increased, especially in the CS-2. Considering that K, Ca, Mg, and Na are the essential elements for microbial growth, microorganisms may fix or absorb some AAEMs in the solid phase with the increase of pretreatment time [28,29]. The whole process may be a competitive process of AAEMs removal and fixation, resulting in a slight increase in the content of AAEMs at the CS-2.
During the pyrolysis, AAEMs can strongly catalyze C-C bond breaking and destroy glucopyranoside and glucofuranose to cause the decomposition of sugar rings [30]. This will reduce the content of sugars in bio-oil, which is harmful to the production of high value-added chemicals such as levoglucosan, affecting the high-value utilization of bio-oil. Meanwhile, AAEMs also affect the production of char in pyrolysis products, which can cause more char to be produced [31]. This result is bad for the catalytic pyrolysis process because a large amount of char can block the catalyst pores, resulting in catalyst deactivation and reducing the catalytic effect. In previous studies, the influence of alkali metals is more significant than that of alkaline earth metals. The influence of AAEMs on producing char is as follows: Na > K > Ca > Mg [32], which may be caused by the difference in ash fusion point. In this study, a good removal effect can be achieved for alkali metals such as Na and K, which can be conducive to the improvement of catalytic pyrolysis efficiency.
3.4 The Thermogravimetric Analysis of Raw and Pretreated Feedstocks
A thermogravimetric analyzer was used to study the pyrolysis process of biomass before and after pretreatment. The results were shown in Fig. S3 and Table 3. After pretreatment, the residual rate of biomass decreased, and the average decomposition rate (Da) increased. This trend increased with the pretreatment time because microbial pretreatment destroyed the biomass structure to promote heat conduction and substance decomposition in the pyrolysis process.
The biomass pyrolysis process can be generally divided into three stages [19]. The first stage is from an ambient temperature of 200°C. At this stage, the inner water is the main weight loss accompanied by slight volatilization of organic matter. The n ext stage (200°C–400°C) is the maximum weight loss stage. In this stage, the biomass breaks down dramatically under high temperature and produces lots of volatile substances. The third stage is form 400°C to the end. Because of the release of large amounts of volatile matter in biomass, this stage is the calcination stage of residual carbon. As for the result, it was found that after microbial pretreatment, the weight loss rate of the second stage (m2) increased significantly, which can reach 56.36% at CS-3. And the maximum decomposition rate (Dmax) after microbial pretreatment also increased. Dmax can reach 7.86 %/min at CS-3, which increased by about 20% compared with CS. These results again indicated that microorganism caused the destruction of biomass structure to promote the pyrolysis of biomass.
Interestingly, after pretreatment, the maximum weight loss temperature moved to a higher temperature, in which all pretreatment samples increased by an average of 20°C. It may be caused by the removal of AAEMs [33]. Meanwhile, after pretreatment, the feedstocks also showed an obvious shoulder peak, which belonged to the pyrolysis shoulder peak of cellulose [34]. It was caused by the increase in the ratio of cellulose to lignin. This illustrated that the cellulose was enriched in biomass components, which was also the same as the result of Section 3.1.
3.5 The Pyrolysis Bio-Oil Analysis of Raw and Pretreated Feedstocks
3.5.1 The Fast Pyrolysis Bio-Oil Analysis of Samples
In this section, Py-GCMS was used to analyze the samples. The corn straw without catalyst was pyrolyzed to discuss the changes of bio-oil components in the pyrolysis products of raw and pretreat feedstocks. As for the result (Fig. 3a), after microbial pretreatment, the total peak area of the sample was improved. When the pretreatment time was 3 weeks, the total peak area reached 243,817,000, while that of the CS only had 13842400. The total peak area of the Py-GCMS result can reflect the content of production during pyrolysis. The more the total peak area, the more substance content is produced. This result illustrated that microbial pretreatment promoted the decomposition of biomass. Compared with the recalcitrant polymeric substances, these depolymerized substances can decompose better during pyrolysis and produce more substances, which increased the total peak area. Meanwhile, the destruction of the surface structure of biomass also promoted better heat conduction, making the exhaustive decomposition of biomass in the pyrolysis process.
