Open Access
REVIEW
Synthesis of Metallic Nanoparticles Based on Green Chemistry and Their Medical Biochemical Applications: Synthesis of Metallic Nanoparticles
1
Department of Pharmaceutical Sciences, University of Kwazulu-Natal, Private Bag X54001, Durban, South Africa
2
Department of Chemical Pathology, School of Pathology, Faculty of Health Sciences, University of the Free State, Bloemfontein,
South Africa
3
Faculty of Pharmaceutical Sciences, University of Lubumbashi, Lubumbashi, Democratic Republic of Congo
4
Department of Biotechnology and Food Sciences, Durban University of Technology, Durban, South Africa
5
Department of Chemistry, M. Kumarasamy College of Engineering, Karur, Tamilnadu, India
6
Department of Botany, Mahatma Gandhi Central University, Motihari, Bihar, India
* Corresponding Authors: Anand Krishnan. Email: ; Ram Prasad. Email:
(This article belongs to the Special Issue: New Insights on Nanomaterials for Energy, Environmental and Agricultural Applications)
Journal of Renewable Materials 2023, 11(6), 2575-2591. https://doi.org/10.32604/jrm.2023.026159
Received 19 August 2022; Accepted 21 October 2022; Issue published 27 April 2023
Abstract
Nanoparticles have distinct properties that make them potentially valuable in a variety of industries. As a result, emerging approaches for the manufacture of nanoparticles are gaining a lot of scientific interest. The biological pathway of nanoparticle synthesis has been suggested as an effective, affordable, and environmentally safe method. Synthesis of nanoparticles through physical and chemical processes uses unsafe materials, expensive equipment and adversely affects the environment. As a result, in order to support the increased utilization of nanoparticles across many sectors, nanotechnology research activities have shifted toward environmentally safe and cost-effective techniques that outperform chemical and/or biological procedures. The use of organisms to produce metal nanoparticles is among the most frequently discussed methods. Plants appear to be the best candidates among these organisms for large-scale nanoparticle biosynthesis. Medicinal plants have been employed as reducing agents and NP stabilizers to minimize the toxicity of NPs in both the environment and the human body. Furthermore, the presence of certain functional components in plant extracts may be extremely useful and effective for the human body. Polyphenol, for example, which may have antioxidant properties, might intercept free radicals before they interact with other biomolecules and cause considerable damage. The current article analyzes the most recent developments and improvements in the green synthesis of metal nanoparticles by different plants and the use of these nanoparticles for various biomedical applications and hopes to provide insights into this exciting research frontier.Graphical Abstract
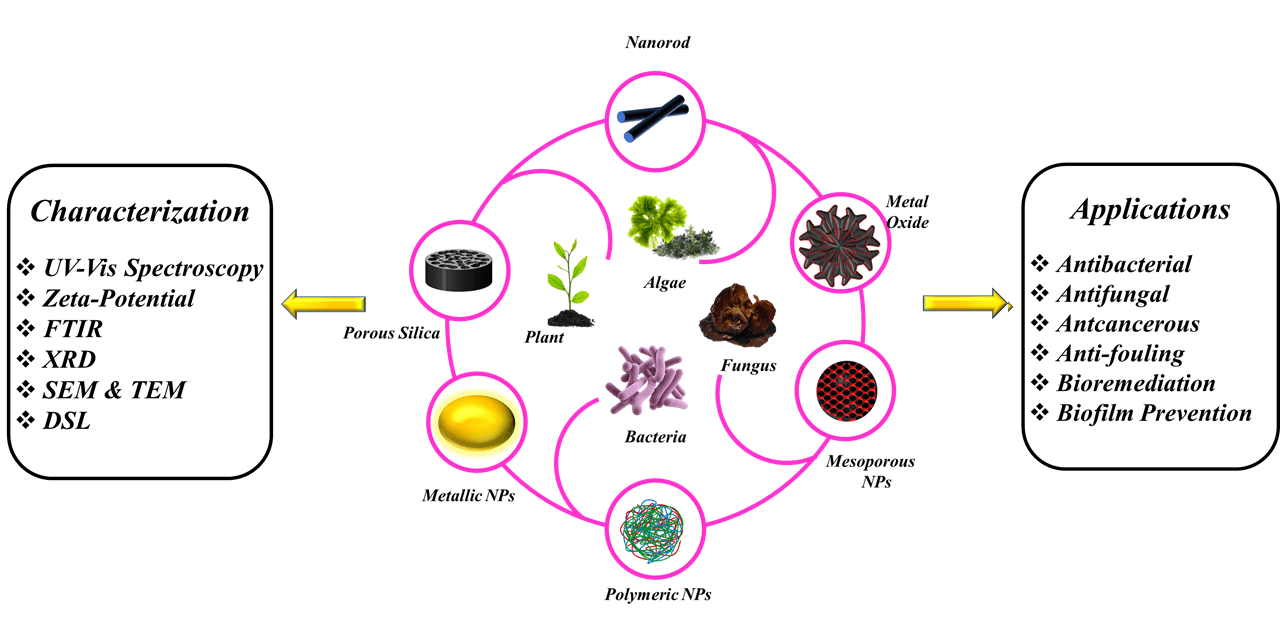
Keywords
Nanotechnology studies nanoscale material characteristics based on size, shape, and morphology [1]. Nanotechnology is highly significant in current research because of its versatility in many disciplines, comprising the chemical industry, energy sciences, electronics, health, food, biomedical sciences, pharmaceuticals, cosmetics, environmental health, mechanics, and space industry [2]. Metallic nanoparticles are nanosized particles with diameters ranging from 1 to 100 nm that are gaining steam due to applications in fields ranging from electronics to sensing, environmental cleanup, oil recovery, and drug delivery. Because of their tiny size, vast surface area with free dangling bonds, and more significant surface energy, metal nanoparticles have higher surface energy and characteristics than bulk materials [3]. Despite numerous nanoparticle synthesis techniques, toxic chemicals and the high cost of the process render it unsuitable for continuous production [4].
As a result, the importance of a sustainable, economic, and ecologically sustainable approach has grown tremendously. In relation to other physical and chemical procedures being used, the green production of nanoparticles (NPs) is a new research trend in green nanotechnology. This is due to the fact that it is non-toxic or less dangerous, eco-friendly, efficient, and cost-effective. Plants, bacteria, algae, and fungi are the biological agents that produce green NPs [5]. The most preferred method is biosynthesis using plant extract since plant extract may serve as both a reducing and a stabilizing agent in the nanoparticle synthesis process [6]. This review discusses the most recent research in NPs biosynthesis and highlights its potential in the medical field.
2 Green Synthesis of Nanoparticles
Current nanoparticle production processes are expensive, unsafe, and harmful to the environment. To overcome these concerns, scientists have found certain green technologies, such as naturally occurring sources and their products, that may be used to produce nanoparticles [7]. Green nanoparticle production has various potential applications in environmental and therapeutic domains, particularly in reducing toxic substances [8]. Green synthesis may be classified into three types: those that employ microorganisms such as fungus, yeasts, bacteria, and actinomycetes; those that use plants and plant extracts; and those that use membranes, viruses, DNA, and diatoms as templates [9,10]. Plant phytochemicals, as opposed to microbes, have been extensively used. This is because plant phytochemicals exhibit higher reduction and stabilization. Fig. 1 depicts a graphic image of the green synthesis of various metallic nanoparticles and the mechanism of their antibacterial characteristics.
Figure 1: Illustration of the green synthesis of metallic nanoparticles from plants, microbes, and fungi. It also depicts the numerous ways of bacterial cell death [11]. Copyrights© 2020, Elsevier B.V
2.1 Bacteria-Mediated Synthesis of Nanoparticles
Bacteria are prokaryotic, usually unicellular bacteria that lack membrane-bound nuclei; they are prevalent in most known habitats, especially forest ecosystems [12]. Bacteria are classified into two categories based on bacterial cell wall composition. Gram-positive bacteria have a dense layer of peptidoglycan in their cell walls. In contrast, gram-negative bacteria have a thin layer of peptidoglycan and an internal exterior membrane composed of lipopolysaccharide (Fig. 2) [13]. The fast spread of antibiotic resistance in microorganisms and the emergence of multidrug-resistant strains pose a significant danger to global public health [14]. For decades, antibacterial remedies such as antibiotics and other anti-infective drugs have been available. Human diseases that are multidrug-resistant (MDR) are currently regarded as one of the world’s most serious health threats [15]. As a result, attention has been turned toward alternative inorganic metal-based bactericidal agents such as silver, copper, titanium and zinc [16].
