Open Access
ARTICLE
Urban Plant Biomass Residues from the Neotropics and Their Potential for Thermal Energy Generation
1
Competitiveness and Sustainability for Development Research Group, Faculty of Engineering, Universidad Libre-Cúcuta Sectional,
Cúcuta, Colombia
2
Catalysis and Nanomaterials Research Group, Faculty of Mines, Universidad Nacional de Colombia-Medellín Sectional, Medellín,
Colombia
3
Detecal Research Group, Faculty of Engineering, Universidad Libre-Bogotá Sectional, Bogotá, Colombia
* Corresponding Author: John Freddy Gelves Díaz. Email:
Journal of Renewable Materials 2023, 11(9), 3547-3566. https://doi.org/10.32604/jrm.2023.029267
Received 10 February 2023; Accepted 11 April 2023; Issue published 20 July 2023
Abstract
The material associated with tree pruning in a city in the Colombian neotropics was characterized in order to determine its energy potential. The species studied for their relevance in the territory were Prosopis juliflora, Licania tomentosa, Terminalia catappa, Azadirachta indica, Pithecellobium dulce, Ficus benjamina and Leucaena leucocephala. Moisture content, bulk density, hygroscopic response, elemental chemical analysis, proximal analysis, calorific value, ease of ignition and combustion, thermogravimetric and heat flow analysis, steam generation capacity, and qualitative analysis of gases (mass spectrometry) were determined. The results that were obtained show high initial moisture contents that vary between 37% and 67% and a variable density (when dry) between 0.21 and 0.41 g/cm3 . Chemically, it was shown that all residues have lower carbon and sulfur content compared to a reference mineral coal. However, the residues of some species have higher nitrogen contents compared to the same coal. All biomasses are characterized by their high content of volatile fractions and by having a lower content of inorganic matter compared to carbon. The lower calorific values of these residues are between 14170 and 16928 kJ/kg, which are not negligible compared to other biomasses. This characteristic would be related to the high presence of hemicellulose in the residues. Flue gas monitoring revealed that there are different airflow needs in order to ensure complete combustion. Steam generation tests showed that the calorific value should not be the most relevant criterion to establish the potential use of the waste, since leucaena leucocephala, despite having the highest calorific value, was the material with the lowest performance in steam generation.Graphical Abstract
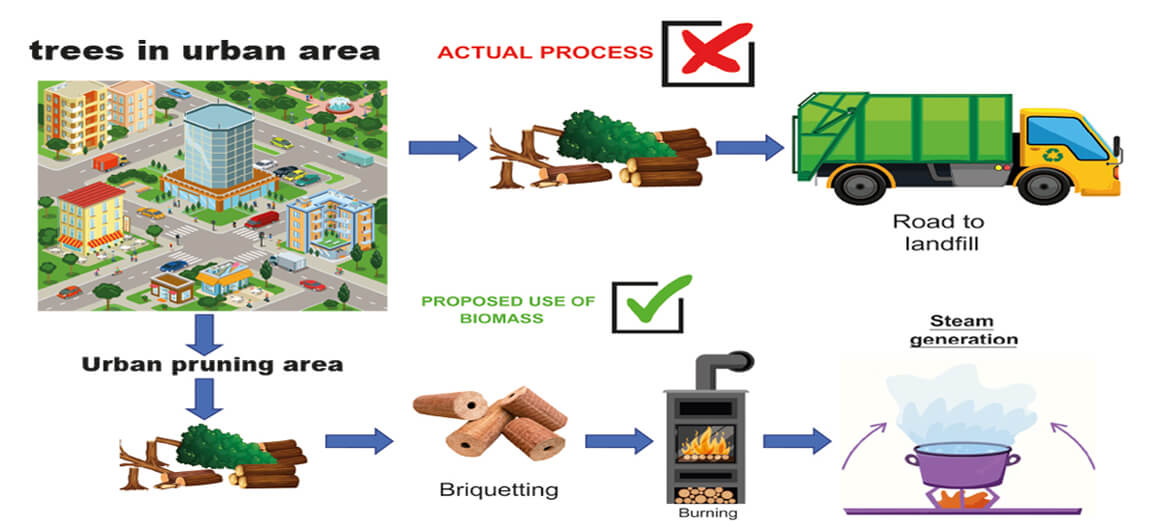
Keywords
Due to the growth of the world population, energy needs have also increased significantly [1,2]. In order to satisfy this energy demand, society has relied significantly on fossil fuels through the combustion process [3,4], which has brought with it the release of very significant amounts of carbon dioxide into the earth’s atmosphere, a gas that has been considered as the main cause for the phenomenon of global warming [2,4].