Figure 3: The fast and catalytic pyrolysis bio-oil of raw and pretreated feedstocks (a) fast pyrolysis products; (b) catalytic pyrolysis products
The relative content of pyrolytic bio-oil was analyzed. Acids and phenols were the main products in the bio-oil, accounting for about 40%. After microbial pretreatment, the phenol’s relative content was significantly reduced. After 3 weeks of pretreatment, the relative content of phenols decreased to 16.93%, which decreased by 6.83% compared with 23.76% of the CS. Since the precursor of phenols in fast pyrolysis is lignin, which composed of phenylpropane structural units [35], this result may be caused by the destruction and removal of lignin, since the precursor of phenols in fast pyrolysis is lignin, which composed of phenylpropane structural units. When lignin was depolymerized and removed, the benzene ring structure was removed, resulting in the reduction of the phenol’s relative content during fast pyrolysis, which was the same as the result of FTIR and three components. Meanwhile, the relative content of alcohol also decreased. This may be because the -OH connection between the corn straw structure was destroyed by microorganism. The contents of linear carbonyls, furans, and acids were all increased after microbial pretreatment, and the contents further increased with the increase of pretreatment time. Especially, the content of furans was increased up to 4.14% in the CS-3, which is 1.90 times the CS. This was due to the enrichment of cellulose in the three components. The source of furans mainly comes from the fracture and isomerization of cellulose and hemicellulose [36]. And the linear carbonyls and acids from the pyrolysis of lignocellulose were mainly derived from the conversion of small molecule intermediates produced from the breakdown of the glucopyranose ring in cellulose [36,37].
Moreover, the content of sugars in bio-oil was also increased after pretreatment. Relevant studies have shown that because AAEMs can catalyze the fracture of glucopyranoside and glucofuranose to reduce the precursors of sugar, the removal of AAEMs and the increase of cellulose content can be beneficial to increase the content of sugars in bio-oil during fast pyrolysis [5,30]. This way provided a new idea for making high value-added chemicals from biomass. However, in the sample of CS-3, the content of sugars lightly decreased, which may be caused by the depolymerization of cellulose to promote the break of chemical bonds. The above results again proved that after microbial pretreatment, the cellulose content was relatively enriched, and the lignin was decreased.
3.5.2 The Catalytic Pyrolysis Bio-Oil Analysis of Samples
In this section, the catalytic pyrolysis products of corn straw were analyzed by adding ZSM-5 catalysis. The primary gas produced by catalytic pyrolysis can pass through the pore of ZSM-5, which had hydrocarbon pool reaction and shape selection deoxidation. It can greatly increase the content of hydrocarbons, especially aromatics in bio-oil [38].
The relevant results were shown in Fig. 3b. With the increase in pretreatment time, the total peak area of catalytic pyrolysis samples significantly increased, showing the same results as that of fast pyrolysis. Especially the total peak area of CS-3 significantly increased to 618516502, which was about 2.14 times higher than that of CS-2 (288642018). Combined with the SEM (as shown in Fig. S2) and three components results, two reasons led to this result. On the one hand, the corn straw had been fully decomposed by microorganism, which can realize the decomposition and release of substances in the pyrolysis process. On the other hand, the ZSM-5 can promote pyrolysis and reduce the activation energy of pyrolysis reactions to catalyze the decomposition of substances [34]. Their combined action made the CS-3 sample produce more substances and achieve a large increase in the total peak area. For the catalytic pyrolysis products of corn straw, due to the excellent shape selection for aromatic hydrocarbons, aromatic hydrocarbons were the main substances in hydrocarbon in the pyrolysis products [39]. After pretreatment, the relative contents of total hydrocarbon and total aromatics increased with the increase of pretreatment time, reaching the maximum at CS-3. In the sample of CS-3, the contents of total hydrocarbon and aromatics reached 34.37% and 30.59%, respectively, which were 1.57 and 1.49 times higher than the CS of 21.89% and 20.58%, respectively. This indicated that after microbial pretreatment, the depolymerized biomass structure can better synthesize hydrocarbons through ZSM-5 to increase the content of total hydrocarbons and aromatics.