Figure 2: Comparison of the bacterial cell wall [13]. Copyrights© 2019, Springer Nature
Bacteria are an excellent choice for biometrics in NP synthesis due to their abundance in nature, exceptional ability to decrease metal ions, and ability to adapt to extreme conditions [17]. Bacteria are good biofactories for synthesizing metallic nanoparticles such as silver and gold because they produce a variety of inorganic elements, either intracellularly or extracellularly [18]. The specific process of NP synthesis by bacteria has yet to be determined. Extracellular metal complexion or precipitation, efflux system, bioaccumulation, absence of specialized metal transportation system, change of solubility, toxicity via reduction or oxidation, and bio-absorption are all possible processes for NP production bacteria [19]. Infections caused by bacterial strains such as Staphylococcus aureus, Shigellaflexneri, and Salmonella sp. are becoming increasingly common. Developing nanoparticles as novel antibacterial agents is a new trend that may be employed in various disciplines, including biosensors, nanomedicine, and bio-luminescence technology [20]. Various microorganisms, including Klebsiella aerogenes, Bacillus subtilis, Pseudomonas stutzeri, Fusarium oxysporum, and Vericillium, have synthesized various types of metallic NPs [21].
Suggested mechanisms for the inhibition activity of nanoparticles on bacteria include adhesion of nanoparticles to the bacterial cell surface, causing cell membrane damage and changes in the transportation systems, penetration of nanoparticles into the cells and their interactions with various biomolecules and organelles that could influence activity inhibitory activity, involvement of nanoparticles in the generation of ROS and causing cell harm, and entry of nanoparticles in the bacterial cell and development of mutations and genotoxicity. Fig. 3 depicts the interaction of nanoparticles with cell components [22].
Figure 3: Interaction of nanoparticles with cell components [22]. Copyrights© 2020, Springer Nature
2.2 Actinomycetes Mediated Synthesis of Nanoparticles
Actinomycetes are filamentous, Gram-positive, spore-forming actinobacteria classified as bacteria and fungi [23]. They are well recognized for their capacity to generate secondary metabolites with diverse biological functions [24,25]. Actinomycetes have received much attention because they have high stability and polydispersity, making them an excellent choice for metal nanoparticle production [26]. Because they exhibit saprophytic behavior and produce a variety of bioactive secondary metabolites and extracellular enzymes, actinomycetes are recognized as superior groupings among microbial species of commercial relevance [27].
It has become clear that the substantial advancement in the synthesis of NPs using actinomycetes is a phase of green chemistry that integrates nanotechnology with microbial biotechnology in a safe, cost-effective, and environmentally benign [28]. These organisms can produce active molecules and compounds that can reduce and stabilize agents in producing nanoparticles with various forms, compositions, and physicochemical characteristics [26]. In particular, endophytic actinomycetes can generate a variety of secondary metabolites, such as enzymes and proteins, which may be utilized for ion reduction and metal capping at the nanoscale [29]. The actinomycetes-based NPs have several benefits over other biological processes frequently employed to make NPs [30].
2.3 Algae-Mediated Synthesis of Nanoparticles
Algae are a vast and varied category of photosynthetic eukaryotic organisms that are autotrophic and polyphyletic. They are primarily divided into macroalgae (seaweeds) and microalgae (unicellular, diatoms, or multicellular) based on morphological features. They can be found on wet rocks and in freshwater and marine habitats [31]. Algae have long been used in commercial and industrial applications as food, feed, additives, cosmetics, medicines, and fertilizer, but the trend is now turning to algae-mediated green nanoparticle production (NPs). Algae are a rich source of secondary metabolites, easy to produce, develop quickly, and adaptable; thus, this trend is growing increasingly [32]. Algae are recognized for their capacity to hyper-accumulate heavy metal ions and restructure them into more flexible forms. Algae have been suggested to be model organisms for synthesizing bio-nano materials due to their attractive properties [33].
Microalgal extracts as capping and reducing agents in nanoparticle production render the procedure non-toxic and ecologically friendly. Algae have a higher growth rate and higher biomass productivity than other microorganisms, making the process inexpensive [34]. Algae include a variety of bioactive compounds, notably polysaccharides, lipids, proteins, vitamins, carotenoids, and polyphenols, which may be useful in synthesizing different metals nanoparticles by activating the detoxifying process in both living cells and cellular extracts [35]. The Biosynthesis of NPs utilizing algae and waste materials is a new and exciting study area. Among the numerous biological techniques utilized for NP synthesis, different types of algae are increasingly being employed as model systems because of their incredible capacity to bioremediate hazardous metals, transforming them into more malleable forms. They can produce a range of metal and metal oxide NPs [36]. Algae are ideal for green synthesis since they can hyper-accumulate metals and convert them into NPs. As shown in Fig. 4, diverse kinds of algae, including blue-green algae, brown algae, green algae, and red algae, produce a variety of metallic and metal oxide NPs [32].
Figure 4: Graphical illustration of algae mediates biosynthesis, characterization, and applications of nanoparticles [32]. Copyrights© 2020, MDPI
2.4 Fungi-Mediated Synthesis of Nanoparticles
Fungi have numerous benefits over plants and other microorganisms in the green biosynthesis of nanoparticles [37]. They are recognized for their incredibly quick ability to absorb metals and reduce metal salts for more significant production of nanoparticles under optimized conditions because they usually form biomass that can also withstand agitation, yield structurally and functionally varied biomolecules, and are simple to handle biochemical processes [38].
Fungi may be grown on large-scale (nano factories) and generate nanoparticles with controlled size and shape. They generate a considerable number of proteins and enzymes, which may be utilized for the rapid and sustainable production of nanoparticles [39]. Because fungi, like bacteria, have high binding capacities, intracellular intake, tolerance, and the ability to bioaccumulate metals, they are used to generate metallic nanoparticles. Phaenerocheate chrysosporium, Fusarium sp., and Colletotrichum sp. have all been used to create nanoparticles. Fungi are more favourable than other microorganisms for nanoparticle synthesis because they grow quickly and are easier to handle and produce in a laboratory procedure than bacteria [17]. In general, fungi-mediated NP synthesis is divided into two categories: in vivo and in vitro techniques. In the first example, the production of NPs happens intracellularly inside live mycelia, and in the latter scenario, the synthesis utilizes fungal cell-free extracts [40].
2.5 Plants-Mediated Synthesis of Nanoparticles
Plant-mediated production of metal nanoparticles has been a hotspot in nanoscience and nanotechnology in recent years. The metal nanoparticles were synthesized by combining the metal precursor with the plant extract under moderate conditions (typically at room temperature) without adding other reducing agents or stabilizers [41]. Many metallic nanoparticles may be synthesized biologically, which is more environmentally friendly and allows for the regulated synthesis of nanoparticles with well-defined sizes and shapes [42]. Fruit extracts, seed powder extracts, seed exudates, peel extracts, bran extracts, bark extracts, flower extracts, and leaf extracts have all been employed in the environmentally friendly synthesis of nanoparticles [43]. Biomolecules in such plant components are responsible for fast-reducing metal salts, capping, and stabilization. Plant-mediated nanoparticles exist in various shapes and sizes, such as spherical, triangular, cubic, and rod-like particles [44]. Phenols, flavonoids, alkaloids, and terpenoids are examples of bioactive secondary metabolites found in medicinal plants. These are used to treat a wide range of ailments, including disorders and infectious infections. Because of their solvent-free nature and low toxicity, the production of nanoparticles from plants has risen in popularity in recent years. Furthermore, their manufacture is both speedier and less expensive [45].
Plant-mediated AgNP synthesis exceeds chemical and physical approaches and might be easily scaled up for large-scale manufacturing. The primary mechanism underlying plant-mediated AgNP generation is a plant-assisted reduction produced by phytochemicals such as ketones, terpenoids, amides, flavones, carboxylic acids, and aldehydes. Metal ions are reduced to metal atoms during the production of metal nanoparticles in a plant extract after bonding with stabilizing and reducing metabolites. A tiny metal nanoparticle is produced due to the interactions between the metal ion and metabolite complex that forms other complexes. The coarsening process then carries about the development of individual tiny particles into larger ones. This process is repeated until the particles have a stable shape and size [46].