In the process of catalytic pyrolysis, ZSM-5 can catalyze the production of a large number of aromatic compounds, including simple aromatic hydrocarbons, such as benzene, toluene, and para toluene, commonly known as MAHs (mono aromatic hydrocarbons), and other aromatic hydrocarbons, such as naphthalene, methyl naphthalene, commonly known as PAHs (polycyclic aromatic hydrocarbons). To further analyze the variation of the contents of these two types of hydrocarbons, the contents of MAHs and PAHs were calculated. After microbial pretreatment, the content of MAHs increased, reaching 28.21% in the CS-3 sample, which was 1.96 times higher than that of the CS (14.40%). The increase of MAHs was due to the shape selection catalysis of ZSM-5 by a “hydrocarbon pool” composed of polymethyl benzene during the pyrolysis process. Compared with the CS, the PAHs of pretreated samples decreased. It can reach the lowest value (2.38%) at CS-3, which decreased by 3.80% compared with CS (6.18%). The results showed that the depolymerization of biomass components by microbial pretreatment reduced the content of PAHs. More importantly, PAHs are important precursors of coke generation during catalytic pyrolysis, which have potential risks of clogging catalysts and leading to catalyst deactivation [40]. Meanwhile, PAHs are toxic, mutant, and carcinogenic, which can cause various harm to the human body, such as the respiratory, circulatory, and nervous systems [41]. Microbial pretreatment effectively reduced the composition of the PAHs, which played a positive role in producing high-quality bio-oil.
3.5.3 The Carbon Content Component Ratio in Catalytic Pyrolysis Bio-Oil
Substances above C5 are the main components of bio-oil. The carbon content can reflect the proportion of light and heavy components in bio-oil, so it is necessary to focus on compounds above C5. According to the carbon content, the bio-oil components are divided into three parts, C5–C9, C10–C14, and ≥C15 (as shown in Fig. 4). In the sample of CS, the bio-oil component of C5–C9, C10–C14, and ≥C15 accounted for 66.67%, 26.63%, and 6.70%, respectively, which had a certain heavy component in bio-oil. After pretreatment, the proportion of ≥C15 in CS-1, CS-2, and CS-3 decreased, which reached 1.90%, 1.78%, and 0.00%, respectively. When the pretreatment time was 3 weeks, The substances of ≥C15 were not detected. This indicated that after microbial pretreatment, the overall component structure of corn straw can be depolymerized. Moreover, these depolymerized substances can be better decomposed in the pyrolysis process to form small molecular substances and reduce the content of heavy components, which can help to alleviate the coking in the catalytic process and promote a better catalytic reaction of the catalyst. With the increase of pretreatment time, the C5–C9 component of bio-oil was also increased, reaching 73.04% in the 3 weeks pretreatment time, which was 6.37% higher than that of CS. The low molecular content of bio-oil increased after pretreatment, which again proved that microbial pretreatment promoted the reaction in the pyrolysis process.
Figure 4: The carbon content component ratio with more than C5 in catalytic pyrolysis bio-oil of raw and pretreated feedstocks
3.6 The Proposed Mechanism of Phanerochaete Chrysosporium Pretreatment Coupled with Catalytic Pyrolysis
In this section, the possible reaction pathways of microbial pretreatment coupled with catalytic fast pyrolysis were proposed. The schematic diagram can be seen in Fig. 5. According to the results of three components, cellulose crystallinity, FTIR, and SEM (as shown in Fig. S2), microbial pretreatment of biomass was an effective method to achieve the decrease of lignin and break the stubborn structure of biomass. Phanerochaete chrysosporium can produce cellulose, hemicellulose hydrolase, and lignin peroxidase [16,17]. After these enzyme substances decompose the surface structure of biomass, microorganism can further decompose the internal structure of corn straw to promote biomass cracking and produce pores of different sizes. Meanwhile, due to the decomposition of the connections of biomass, the content of AAEMs in biomass is also released and reduced.
Figure 5: The schematic of Phanerochaete chrysosporium pretreated mechanism of corn straw coupled with catalytic pyrolysis
In the pyrolysis process, the destruction of biomass structure by microorganism pretreatment can benefit heat conduction and thermal decomposition. Moreover, the change of three components of biomass also adjusted the proportion of pyrolysis products. Due to the decrease of lignin, the production of phenols in the bio-oil decreased. Whereas, as for the enriched cellulose, with the increase of pretreatment time, enriched cellulose can be further depolymerized, resulting in the destruction of the connections between cellulose units. This result can be confirmed in Section 3.1. And then, In the process of pyrolysis, these depolymerized substances can form sugars, furans, acids, linear carbonyls, and other substances after dehydration, decarboxylation, dehydroxylation, and ring decomposition to increase their relative content of them in bio-oil [2,36,37].