3 Applications of Medicinal Plants as Nanomedicine
Jagathesan and Rajiv produced and assessed the antibacterial activity of iron oxide nanoparticles using Eichhornia crassipes leaf extract. The SEM analysis was used to evaluate the shape of the nanoparticles. The Eichhornia-mediated FeNPs that were produced are rod-shaped and organized without aggregation. Antibacterial activity of synthesized FeNPs revealed that at a concentration of 100 µg/ml, iron oxide nanoparticles were effective, S. aureus (23.3 ± 1 mm), P. fluorescens (22.6 ± 1 mm), and E. coli (20 ± 1 mm) had the maximum zones of inhibition. This test demonstrates that FeNPs have antibacterial efficacy against S. aureus, P. fluorescens, and E. coli [47].
Sharmila et al. used Bauhinia tomentose leaf extract to synthesize zinc oxide nanoparticles (ZnONPs). UV–vis, TEM, EDX, XRD, and FTIR studies were used to characterize the green-synthesized ZnONPs. ZnONPs with a distinct hexagonal shape were seen in the TEM image. It was discovered that the ZnO nanoparticles ranged in size from 22 to 94 nm. The antibacterial activity of biosynthesized ZnONPs produced from B. tomentose leaf extract was evaluated against B. subtilis, S. aureus, P. aeruginosa, and E. coli. The results indicated that ZnONPs produced from B. tomentosa leaf extract had a considerable zone of inhibition for P. aeruginosa (20.3 mm) and E. coli (19.8 mm) [48].
Similarly, Aziz et al. used a Mint extract ingredient to produce cupric oxide nanoparticles (CuONPs) and tested their antibacterial efficacy. The nanoparticles’ structural and optical characteristics were investigated using XRD, FE-SEM, and UV-vis. Based on the TEM, the nanoparticles possessed a basic cubic structure with a crystalline size range of 22 to 25 nm. The bactericidal activity was tested on E coli and B. subtilis samples, with inhibitory activity of 35 and 38 mm, respectively [49].
Ailanthus altissima leaf aqueous extract was used by Awwad and Amer to produce copper oxide nanoparticles (CuONPs). Copper oxide nanoparticles were evaluated in terms of shape and crystalline structure using UV-vis, SEM, TEM, and FT-IR analysis techniques and antibacterial activity. The TEM observation revealed that copper oxide nanoparticles produced were crystalline, spherical in form, and had an average particle size of 20 nm. The disk diffusion technique was used to test the antibacterial activity of CuONPs against various microorganisms. S. aureus was shown to have the highest inhibition effect, followed by E. coli [50].
Copper nanoparticles (CuNPs) were synthesized by Rajeshkumar et al. from the rare medicinal plant Cissus arnotiana, and their effectiveness against both gram-positive and gram-negative bacteria was examined. TEM showed that the nanoparticles were typically between 60 and 90 nm in size and had a spherical shape. The biosynthesized CuNPs were more efficient against the gram-negative bacteria E. coli with an inhibitory zone of 22.20 ± 0.16 mm at 75 µg/mL [51].
In the presence of monogyna leaf extract as a reducing and capping agent, Ahodashi et al. synthesized gold and silver nanoparticles the synthesized silver and gold nanoparticles weer analysed using UV-vis, FT-IR, DLS, SEM, TEM with EDAX, and XRD. Silver and gold nanoparticles were 50 and 30 nm in size, according to TEM micrographs. The MIC values for synthetic CML@Ag-NPs and CML@Au-NPs against S. aureus, E. faecalis, P. aeruginosa, A. baumannii, E. coli, K. pneumonia, and P. mirabilis were 0.29 and 750, 0.5 and 185, 0.29, 750, 0.29, 1500 µg/mL, respectively [52].
Shirzadi-Adodashti et al. used an ultrasound-assisted precipitation approach to produce MnFe2O4@SiO2@Au magnetic nanocomposites with glucose, sucrose, and PVA as capping agents and C. pentagyna and C. microphylla as reducing agents. The magnetic nanocomposites’ spherical form, homogeneous distribution, and mean size of roughly 65 nm were confirmed by TEM scans. The findings indicate that the green reducing and capping agents used in the production of high, regular, and small-sized particles can have a considerable impact. The magnetic nanocomposites showed significant inhibitory activity at 1.25 mg/mL against P. aeruginosa, K. pneumoniae, and P. mirabilis [53].
Mycogenic silver nanoparticles from endophytic Trichoderma atroviride were synthesized, and their antimicrobial activity was evaluated by Abdel-Azeem et al. X-ray diffraction, UV-vis, and HRTEM techniques were used to characterize the mycosynthesize. According to the HRTEM data, the dispersion of spherical AgNPs varied from 10 to 15 nm. According to the findings, pathogenic bacteria and fungi were most successfully prevented when mycogenic AgNPs were treated at a concentration of 100 ppm. These studies revealed that AgNPs derived from Trichoderma atroviride native isolates might be exploited to quickly synthesize low-cost, environmentally friendly biomaterials with antibacterial capabilities [54].
Aboutorabi et al. produced silver nanoparticles (AgNPs) by a bio-reduction approach that included aqueous mullein leaf extract as both a reducing and a stabilizing agent. UV-vis, X-ray, SEM, and TEM were used to examine the produced AgNPs. According to electron microscopy studies, polydispersed, spherical AgNPs with an average particle size of roughly 20 nm have developed. These nanoparticles have also been applied to the exhaustion method of treating wound dressings. The newly developed wound dressings inhibited a gram-positive bacterial strain, Staphylococcus aureus [55].
Solorzano-Toala et al. used Annona diversifolia Safford to synthesize silver nanoparticles. The silver nanoparticles were analyzed using spectroscopic and dynamic light scattering techniques. The antibacterial activity of the obtained AgNPs was tested against B. cereus, K. pneumoniae, and E. aerogenes. AgNPs exhibit high values at 3 keV and a homogeneous shape with particles ranging in size from 45 to 58 nm. The antibacterial activity of AgNPs against K. pneumoniae and E. aerogenes shown considerable growth inhibition [56].
Pagar et al. developed CuO nanoparticles from Moringa oleifera leaves extract and tested their antifungal efficacy. XRD, FESEM, EDX, FT-IR, UV-DRS analyses were used to characterize the produced CuONPs. FESEM was used to examine the morphology of biosynthesized CuONPs. Except for little aggregation, the overall average crystalline size of the CuONPs was 35–95 nm, with a quasispherical appearance. The antifungal efficacy of the generated CuONPs against C. albicans, A. niger, A. clavatus, T. mentographytes, and E. floccosum was assessed in-vitro using the Agar plate technique. Compared to the reference standard Griseofulvin, biosynthesized CuO NPs demonstrated moderate efficacy against T. mentographyte, E. floccosum, and good activity against C. albicans [57].
Pillai et al. used four different plant extracts to produce zinc oxide nanoparticles quickly and cheaply (ZnONPs). Beta vulgaris, Cinnamomum tamala, Cinnamomum verum, and Brassica oleracea var. Italica were the plants used in this study. XRD, FTIR, and SEM were used to characterize ZnONPs. The TEM was used to determine nanoparticle size and form. The nanoparticles formed by the aqueous extract of Beta vulgaris were round and almost 202 nm in size. Cinnamomum tamala aqueous extract nanoparticle structure was rod-shaped but isolated small-sized particles with a diameter of 303 nm. According to TEM micrographs of PA1, ZnONPs have a spherical-like structure with some large and other tiny particles of various sizes agglomerated. The antifungal activity of ZnONPs was tested against C. albicans and A. niger stains. B. vulgaris. ZnONPs were shown to be active against A. niger, while C. tamala ZnONPs were found to be active against C. albicans. ZnONPs produced from B. oleracea var. Italica extracts have also demonstrated antifungal efficacy against both stains [58].
Jamdagni et al. produced zinc oxide nanoparticles from Nyctanthes arbor-tristis aqueous floral extract. They tested their antifungal activity against A. alternata, A. niger, B. cinerea, F. oxysporum, and P. expansum. The resultant nanopowder was characterized using XRD, DLS, UV-vis, and TEM. Individual particle sizes in the TEM aggregates of nanoparticles ranged from 12–32 nm. The antifungal findings revealed that A. niger was the most susceptible and had the lowest MIC value (16 µg/mL), whereas B. cinerea and P. expansum had the highest MIC value (128 µg/mL) [59].