When the ZSM-5 catalyst is added, there are two reactions. Firstly, aliphatic hydrocarbons pass through ZSM-5 pores to be catalyzed into aromatics by shape selection via oligomerization and aromatization [39]. Secondly, a large number of sugars, furans, acids, carbonyls, and other substances, especially furans, produced in the pyrolysis process of enriched cellulose can depend on the “hydrocarbon pool” formed by ZSM-5 via shape-selective, deoxygenation, decarbonylation, aromatization, cracking and Da (Diels-Alder) reaction to synthesize aromatics [38,42]. These reactions greatly promote the content of hydrocarbons and armotics in bio-oil. Microbial pretreatment also increased the proportion of C5-C9 in bio-oil and reduced the heavy components (≥C15), which can significantly benefit the entry of primary pyrolysis steam into the catalyst pores and reduce the coking on the catalyst surface to improve the catalytic efficiency and hydrocarbon contents.
In short, it is feasible to use Phanerochaete chrysosporium to pretreat corn straw combined with catalytic pyrolysis to produce hydrocarbon-rich bio-oil. Microbial treatment is a green, economical, and environmentally friendly method to efficiently pretreated biomass. Moreover, Since the whole process is mild and environmentally friendly, the problem of long processing times can be solved during the stacking process on the farm. We hope that this paper can supplement the theory of microbial pretreatment of biomass, and then provide a novel idea for the utilization of biomass after microbial pretreatment.
This paper proposed a biorefining pretreatment way by Phanerochaete chrysosporium coupled with fast pyrolysis to produce hydrocarbon-rich bio-oil. Ascribed to the removal of lignin, enrichment of cellulose, and destruction of the stubborn structure of biomass after microbial pretreatment, furans, sugars, linear carbonyls, and acids increased, while phenols decreased during fast pyrolysis. Additionally, under the action of the hydrocarbon pool of the ZSM-5 catalyst, the depolymerized biomass can be catalyzed better to improve the contents of total hydrocarbon and aromatics, which can be increased to 34.37% and 30.59%, respectively. This study provided a green and sustainable way to produce hydrocarbon-rich bio-oils.
Funding Statement: This work was supported by the Natural Science Fund Program of China (No. 52306043), the Science and Technology Project of Jiangsu Province (Nos. BE2022604 & BE2021701), the Youth Talent Promotion Project of Jiangsu Provincial Association for Science and Technology (No. JSTJ-2022-046), the Inner Mongolia Major Science and Technology Project (No. 2021ZD0022), and the State Key Laboratory of High-Efficiency Utilization of Coal and Green Chemical Engineering (China) (Nos. 2022-K25 & 2022-K37).
Conflicts of Interest: The authors declare that they have no conflicts of interest to report regarding the present study.
References
1. Kucherov, F. A., Gordeev, E. G., Kashin, A. S., Ananikov, V. P. (2017). Three-dimensional printing with biomass-derived PEF for carbon-neutral manufacturing. Angewandte Chemie-International Edition, 56(50), 15931–15935. [Google Scholar] [PubMed]
2. Zhang, B., Zhong, Z., Xie, Q., Liu, S., Ruan, R. (2016). Two-step fast microwave-assisted pyrolysis of biomass for bio-oil production using microwave absorbent and HZSM-5 catalyst. Journal of Environmental Sciences, 45, 240– 247. [Google Scholar]
3. Chen, Z., Wang, M., Jiang, E., Wang, D., Zhang, K. et al. (2018). Pyrolysis of torrefied biomass. Trends in Biotechnology, 36(12), 1287–1298. [Google Scholar] [PubMed]
4. Wu, K., Wu, H., Zhang, H., Zhang, B., Wen, C. et al. (2020). Enhancing levoglucosan production from waste biomass pyrolysis by Fenton pretreatment. Waste Management, 108, 70–77. [Google Scholar] [PubMed]