Jebril et al. synthesized silver nanoparticles using Melia azedarach leaf extract and assessed their antifungal against Verticillium dahlia. The UV-vs, dynamic light scattering technique, zeta potential, SEM, EDS, XRD, and FTIR were used to examine the nanoparticles. SEM investigation reveals the presence of tiny spherical nanoparticles with diameters ranging from 18 to 30 nm. The in vitro effect of AgNPs treatment on Verticillium dahlia indicated that the pathogenic fungus was significantly inhibited at p ≤ 0.05. Compared to the untreated control, AgNPs slowed the radial development of Verticillium dahliae mycelium [60].
Dawoud et al. used the corn grain contaminant Nigrospora oryzae to biologically synthesize Ag NPs, which were well-characterized using a UV-vs. and XRD, TEM, EDS, and a particle size analyzer. The TEM revealed a varied morphology consisting primarily of spherical nanoparticles 3 to 13 nm in size with a nice Gaussian profile. Eight Fusarium spp. were utilized to assess the antifungal activity of Ag NPs. The results showed that when exposed to 25 to 200 ppm of biosynthesized Ag NPs, all species’ growth was suppressed [61].
Das et al. synthesized copper nanoparticles using a hydroalcoholic extract of M. oleifera leaves, UV-vis, FTIR XRD, and the electron diffraction pattern from HRTEM images all indicated the formation of copper nanoparticles and the participation of biomolecules in this synthesis. The SEM imaging revealed that the produced copper nanoparticles were found to have a particle size range of 35.8–49.2 nm. Copper is found in produced encapsulated nanoparticles, according to EDS analysis. M. oleifera leaf extract and copper nanoparticles demonstrate antibacterial activity against E. coli, K. pneumoniae, S.aureus, and E. faecalis, with MIC values ranging from 250 to 500 µg/mL. M. oleifera leaves extract and produced copper nanoparticles have antifungal activity against A. niger, A. flavus, C. albicans, and C. glabrata, with MIC values ranging from 62.5–250 and 31.2–125 µg/mL, respectively [62].
3.3 Antioxidant Activity of Nanoparticles
Sharmila et al. used Tecoma castanifolia leaf extract to produce ZnO nanoparticles and tested their antioxidant potential. The DPPH test was used to evaluate the free radical scavenging activity of the green produced ZnONPs. ZnONPs were studied using UV-vis spectroscopy, TEM, EDX, XRD, and FTIR. The size and form of the produced ZnO NPs were investigated using TEM, which revealed dark spots of spherical shape with diameters of 70–75 nm. The results showed that as the concentration of ZnONPs increased, the radical scavenging activity reached a peak of 67% at 100 µg/mL [63].
Yousaf et al. produced silver nanoparticles (SNPs) from three extracts of A. millefolium: water, ethanol, and methanol, and assessed their antioxidant ability using the DPPH assay. Various analytical methods such as UV–viss, XRD, SEM and FTIR were used to characterize and confirm the prepared. The SNPs, generated from aqueous, ethanol, and methanol extracts, had an average diameter of 20.77, 18.53, and 14.27 nm, with spherical, rectangular, and cubical shapes, respectively. The SNPs demonstrated a remarkable potential to scavenge free radicals compared to the well-known antioxidant ascorbic acid, notably those produced from the antioxidant-rich methanol extract of A. millefolium. With an IC50 of 7.03 ± 0.31 µg/mL, the SNPs made from methanol extract had the maximum potential to scavenge DPPH radicals, while ascorbic acid had an IC50 of 4.29 ± 1.74 µg/mL [64].
Kiran et al. synthesized silver nanoparticles (AgNPs) with Eucalyptus tereticornis leaves extract as a reducing agent and measured antioxidant activity with the DPPH method. The produced AgNPs were confirmed by UV-vis, XRD, SEM with EDS, and TEM. The TEM picture of AgNPs taken at various magnifications reveals that the particles are nanoscale and homogenous. The nanoparticles generated were spherical in shape, with a size of 24 to 54 nm. The synthesized AgNPs showed remarkable free radical scavenging activity with an IC50 of 59.0 µg/mL [65].
Copper nanoparticles (CuNPs) were produced by Hasheminya and Dehghannya using an aqueous extract of E. caucasicum Trautv leaves. UV-vis, FTIR, XRD, and SEM were used to characterize the formed nanoparticles. The SEM demonstrated the formation of spherical copper nanoparticles with diameters lower than 40 nm. CuNPs demonstrated significant antioxidant properties in total phenolic compounds, total flavonoids, and free radical scavenging activity. E. caucasicum Trautv’s aqueous extract demonstrated a greater percentage of DPPH free radical scavenging activity (58.98%) than CuNPs [66].
3.4 Anticancer Activity of Nanoparticles
Ibrahim et al. used Fe2+, Cu2+, Zn2+, and Ag+ ions to form metal nanoparticles from rhus and safflower extracts. AgNPs from rhus extract varied in size from 22.41 to 37.58 nm, according to the TEM. Furthermore, the safflower AgNPs were spherical with low amounts of tetragonal particles and varied in size from 14.52 to 35.77 nm. AgNPs nanoparticles from rhus extract seemed to be more aggregated than AgNPs particles from safflower. The cytotoxic activity of the nanoparticles generated was tested on three cancer cell lines. CuNP safflower nanoparticles had the best anticancer activity (98.94% against T47D, 97.68% against HEPG2, and 89.33% against CaCo−2) [67].
Ansar et al. studied the antibacterial, anticancer, and antioxidant activities of silver nanoparticles made from Brassica oleracea (BO). UV-vs, particle size analysis, electrokinetic/zeta potential analysis, and TEM were used to investigate the properties of produced BO-AgNPs. A pronounced absorbance maximum verified the synthesis of BO-AgNPs at 400 nm. TEM analysis revealed that the produced nanoparticles were primarily spherical with an average diameter of 20 nm, with a relatively homogenous distribution. The cytotoxic activity of the BO-AgNPs was tested against MCF-7 cells. The cytotoxicity of the green-produced BO-AgNPs increased proportionally with increasing concentration, with the highest effect at 100 μg/ml and an IC50 of 55 μg/ml [68].
Hajebi et al. exploited rapeseed flower pollen (RFP) water extract to produce silver nanoparticles (RFP/Ag-NPs). They investigated their cytotoxicity against MDA-MB-231 and MCF7 cancer cell lines and HDF, a healthy human skin fibroblast. According to the FTIR results, the average size of RFP/Ag-NPs is 24 nm, with a peak at 430 nm and a spherical shape. RFP/Ag-NPs show cytotoxic effects on MDA-MB-231 and MCF7 cells and reduce malignant cell viability (IC50 = 3 µg/ml and 2 µg/ml, respectively) [69].
A. lebbeck stem bark extract was used by Umar et al. to synthesize ZnO NPs; FTIR investigations showed that the extract has reducing and stabilizing properties. SEM was used to detect many agglomerated particles with an average size of 66.25 nm and an atypical hexagonal shape. The hexagonal wurtzite structure was made clear by the XRD spectrum. The cytotoxicity of ZnO NPs was examined using the MDA-MB 231 and MCF-7 cell lines. The outcomes demonstrated that the produced ZnO NPs dramatically decreased the viability of MDA-MB 231 cells by 53.6%, 60.1%, and 66.2% for concentrations higher than the control [70].
Using an aqueous leaf extract of Pouteria campechiana, Narayanan et al. investigated the larvicidal and pupicidal effects of titanium dioxide (TiO2) nanoparticles (TiO2 NPs) against Aedes aegypti. The UV-vis spectrum analysis showed that the plant extract produced TiO2 NPs with a clear peak at 320 nm. The resultant TiO2 NPs were spherical in the SEM, and the XRD spectrum analysis revealed five different diffraction patterns. Alcohol was identified as a significant signal in the FTIR study at 1052.41 cm−1, indicating metal reduction. The EDX analysis, which shows a signal of about 58.44% and accounts for the remaining percentages of Ca, Al, and Mg, confirms the decrease in Ti from TiO2 NPs. At 900 µg/mL concentration, TiO2 NPs exhibited excellent lethality [71].
The bio-efficacy of Pimenta dioica leaf-derived silver nanoparticles (Pd@AgNPs) and leaf extract obtained using various solvents against malaria, filarial, and dengue vector larvae were studied by Kumar et al. UV-vs, XRD, SEM, and TEM were used to confirm that Pd@AgNPs. The TEM revealed that the synthesized Pd@AgNPs were triangular and spherical, with an average size of 20–40 nm. After 72 h of exposure, Pd@AgNPs were shown to have potential larvicidal efficacy against Aedes aegypti (LC50, 2.605; LC90, 5.084 ppm), Anopheles stephensi (LC50, 3.269; LC90, 7.790 ppm), and Culex quinquefasciatus (LC50, 5.373; LC90, 14.738 ppm). This research focuses on the green chemistry that goes into the synthesis of AgNPs, which can be used to control mosquitos and for other medicinal purposes [72].
Johnson et al. used Uvaria chamae leaf extract and an aqueous solution of (0.01 M) iron (III) chloride to create iron nanoparticles (FeNPs), which they then tested for larvicidal activity on Culex quinquefasciatus and Anopheles gambae second instar mosquito larvae. FeNPs were observed using SEM and TEM, with an average particle size of 40.4 nm. FeNPs showed larvicidal efficacy against C. quinquefasciatus and A. gambae second instar larvae in a mosquito bioassay. The findings show that FeNPs were quite effective, killing 100% of the larvae within 24 h of exposure and might be used to control mosquitoes [73].
3.6 Anti-Diabetic Activity of Nanoparticles
Sati et al. published on the anti-diabetic effects of produced metal nanoparticles (AgNPs and ZnONPs) using green leaf extract of the Bedu (Ficus palmata) tree as a reducing agent and the support of natural fibers. UV-vs, XRD, SEM, EDAX, TEM, and FTIR were used to examine the obtained AgNPs and ZnONPs. While the hexagonal wurtzite-structured ZnONPs seemed to be deformed spheres with an average particle size of 34 nm, the leaves-mediated metal nanoparticles were polydispersed and spherical AgNPs with an average particle size of 30 nm. The synthesized AgNPs and ZnONPs exhibited potent activity. It was revealed that suppressing α-amylases and α-glucosidases have in vitro anti-diabetic properties [74].
Vinotha et al. produced ZnO nanoparticles from the leaf extract of Costus igneus, which were then examined using UV-vs, FTIR, XRD, and TEM. Ci-ZnO NPs were detected using UV-vis, and a peak at 365 nm was found. The hexagonal wurtzite generated by the Ci-ZnO NPs was crystallized, as seen by the XRD spectrum. The presence of possible functional groups in Ci-ZnO NPs was discovered via the FTIR spectra. A hexagonal structure with a size of 26.55 nm was visible in the TEM image and is typical of Ci-ZnO NPs. Ci-ZnO NPs demonstrated anti-diabetic activity, as evidenced by the investigations on the suppression of glucosidase and amylase (74% and 82%, respectively) [75].
Zn-doped Catharanthus roseus nanoparticles were synthesized by Govindan and colleagues and assessed their anti-diabetic efficiency. Clusters of spherical NPs were visible in the TEM image. The results demonstrated that the biosynthesized NPs had multi-dispersed particle sizes between 10 and 20 nm. This work investigated the biosynthesized NPs for potential medical use as anti-diabetic drugs. The Zn-doped C. roseus NPs displayed good-amylase inhibitory efficacy compared to the standard drug (Acarbose). The Zn-doped C. roseus NPs showed good-amylase inhibitory activity [76].
Inam et al. synthesized Ag-NPs from the roots of Pueraria Lobata, and the UV-vis of Ag-NPs indicated the synthesis with a high peak at 248 nm; TEM revealed the spherical shape of Ag-NPs with sizes ranging from 30 to 60 nm. The anti-diabetic activity of Ag-NPs was tested in vitro against the carbohydrate-digesting enzyme-amylase. The findings suggest that Ag-NPs have a high potential for anti-diabetic activity against the major enzyme in diabetes and are suited for nano bio-medical applications [77].
Rajakumar et al., bio-mediated synthesis of zinc oxide nanoparticles (ZnONPs) using Andrographis paniculata leaf extract’s reducing and capping capabilities. UV-vis, XRD, FTIR, SEM, TEM, and SAED techniques were used to characterize the capped ZnONPs. The relevance of phenolic chemicals, terpenoids, and proteins in the nucleation and stability of ZnONPs was proposed by FTIR analysis of A. paniculata leaf extract. In comparison to the conventional spectrum, the XRD pattern demonstrated that the zinc oxide particles generated in the current investigations were nanocrystals. SEM and TEM analysis reveal spherical and hexagonal ZnONPs with sizes ranging from 96–115 and 570.3 nm, respectively. The synthesized NPs inhibited-amylase moderately, and the results are reported as IC50 values. The IC50 values of ZnONPs were lower than those of A. paniculata leaf extract, ZnNO3, indicating that ZnONPs had more anti-diabetic potential in-amylase inhibitory action [78].
Lava et al. utilized Justica wynaadensis leaf extract to synthesize silver nanoparticles. The leaf extract solution reduced and stabilized AgNO3 to form Silver nanoparticles. The nanoparticles were analyzed using XRD, TEM, and FTIR. As per TEM analysis, the silver nanoparticles had a crystalline structure with diameters ranging from 30 to 50 nm. The inhibitory effects of-amylase were compared to that of metformin, a commonly used diabetes treatment. The Ag nanoparticles’ IC50 value was 493.87 µg/mL [79].
Nanotechnology has key features owing to the green synthesis of nanoparticles and its unparalleled uses. Plants can be more advantageous than other biological entities for the synthesis of nanoparticles because they can prevent the time-consuming procedure of using microbes and maintaining their culture, which can result in the loss of their potential for the synthesis of nanoparticles. Pharmaceutically valuable phytometabolites are present in medicinal plants, which are now used for human benefit. This method has been shown to be successful and ecologically benign for the production of metal nanoparticles. Our review paper investigated the production of metallic nanoparticles from several medicinal plants and their potential medical applications. The production of metallic nanoparticles using plant extracts is simple, efficient, inexpensive, easily scaled up, ecologically benign, and uses less hazardous materials while increasing process efficiency.
However, procedures must be improved further in order for these approaches to be cost-effective and comparable to established methods for large-scale nanoparticle production. The discovery of more stable and environmentally benign methods for generating metallic nanoparticles represents a significant step forward in applied nanotechnology.
Additionally, most of these approaches are still in the early phases of development, and challenges must be overcome. These are examples of nanoparticle stability, aggregation, crystal formation, shape, and size management. The separation and purification of nanoparticles is another critical component that has to be investigated further. Metal nanoparticles generated by plants and/or plant extracts are more stable than other microorganisms. The possibilities of nanotechnology are emerging in more new disciplines, such as DNA nanotechnology, which may aid in decreasing medication toxicity and enhancing therapeutic targeting efficacy. This brief review, in our opinion, will aid researchers in investigating the possible long-term benefits of metallic nanoparticles produced by biosynthesis.
Acknowledgement: The authors would like to thank KwaZulu Natal University for their support.
Funding Statement: The authors received no specific funding for this study.
Conflicts of Interest: The authors declare that they have no conflicts of interest to report regarding the present study.