5. Wu, K., Luo, B., Wu, H., Chen, M., Wang, Y. et al. (2022). Enhancement of the production of chemicals and liquid fuels from grass biowaste via NaOH-Fenton pretreatment coupled with fast pyrolysis. Energy Conversion and Management, 251, 114954. [Google Scholar]
6. Jeong, S. Y., Lee, E. J., Ban, S. E., Lee, J. W. (2021). Structural characterization of the lignin-carbohydrate complex in biomass pretreated with Fenton oxidation and hydrothermal treatment and consequences on enzymatic hydrolysis efficiency. Carbohydrate Polymers, 270, 118375. [Google Scholar] [PubMed]
7. Lu, H., Liu, S., Shi, Y., Chen, Q. (2022). Efficient delignification of sugarcane bagasse by Fenton oxidation coupled with ultrasound-assisted NaOH for biotransformation from Agaricus sinodeliciosus var. Chaidam. Chemical Engineering Journal, 448, 137719. [Google Scholar]
8. Zhang, S., Zhu, S., Zhang, H., Chen, T., Xiong, Y. (2018). Catalytic fast pyrolysis of rice husk: Effect of coupling leaching with torrefaction pretreatment. Journal of Analytical and Applied Pyrolysis, 133, 91–96. [Google Scholar]
9. Bao, X., Guo, G., Huo, W., Li, Q., Xu, Q. et al. (2022). Ensiling pretreatment fortified with laccase and microbial inoculants enhances biomass preservation and bioethanol production of alfalfa stems. Science of the Total Environment, 857(2), 159442. [Google Scholar] [PubMed]
10. Titiri, E., Filippi, K., Giannakis, N., Vlysidis, A., Koutinas, A. et al. (2023). Optimisation of alkaline pretreatment of spent coffee grounds for microbial oil production by Cryptococcus curvatus. Biochemical Engineering Journal, 193, 108841. [Google Scholar]
11. Liu, X., Hiligsmann, S., Gourdon, R., Bayard, R. (2017). Anaerobic digestion of lignocellulosic biomasses pretreated with Ceriporiopsis subvermispora. Journal of Environmental Management, 193, 154–162. [Google Scholar] [PubMed]
12. Guo, H., Zhao, Y., Chen, X., Shao, Q., Qin, W. (2019). Pretreatment of miscanthus with biomass-degrading bacteria for increasing delignification and enzymatic hydrolysability. Microbial Biotechnology, 12(4), 787–798. [Google Scholar] [PubMed]
13. He, J., Zhu, N., Xu, Y., Wang, L., Zheng, J. et al. (2022). The microbial mechanisms of enhanced humification by inoculation with Phanerochaete chrysosporium and Trichoderma longibrachiatum during biogas residues composting. Bioresource Technology, 351, 126973. [Google Scholar] [PubMed]
14. García-Torreiro, M., López-Abelairas, M., Lu-Chau, T. A., Lema, J. M. (2016). Fungal pretreatment of agricultural residues for bioethanol production. Industrial Crops and Products, 89, 486–492. [Google Scholar]
15. Xu, C., Ma, F., Zhang, X., Chen, S. (2010). Biological pretreatment of corn stover by Irpex lacteus for enzymatic hydrolysis. Journal of Agricultural and Food Chemistry, 58(20), 10893–10898. [Google Scholar] [PubMed]
16. Qin, Y., Wang, N., Ma, Z., Li, J., Wang, Y. et al. (2022). A mechanistic study on electro-Fenton system cooperating with phangerochate chrysosporium to degrade lignin. RSC Advances, 12, 17285–17293. [Google Scholar] [PubMed]
17. Sun, Z., Mao, Y., Liu, S., Zhang, H., Xu, Y. et al. (2022). Effect of pretreatment with Phanerochaete chrysosporium on physicochemical properties and pyrolysis behaviors of corn stover. Bioresource Technology, 361, 127687. [Google Scholar] [PubMed]
18. Segal, L., Creely, J., Martin, A., Conrad, C. (1959). An empirical method for estimating the degree of crystallinity of native cellulose using the X-Ray diffractometer. Textile Research Journal, 29(10), 786–794. [Google Scholar]
19. Wang, J., Zhang, B., Shujaa Aldeen, A., Mwenya, S., Cheng, H. et al. (2022). Enhancing production of hydrocarbon-rich bio-oil from biomass via catalytic fast pyrolysis coupled with advanced oxidation process pretreatment. Bioresource Technology, 359, 127450. [Google Scholar] [PubMed]