References
1. Esa, Y. A. M., Sapawe, N. (2020). A short review on biosynthesis of cobalt metal nanoparticles. Materials Today: Proceedings, 31, 378–385. [Google Scholar]
2. Rabiee, N., Bagherzadeh, M., Kiani, M., Ghadiri, A. M., Etessamifar, F. et al. (2020). Biosynthesis of copper oxide nanoparticles with potential biomedical applications. International Journal of Nanomedicine, 15, 3983. [Google Scholar] [PubMed]
3. Srivastava, S., Usmani, Z., Atanasov, A. G., Singh, V. K., Singh, N. P. et al. (2021). Biological nanofactories: Using living forms for metal nanoparticle synthesis. Mini Reviews in Medicinal Chemistry, 21, 245–265. [Google Scholar] [PubMed]
4. Saravanan, A., Kumar, P. S., Karishma, S., Vo, D. V. N., Jeevanantham, S. et al. (2020). A review on biosynthesis of metal nanoparticles and its environmental applications. Chemosphere, 264, 128580. [Google Scholar] [PubMed]
5. Goutam, S. P., Saxena, G., Roy, D., Yadav, A. K., Bharagava, R. N. (2020). Green synthesis of nanoparticles and their applications in water and wastewater treatment. In: Bioremediation of industrial waste for environmental safety, pp. 349–379. Singapore: Springer. [Google Scholar]
6. Yew, Y. P., Shameli, K., Miyake, M., Khairudin, N. B. B. A., Mohamad, S. E. B. et al. (2020). Green biosynthesis of superparamagnetic magnetite Fe3O4 nanoparticles and biomedical applications in targeted anticancer drug delivery system: A review. Arabian Journal of Chemistry, 13(1), 2287–2308. [Google Scholar]
7. Rafique, M., Sadaf, I., Rafique, M. S., Tahir, M. B. (2017). A review on green synthesis of silver nanoparticles and their applications. Artificial Cells, Nanomedicine, and Biotechnology, 45(7), 1272–1291. https://doi.org/10.1080/21691401.2016.1241792 [Google Scholar] [PubMed] [CrossRef]
8. Jadoun, S., Arif, R., Jangid, N. K., Meena, R. K. (2021). Green synthesis of nanoparticles using plant extracts: A review. Environmental Chemistry Letters, 19(1), 355–374. https://doi.org/10.1007/s10311-020-01074-x [Google Scholar] [CrossRef]
9. Chandra, H., Kumari, P., Bontempi, E., Yadav, S. (2020). Medicinal plants: Treasure trove for green synthesis of metallic nanoparticles and their biomedical applications. Biocatalysis and Agricultural Biotechnology, 24(1), 101518. https://doi.org/10.1016/j.bcab.2020.101518 [Google Scholar] [CrossRef]
10. Rana, A., Yadav, K., Jagadevan, S. (2020). A comprehensive review on green synthesis of nature-inspired metal nanoparticles: Mechanism, application and toxicity. Journal of Cleaner Production, 272(5), 122880. https://doi.org/10.1016/j.jclepro.2020.122880 [Google Scholar] [CrossRef]
11. Singh, A., Gautam, P. K., Verma, A., Singh, V., Shivapriya, P. M. et al. (2020). Green synthesis of metallic nanoparticles as effective alternatives to treat antibiotics resistant bacterial infections: A review. Biotechnology Reports, 25(1), e00427. https://doi.org/10.1016/j.btre.2020.e00427 [Google Scholar] [PubMed] [CrossRef]
12. Asiegbu, F. O., Kovalchuk, A. (2021). An introduction to forest biome and associated microorganisms. Forest Microbiology, 1(2), 3–16. https://doi.org/10.1016/B978-0-12-822542-4.00009-7 [Google Scholar] [CrossRef]
13. Slavin, Y. N., Asnis, J., Hafeli, U. O., Bach, H. (2017). Metal nanoparticles: Understanding the mechanisms behind antibacterial activity. Journal of Nanobiotechnology, 15(1), 1–20. https://doi.org/10.1186/s12951-017-0308-z [Google Scholar] [PubMed] [CrossRef]
14. Kaweeteerawat, C., Na Ubol, P., Sangmuang, S., Aueviriyavit, S., Maniratanachote, R. (2017). Mechanisms of antibiotic resistance in bacteria mediated by silver nanoparticles. Journal of Toxicology and Environmental Health, Part A, 80(23–24), 1276–1289. https://doi.org/10.1080/15287394.2017.1376727 [Google Scholar] [PubMed] [CrossRef]
15. Dastan, D., Salehi, P., Aliahmadi, A., Gohari, A. R., Maroofi, H. et al. (2016). New coumarin derivatives from ferula pseudalliacea with antibacterial activity. Natural Product Research, 30(24), 2747–2753. https://doi.org/10.1080/14786419.2016.1149705 [Google Scholar] [PubMed] [CrossRef]
16. Dizaj, S. M., Lotfipour, F., Barzegar-Jalali, M., Zarrintan, M. H., Adibkia, K. (2014). Antimicrobial activity of the metals and metal oxide nanoparticles. Materials Science and Engineering: C, 44, 278–284. https://doi.org/10.1016/j.msec.2014.08.031 [Google Scholar] [PubMed] [CrossRef]
17. Pal, G., Rai, P., Pandey, A. (2019). Green synthesis of nanoparticles: A greener approach for a cleaner future. Green synthesis, characterization and applications of nanoparticles. Micro and Nano Technologies, 2019, 1–26. [Google Scholar]
18. Paulkumar, K., Rajeshkumar, S., Gnanajobitha, G., Vanaja, M., Malarkodi, C. et al. (2013). Biosynthesis of silver chloride nanoparticles using Bacillus subtilis MTCC 3053 and assessment of its antifungal activity. International Scholarly Research Notices, 2013, 1–8. [Google Scholar]
19. Aswani, T., Reshmi, S., Suchithra, T. (2019). Actinomycetes: Its realm in nanotechnology. Microbial Nanobionics, 1, 127–140. [Google Scholar]
20. Saleem, A. M., Rajasekar, S., Kaviyarasu, K., Perumalsamy, R., Ayeshamariam, A. et al. (2019). Green combustion synthesis of CeO2 and TiO2 nanoparticles doped with same oxide materials of ZrO2: Investigation of in vitro assay with antibiotic resistant bacterium (ARB) and anticancer effect. European Journal of Medicinal Plants, 30(2), 1–17. [Google Scholar]
21. Abdeen, S., Geo, S., Praseetha, P., Dhanya, R. (2014). Biosynthesis of silver nanoparticles from actinomycetes for therapeutic applications. International Journal of Nano Dimension, 5(2), 155–162. [Google Scholar]
22. Karmous, I., Pandey, A., Haj, K. B., Chaoui, A. (2020). Efficiency of the green synthesized nanoparticles as new tools in cancer therapy: Insights on plant-based bioengineered nanoparticles, biophysical properties, and anticancer roles. Biological Trace Element Research, 196(1), 330–342. https://doi.org/10.1007/s12011-019-01895-0 [Google Scholar] [PubMed] [CrossRef]
23. Gupta, A., Singh, D., Singh, S. K., Singh, V. K., Singh, A. V. et al. (2019). Role of actinomycetes in bioactive and nanoparticle synthesis. Role of Plant Growth Promoting Microorganisms in Sustainable Agriculture and Nanotechnology, 2019, 163–182. [Google Scholar]
24. Manimaran, M., Kannabiran, K. (2017). Actinomycetes-mediated biogenic synthesis of metal and metal oxide nanoparticles: Progress and challenges. Letters in Applied Microbiology, 64(6), 401–408. https://doi.org/10.1111/lam.12730 [Google Scholar] [PubMed] [CrossRef]
25. Salem, S. S., Fouda, A. (2021). Green synthesis of metallic nanoparticles and their prospective biotechnological applications: An overview. Biological Trace Element Research, 199(1), 344–370. https://doi.org/10.1007/s12011-020-02138-3 [Google Scholar] [PubMed] [CrossRef]
26. Nabila, M. I., Kannabiran, K. (2018). Biosynthesis, characterization and antibacterial activity of copper oxide nanoparticles (CuONPs) from actinomycetes. Biocatalysis and Agricultural Biotechnology, 15, 56–62. https://doi.org/10.1016/j.bcab.2018.05.011 [Google Scholar] [CrossRef]
27. Ranjitha, V., Rai, V. R. (2017). Actinomycetes mediated synthesis of gold nanoparticles from the culture supernatant of streptomyces griseoruber with special reference to catalytic activity. 3 Biotech, 7(5), 1–7. https://doi.org/10.1007/s13205-017-0930-3 [Google Scholar] [PubMed] [CrossRef]
28. Kumari, S., Tehri, N., Gahlaut, A., Hooda, V. (2020). Actinomycetes mediated synthesis, characterization, and applications of metallic nanoparticles. Inorganic and Nano-Metal Chemistry, 51, 1386–1395. [Google Scholar]
29. Hassan, S. E. D., Fouda, A., Radwan, A. A., Salem, S. S., Barghoth, M. G. et al. (2019). Endophytic actinomycetes streptomyces spp mediated biosynthesis of copper oxide nanoparticles as a promising tool for biotechnological applications. Journal of Biological Inorganic Chemistry, 24, 377–393. [Google Scholar] [PubMed]
30. Bhosale, R. S., Hajare, K. Y., Mulay, B., Mujumdar, S., Kothawade, M. et al. (2015). Biosynthesis, characterization and study of antimicrobial effect of silver nanoparticles by Actinomycetes spp. International Journal of Current Microbiology and Applied Sciences, 2, 144–151. [Google Scholar]
31. Uzair, B., Liaqat, A., Iqbal, H., Menaa, B., Razzaq, A. et al. (2020). Green and cost-effective synthesis of metallic nanoparticles by algae: Safe methods for translational medicine. Bioengineering, 7(4), 129. [Google Scholar] [PubMed]
32. Chaudhary, R., Nawaz, K., Khan, A. K., Hano, C., Abbasi, B. H. et al. (2020). An overview of the algae-mediated biosynthesis of nanoparticles and their biomedical applications. Biomolecules, 10, 1498. [Google Scholar] [PubMed]
33. Khanna, P., Kaur, A., Goyal, D. (2019). Algae-based metallic nanoparticles: Synthesis, characterization and applications. Journal of Microbiological Methods, 163, 105656. [Google Scholar] [PubMed]
34. Bhattacharya, P., Swarnakar, S., Ghosh, S., Majumdar, S., Banerjee, S. et al. (2019). Disinfection of drinking water via algae mediated green synthesized copper oxide nanoparticles and its toxicity evaluation. Journal of Environmental Chemical Engineering, 7, 102867. [Google Scholar]
35. Li, S. N., Wang, R., Ho, S. H. (2021). Algae-mediated biosystems for metallic nanoparticle production: From synthetic mechanisms to aquatic environmental applications. Journal of Hazardous Materials, 420(15), 126625. [Google Scholar] [PubMed]
36. Sharma, D., Kanchi, S., Bisetty, K. (2019). Biogenic synthesis of nanoparticles: A review. Arabian Journal of Chemistry, 12, 3576–3600. [Google Scholar]
37. Akther, T., Mathipi, V., Kumar, N. S., Davoodbasha, M., Srinivasan, H. et al. (2019). Fungal-mediated synthesis of pharmaceutically active silver nanoparticles and anticancer property against A549 cells through apoptosis. Environmental Science and Pollution Research, 26, 13649–13657. [Google Scholar] [PubMed]
38. Kadam, V. V., Ettiyappan, J. P., Balakrishnan, R. M. (2019). Mechanistic insight into the endophytic fungus mediated synthesis of protein capped ZnO nanoparticles. Materials Science and Engineering: B, 243(5), 214–221. https://doi.org/10.1016/j.mseb.2019.04.017 [Google Scholar] [CrossRef]
39. Guilger-Casagrande, M., Lima, L. D. (2019). Synthesis of silver nanoparticles mediated by fungi: A review. Frontiers in Bioengineering and Biotechnology, 7, 287. https://doi.org/10.3389/fbioe.2019.00287 [Google Scholar] [PubMed] [CrossRef]
40. Molnar, Z., Bodai, V., Szakacs, G., Erdelyi, B., Fogarassy, Z. et al. (2018). Green synthesis of gold nanoparticles by thermophilic filamentous fungi. Scientific Reports, 8(1), 1–12. https://doi.org/10.1038/s41598-018-22112-3 [Google Scholar] [PubMed] [CrossRef]
41. Xiao, A., Xu, C., Lin, Y., Ni, H., Zhu, Y. et al. (2016). Preparation and characterization of κ-carrageenase immobilized onto magnetic iron oxide nanoparticles. Electronic Journal of Biotechnology, 19, 1–7. https://doi.org/10.1016/j.ejbt.2015.10.001 [Google Scholar] [CrossRef]
42. Sundrarajan, M., Ambika, S., Bharathi, K. (2015). Plant-extract mediated synthesis of ZnO nanoparticles using pongamia pinnata and their activity against pathogenic bacteria. Advanced Powder Technology, 26(5), 1294–1299. https://doi.org/10.1016/j.apt.2015.07.001 [Google Scholar] [CrossRef]
43. Ebrahiminezhad, A., Zare-Hoseinabadi, A., Sarmah, A. K., Taghizadeh, S., Ghasemi, Y. (2018). Plant-mediated synthesis and applications of iron nanoparticles. Molecular Biotechnology, 60(2), 154–168. https://doi.org/10.1007/s12033-017-0053-4 [Google Scholar] [PubMed] [CrossRef]
44. Kuunal, S., Rauwel, P., Rauwel, E. (2018). Plant extract mediated synthesis of nanoparticles. Emerging Applications of Nanoparticles and Architecture Nanostructures, 2018, 411–466. [Google Scholar]
45. Lakshmanan, G., Sathiyaseelan, A., Kalaichelvan, P., Murugesan, K. (2018). Plant-mediated synthesis of silver nanoparticles using fruit extract of Cleome Viscosa L.: Assessment of their antibacterial and anticancer activity. Karbala International Journal of Modern Science, 4(1), 61–68. https://doi.org/10.1016/j.kijoms.2017.10.007 [Google Scholar] [CrossRef]
46. Makarov, V., Love, A., Sinitsyna, O., Makarova, S., Yaminsky, I. (2014). “Green” nanotechnologies: Synthesis of metal nanoparticles using plants. Acta Naturae, 6(1), 35–44. https://doi.org/10.32607/20758251-2014-6-1-35-44 [Google Scholar] [CrossRef]
47. Jagathesan, G., Rajiv, P. (2018). Biosynthesis and characterization of iron oxide nanoparticles using eichhornia crassipes leaf extract and assessing their antibacterial activity. Biocatalysis and Agricultural Biotechnology, 13, 90–94. https://doi.org/10.1016/j.bcab.2017.11.014 [Google Scholar] [CrossRef]
48. Sharmila, G., Muthukumaran, C., Sandiya, K., Santhiya, S., Pradeep, R. S. (2018). Biosynthesis, characterization, and antibacterial activity of zinc oxide nanoparticles derived from Bauhinia tomentosa leaf extract. Journal of Nanostructure in Chemistry, 8(3), 293–299. https://doi.org/10.1007/s40097-018-0271-8 [Google Scholar] [CrossRef]
49. Aziz, W. J., Abid, M. A., Hussein, E. H. (2020). Biosynthesis of CuO nanoparticles and synergistic antibacterial activity using mint leaf extract. Materials Technology, 35(8), 447–451. https://doi.org/10.1080/10667857.2019.1692163 [Google Scholar] [CrossRef]
50. Awwad, A., Amer, M. (2020). Biosynthesis of copper oxide nanoparticles using ailanthus altissima leaf extract and antibacterial activity. Chemistry International, 6(4), 210–217. [Google Scholar]
51. Rajeshkumar, S., Menon, S., Kumar, S. V., Tambuwala, M. M., Bakshi, H. A. et al. (2019). Antibacterial and antioxidant potential of biosynthesized copper nanoparticles mediated through Cissus arnotiana plant extract. Journal of Photochemistry and Photobiology B: Biology, 197, 111531. https://doi.org/10.1016/j.jphotobiol.2019.111531 [Google Scholar] [PubMed] [CrossRef]
52. Shirzadi-ahodashti, M., Mortazavi-Derazkola, S., Ebrahimzadeh, M. A. (2020). Biosynthesis of noble metal nanoparticles using crataegus monogyna leaf extract (CML@ X-NPs, X= Ag, AuAntibacterial and cytotoxic activities against breast and gastric cancer cell lines. Surfaces and Interfaces, 21, 100697. https://doi.org/10.1016/j.surfin.2020.100697 [Google Scholar] [CrossRef]
53. Shirzadi-Ahodashti, M., Ebrahimzadeh, M. A., Ghoreishi, S. M., Naghizadeh, A., Mortazavi-Derazkola, S. J. A. O. C. et al. (2020). Facile and eco-benign synthesis of a novel MnFe2O4@ SiO2@ Au magnetic nanocomposite with antibacterial properties and enhanced photocatalytic activity under UV and Visible-light irradiations. Applied Organometal Chemistry, 34, e5614. [Google Scholar]
54. Abdel-Azeem, A., Nada, A. A., O’Donovan, A., Thakur, V. K., Elkelish, A. J. J. O. R. M. et al. (2020). Mycogenic silver nanoparticles from endophytic trichoderma atroviride with antimicrobial activity. Journal of Renewable Materials, 8(2), 171–185. https://doi.org/10.32604/jrm.2020.08960 [Google Scholar] [CrossRef]
55. Aboutorabi, S. N., Nasiriboroumand, M., Mohammadi, P., Sheibani, H., Barani, H. J. J. O. R. M. et al. (2019). Preparation of antibacterial cotton wound dressing by green synthesis silver nanoparticles using mullein leaves extract. Journal of Renewable Materials, 7(8), 787–794. https://doi.org/10.32604/jrm.2019.06438 [Google Scholar] [CrossRef]