20. Ahmed-Haras, M. R., Kao, N., Ward, L., Islam, M. S. (2020). Insights into the production and physicochemical properties of oxycellulose microcrystalline with coexisting crystalline forms. International Journal of Biological Macromolecules, 146, 150–161. [Google Scholar] [PubMed]
21. Liu, Z., Inokuma, K., Ho, S. H., den Haan, R., van Zyl, W. H. et al. (2017). Improvement of ethanol production from crystalline cellulose via optimizing cellulase ratios in cellulolytic Saccharomyces cerevisiae. Biotechnology and Bioengineering, 114(6), 1201–1207. [Google Scholar] [PubMed]
22. Pandey, K. K., Pitman, A. J. (2003). FTIR studies of the changes in wood chemistry following decay by brown-rot and white-rot fungi. International Biodeterioration & Biodegradation, 52(3), 151–160. [Google Scholar]
23. Chen, W. H., Wang, C. W., Ong, H. C., Show, P. L., Hsieh, T. H. (2019). Torrefaction, pyrolysis and two-stage thermodegradation of hemicellulose, cellulose and lignin. Fuel, 258, 116168. [Google Scholar]
24. Grams, J. (2022). Surface analysis of solid products of thermal treatment of lignocellulosic biomass. Journal of Analytical and Applied Pyrolysis, 161, 105429. [Google Scholar]
25. Chen, D., Cen, K., Cao, X., Li, Y., Zhang, Y. et al. (2018). Restudy on torrefaction of corn stalk from the point of view of deoxygenation and decarbonization. Journal of Analytical and Applied Pyrolysis, 135, 85–93. [Google Scholar]
26. Wei, X., Wang, Y., Li, J., Wang, F., Chang, G. et al. (2018). Effects of temperature on cellulose hydrogen bonds during dissolution in ionic liquid. Carbohydrate Polymers, 201, 387–391. [Google Scholar] [PubMed]
27. Zheng, A., Zhao, Z., Huang, Z., Zhao, K., Wei, G. et al. (2015). Overcoming biomass recalcitrance for enhancing sugar production from fast pyrolysis of biomass by microwave pretreatment in glycerol. Green Chemistry, 17(2), 1167–1175. [Google Scholar]
28. Liu, L., Tan, Z., Gong, H., Huang, Q. (2018). Migration and transformation mechanisms of nutrient elements (N, P, K) within biochar in straw-biochar–soil-plant systems: A review. ACS Sustainable Chemistry & Engineering, 7(1), 22–32. [Google Scholar]
29. Treesubsuntorn, C., Dhurakit, P., Khaksar, G., Thiravetyan, P. (2018). Effect of microorganisms on reducing cadmium uptake and toxicity in rice (Oryza sativa L.). Environmental Science and Pollution Research, 25, 25690–25701. [Google Scholar] [PubMed]
30. Zhang, H., Ma, Y., Shao, S., Xiao, R. (2017). The effects of potassium on distributions of bio-oils obtained from fast pyrolysis of agricultural and forest biomass in a fluidized bed. Applied Energy, 208, 867–877. [Google Scholar]
31. Dalluge, D. L., Kim, K. H., Brown, R. C. (2017). The influence of alkali and alkaline earth metals on char and volatile aromatics from fast pyrolysis of lignin. Journal of Analytical and Applied Pyrolysis, 127, 385–393. [Google Scholar]
32. Mahadevan, R., Adhikari, S., Shakya, R., Wang, K., Dayton, D. et al. (2016). Effect of alkali and alkaline earth metals on in-situ catalytic fast pyrolysis of lignocellulosic biomass: A microreactor study. Energy & Fuels, 30(4), 3045–3056. [Google Scholar]
33. Jiang, G., Nowakowski, D. J., Bridgwater, A. V. (2010). Effect of the temperature on the composition of lignin pyrolysis products. Energy & Fuels, 24(8), 4470–4475. [Google Scholar]
34. Zhong, S., Zhang, B., Liu, C., Shujaa Aldeen, A. (2021). Mechanism of synergistic effects and kinetics analysis in catalytic co-pyrolysis of water hyacinth and HDPE. Energy Conversion and Management, 228, 113717. [Google Scholar]
35. Shuai, L., Talebi Amiri, M., Luterbacher, J. S. (2016). The influence of interunit carbon-carbon linkages during lignin upgrading. Current Opinion in Green and Sustainable Chemistry, 2, 59–63. [Google Scholar]
36. Chen, L., Liao, Y., Guo, Z., Cao, Y., Ma, X. (2019). Products distribution and generation pathway of cellulose pyrolysis. Journal of Cleaner Production, 232, 1309–1320. [Google Scholar]
37. Wang, T., Ai, Y., Peng, L., Zhang, R., Lu, Q. et al. (2018). Pyrolysis characteristics of poplar sawdust by pretreatment of anaerobic fermentation. Industrial Crops and Products, 125, 596–601. [Google Scholar]
38. Zhang, B., Zhong, Z., Zhang, J., Ruan, R. (2018). Catalytic fast co-pyrolysis of biomass and fusel alcohol to enhance aromatic hydrocarbon production over ZSM-5 catalyst in a fluidized bed reactor. Journal of Analytical and Applied Pyrolysis, 133, 147–153. [Google Scholar]
39. Wang, J., Zhong, Z., Ding, K., Li, M., Hao, N. et al. (2019). Catalytic fast co-pyrolysis of bamboo sawdust and waste tire using a tandem reactor with cascade bubbling fluidized bed and fixed bed system. Energy Conversion and Management, 180, 60–71. [Google Scholar]
40. Wang, S., Li, Z., Yi, W., Fu, P., Zhang, A. et al. (2021). Renewable aromatic hydrocarbons production from catalytic pyrolysis of lignin with Al-SBA-15 and HZSM-5: Synergistic effect and coke behaviour. Renewable Energy, 163, 1673–1681. [Google Scholar]
41. Ma, Y., Liu, A., Egodawatta, P., McGree, J., Goonetilleke, A. (2017). Quantitative assessment of human health risk posed by polycyclic aromatic hydrocarbons in urban road dust. Science of the Total Environment, 575, 895–904. [Google Scholar] [PubMed]
42. Wijaya, Y. P., Kristianto, I., Lee, H., Jae, J. (2016). Production of renewable toluene from biomass-derived furans via Diels-Alder and dehydration reactions: A comparative study of Lewis acid catalysts. Fuel, 182, 588–596. [Google Scholar]
Supplementary Materials
Figure S1: The result of XRD analysis of raw and pretreated feedstocks
Figure S2: The SEM images of raw and pretreated feedstocks: (a), (e) CS; (b), (f) CS-1; (c), (g) CS-2; (d), (h) CS-3
Figure S3: The TG/DTG analysis of raw and pretreated feedstocks (a) TG (b) DTG
References
1. García-Torreiro, M., López-Abelairas, M., Lu-Chau, T. A., Lema, J. M. (2016). Fungal pretreatment of agricultural residues for bioethanol production. Industrial Crops and Products, 89, 486–492.
2. Xu, C., Ma, F., Zhang, X., Chen, S. (2010). Biological pretreatment of corn stover by Irpex lacteus for enzymatic hydrolysis. Journal of Agricultural and Food Chemistry, 58, 10893–10898.
3. Salvachua, D., Prieto, A., Lopez-Abelairas, M., Lu-Chau, T., Martinez, A. T. et al. (2011). Fungal pretreatment: An alternative in second-generation ethanol from wheat straw. Bioresource Technology, 102, 7500–7506.
4. Alexandropoulou, M., Antonopoulou, G., Fragkou, E., Ntaikou, I., Lyberatos, G. (2017). Fungal pretreatment of willow sawdust and its combination with alkaline treatment for enhancing biogas production. Journal of Environmental Management, 203, 704–713.
5. Xie, C., Gong, W., Yang, Q., Zhu, Z., Yan, L. et al. (2017). White-rot fungi pretreatment combined with alkaline/oxidative pretreatment to improve enzymatic saccharification of industrial hemp. Bioresource Technology, 243, 188–195.
6. Chen, Y., Sharma-Shivappa, R. R., Chen, C. (2007). Ensiling agricultural residues for bioethanol production. Applied Biochemistry and Biotechnology, 143, 80–92.
7. Grubišić, M., Galić Perečinec, M., Peremin, I., Mihajlovski, K., Beluhan, S. et al. (2022). Optimization of pretreatment conditions and enzymatic hydrolysis of corn cobs for production of microbial lipids by trichosporon oleaginosus. Energies, 15, 3208.
8. Wu, K., Wu, H., Zhang, H., Zhang, B., Wen, C. et al. (2020). Enhancing levoglucosan production from waste biomass pyrolysis by Fenton pretreatment. Waste Management, 108, 70–77.
9. Titiri, E., Filippi, K., Giannakis, N., Vlysidis, A., Koutinas, A. et al. (2023). Optimisation of alkaline pretreatment of spent coffee grounds for microbial oil production by Cryptococcus curvatus. Biochemical Engineering Journal, 193, 108841.
Cite This Article
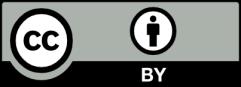