56. Solorzano-Toala, R., Gonzalez-Mendoza, D., Valdez-Salas, B., Mendez-Trujillo, V., Gutierrz-Miceli, F. et al. (2020). Green synthesis of silver nanoparticles using Annona diversifolia leaf extract and their antimicrobial application. Journal of Renewable Materials, 8(9), 1129–1137. https://doi.org/10.32604/jrm.2020.09845 [Google Scholar] [CrossRef]
57. Pagar, K., Ghotekar, S., Pagar, T., Nikam, A., Pansambal, S. et al. (2020). Antifungal activity of biosynthesized cuo nanoparticles using leaves extract of Moringa oleifera and their structural characterizations. Asian Journal of Nanosciences and Materials, 3, 15–23. [Google Scholar]
58. Pillai, A. M., Sivasankarapillai, V. S., Rahdar, A., Joseph, J., Sadeghfar, F. et al. (2020). Green synthesis and characterization of zinc oxide nanoparticles with antibacterial and antifungal activity. Journal of Molecular Structure, 1211(12), 128107. https://doi.org/10.1016/j.molstruc.2020.128107 [Google Scholar] [CrossRef]
59. Jamdagni, P., Khatri, P., Rana, J. (2018). Green synthesis of zinc oxide nanoparticles using flower extract of nyctanthes arbor-tristis and their antifungal activity. Journal of King Saud University-Science, 30(2), 168–175. https://doi.org/10.1016/j.jksus.2016.10.002 [Google Scholar] [CrossRef]
60. Jebril, S., Jenana, R. K. B., Dridi, C. (2020). Green synthesis of silver nanoparticles using Melia azedarach leaf extract and their antifungal activities: In vitro and in vivo. Materials Chemistry and Physics, 248, 122898. https://doi.org/10.1016/j.matchemphys.2020.122898 [Google Scholar] [CrossRef]
61. Dawoud, T. M., Yassin, M. A., El-samawaty, A. R. M., Elgorban, A. M. (2021). Silver nanoparticles synthesized by Nigrospora oryzae showed antifungal activity. Saudi Journal of Biological Sciences, 28(3), 1847–1852. https://doi.org/10.1016/j.sjbs.2020.12.036 [Google Scholar] [PubMed] [CrossRef]
62. Das, P. E., Abu-Yousef, I. A., Majdalawieh, A. F., Narasimhan, S., Poltronieri, P. et al. (2020). Green synthesis of encapsulated copper nanoparticles using a hydroalcoholic extract of Moringa oleifera leaves and assessment of their antioxidant and antimicrobial activities. Molecules, 25(3), 555. https://doi.org/10.3390/molecules25030555 [Google Scholar] [PubMed] [CrossRef]
63. Sharmila, G., Thirumarimurugan, M., Muthukumaran, C. (2019). Green synthesis of ZnO nanoparticles using Tecoma castanifolia leaf extract: Characterization and evaluation of its antioxidant, bactericidal and anticancer activities. Microchemical Journal, 145, 578–587. https://doi.org/10.1016/j.microc.2018.11.022 [Google Scholar] [CrossRef]
64. Yousaf, H., Mehmood, A., Ahmad, K. S., Raffi, M. (2020). Green synthesis of silver nanoparticles and their applications as an alternative antibacterial and antioxidant agents. Materials Science and Engineering: C, 112, 110901. [Google Scholar] [PubMed]
65. Kiran, M., Betageri, V. S., Kumar, C., Vinay, S., Latha, M. (2020). In-vitro antibacterial, antioxidant and cytotoxic potential of silver nanoparticles synthesized using novel Eucalyptus tereticornis leaves extract. Journal of Inorganic and Organometallic Polymers and Materials, 30(8), 2916–2925. https://doi.org/10.1007/s10904-020-01443-7 [Google Scholar] [CrossRef]
66. Hasheminya, S. M., Dehghannya, J. (2020). Green synthesis and characterization of copper nanoparticles using Eryngium caucasicum trautv aqueous extracts and its antioxidant and antimicrobial properties. Particulate Science and Technology, 38(8), 1019–1026. https://doi.org/10.1080/02726351.2019.1658664 [Google Scholar] [CrossRef]
67. Ibrahim, F. Y., El-khateeb, A. Y., Mohamed, A. H. (2019). Rhus and safflower extracts as potential novel food antioxidant, anticancer, and antimicrobial agents using nanotechnology. Foods, 8(4), 139. https://doi.org/10.3390/foods8040139 [Google Scholar] [PubMed] [CrossRef]
68. Ansar, S., Tabassum, H., Aladwan, N. S., Ali, M. N., Almaarik, B. et al. (2020). Eco friendly silver nanoparticles synthesis by Brassica oleracea and its antibacterial, anticancer and antioxidant properties. Scientific Reports, 10(1), 1–12. https://doi.org/10.1038/s41598-020-74371-8 [Google Scholar] [PubMed] [CrossRef]
69. Hajebi, S., Tabrizi, M. H., Moghaddam, M. N., Shahraki, F., Yadamani, S. (2019). Rapeseed flower pollen bio-green synthesized silver nanoparticles: A promising antioxidant, anticancer and antiangiogenic compound. Journal of Biological Inorganic Chemistry, 24(3), 395–404. https://doi.org/10.1007/s00775-019-01655-4 [Google Scholar] [PubMed] [CrossRef]
70. Umar, H., Kavaz, D., Rizaner, N. (2019). Biosynthesis of zinc oxide nanoparticles using albizia lebbeck stem bark, and evaluation of its antimicrobial, antioxidant, and cytotoxic activities on human breast cancer cell lines. International Journal of Nanomedicine, 14, 87–100. https://doi.org/10.2147/IJN.S186888 [Google Scholar] [PubMed] [CrossRef]
71. Narayanan, M., Devi, P. G., Natarajan, D., Kandasamy, S., Devarayan, K. et al. (2021). Green synthesis and characterization of titanium dioxide nanoparticles using leaf extract of Pouteria campechiana and larvicidal and pupicidal activity on Aedes aegypti. Environmental Research, 200, 111333. https://doi.org/10.1016/j.envres.2021.111333 [Google Scholar] [PubMed] [CrossRef]
72. Kumar, D., Kumar, P., Vikram, K., Singh, H. (2021). Fabrication and characterization of noble crystalline silver nanoparticles from Pimenta dioica leave extract and analysis of chemical constituents for larvicidal applications. Saudi Journal of Biological Sciences, 29(2), 1134–1146. https://doi.org/10.1016/j.sjbs.2021.09.052 [Google Scholar] [PubMed] [CrossRef]
73. Johnson, A., Uwa, P. (2019). Eco-friendly synthesis of iron nanoparticles using Uvaria chamae: Characterization and biological activity. Inorganic and Nano-Metal Chemistry, 49(12), 431–442. https://doi.org/10.1080/24701556.2019.1661448 [Google Scholar] [CrossRef]
74. Sati, S. C., Kour, G., Bartwal, A. S., Sati, M. D. (2020). Biosynthesis of metal nanoparticles from leaves of Ficus palmata and evaluation of their anti-inflammatory and anti-diabetic activities. Biochemistry, 59(33), 3019–3025. https://doi.org/10.1021/acs.biochem.0c00388 [Google Scholar] [PubMed] [CrossRef]
75. Vinotha, V., Iswarya, A., Thaya, R., Govindarajan, M., Alharbi, N. S. et al. (2019). Synthesis of ZnO nanoparticles using insulin-rich leaf extract: anti-diabetic, antibiofilm and antioxidant properties. Journal of Photochemistry and Photobiology B: Biology, 197, 111541. [Google Scholar] [PubMed]
76. Govindan, N., Vairaprakasam, K., Chinnasamy, C., Sivalingam, T., Mohammed, M. K. (2020). Green synthesis of Zn-doped Catharanthus Roseus nanoparticles for enhanced anti-diabetic activity. Materials Advances, 1, 3460–3465. [Google Scholar]
77. Inam, M., Shah, A., Khan, W. N., Sharif, S., Saqib, N. U. (2021). Biosynthesis of silver nanoparticles: Preparation, optimization and in vitro anti-diabetic effect. BioNanoScience, 11, 1154–1159. [Google Scholar]
78. Rajakumar, G., Thiruvengadam, M., Mydhili, G., Gomathi, T., Chung, I. M. (2018). Green approach for synthesis of zinc oxide nanoparticles from Andrographis paniculata leaf extract and evaluation of their antioxidant, anti-diabetic, and anti-inflammatory activities. Bioprocess and Biosystems Engineering, 41, 21–30. [Google Scholar] [PubMed]
79. Lava, M., Muddapur, U. M., Basavegowda, N., More, S. S., More, V. S. (2021). Characterization, anticancer, antibacterial, anti-diabetic and anti-inflammatory activities of green synthesized silver nanoparticles using Justica wynaadensis leaves extract. Materials Today: Proceedings, 46, 5942–5947. [Google Scholar]
Cite This Article
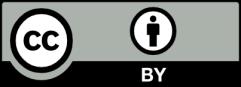