Due to this situation, the academic and business community has been making great efforts in research and development related to the use of alternative energies. Significant improvements have been achieved in terms of solar (photovoltaic and solar thermal), hydraulic, wind, geothermal, tidal energy, and use of energy contained in plant and animal biomasses [5–9]. In the latter case, plant biomass has been booming due to the high availability of the resource in most territories, its low acquisition cost, reduction of visual pollution problems, bad odors, and generation of vectors (health), and especially because it is considered a neutral fuel due to the carbon cycle of the plant, since the same plant can capture the carbon dioxide generated during the combustion of the vegetable residue to give growth to new branches and fruits [10–12].
Around the world, a large number of studies have been carried out that seek to take advantage of the energy contained in biomass, highlighting the processes of extraction, fermentation, direct combustion, pyrolysis, thermal gasification, and biotechnological processes [11,13,14]. Among the biomasses that stand out in scientific literature are those of the primary type, cultivated for energy purposes, whether they are forestry (lignocellulosic), such as coniferous species, eucalyptus, paulownia, or agro-industrial species, either for oilseed or alcohol use purposes [15]. There is also secondary or residual biomass from the agroindustry, such as sugarcane bagasse, rice husks, bananas, orujillo, cereal residues, forest residues (pruning and wood processing), as well as urban solid residues [15].
Within the group of secondary biomasses, lignocellulosic residues from urban pruning can become very significant within the energy matrix of cities if they are used well [16,17]. However, when reviewing the scientific literature, it is evident that academic studies on the use of pruning residues as an energy source are scarce, with Brazil (Sao Paulo, Piracicaba, and Pato Branco) [17–20] as the territory where the subject has been studied most. Some works are also evidenced in the cities of Mexico (Mérida) [21], Ecuador (Guayaquil) [22], Italy (Rome) [23], Spain (Madrid) [24], and the United States (Woodward) [25]. Many of these works are of a theoretical type, exploring the energy, economic, and environmental potential. Some others have a characterization approach, highlighting the analysis of relevant parameters, such as calorific value and elemental analysis. Very few are totally experimental, highlighting the analysis for the production of charcoal, briquettes, pyrolysis treatment, and catalytic pyrolysis. Among the species under study are Nectandra megapotamica, Ligustrum lucidum, Terminalia catappa, Schinusmolle, Schinus terebinthifolius, Ficus benjamina, Tipuana tipu, Lagerstroemia indica, Cassia fistula, Cenostigma pluviosum, Handroanthus sp., Licania, tomentosa, Morus alba L., Pinus pinea, Pinus nigra J.F. Arnold.
In neotropical countries such as Colombia, Venezuela, Brazil, Ecuador, Peru, and Mexico, urban centers are characterized by the high density of trees on sidewalks, street dividers, and inside houses. This brings with it the need for pruning, whether ornamental in nature or for the maintenance of electrical, telephone, and traffic light networks, among other causes [17,26]. In Colombia, for example, intermediate cities such as Cúcuta stand out for this condition, where there is an inventory of close to 420,000 specimens, where a potential for generating 2100 tons of waste per month has been estimated, an amount that is not negligible for use as a power source. Something similar happens in Cuenca (Ecuador), with an estimate of 51 tons/month. Other larger cities could contribute a greater amount of biomass, as in the case of Bogotá, where an inventory of 1,200,000 specimens has been estimated in its urban area [26–29].
The poor characterization of these residues, many of them are typical of endemic species of the Neotropics [30]. The lack of knowledge of their energy potential has meant that these materials are currently wasted, a very different situation from what occurs in other latitudes of the world, where the high use and research that occurs in territories such as the United Kingdom, Finland, Poland, the United States, and India, among others [31,32] is worth highlighting. With the development of this project, we wanted to contribute by providing information on the energy characterization of the residues of species that are typical of the area, as well as some other species that, due to the effects of globalization, have gained space in the Latin American environment.
For the present work, the City of Cúcuta, Colombia was taken as a reference. There, material from urban pruning was collected directly from the process carried out by one of the companies that performs maintenance activities for electrical networks and ornamentation. In order to guarantee better representativeness, samples were collected from different areas of the city in order to reduce the effects of variables such as the age of the tree and the influence of the water table. The pruning residues of seven of the most representative species were studied according to previous research [26–27]. The residues studied are from the species Licania tomentosa (A), Azadirachta indica (B), Ficus benjamina (C), Terminalia catappa (D), Leucaena leucocephala (E), Prosopis juliflora (F) and Pithecellobium dulce (G), in addition to a mineral coal from the region as a comparative element in some of the results (H). The material that is recently cut from the tree is subjected to a mechanical grinding process, which reduces the material to a size of less than 30 millimeters. Once said granulometry was obtained, it was collected and taken to the laboratory in order to determine its initial moisture content.
The determination of the initial moisture content of each residue was carried out with the help of a Construmetálicas brand drying oven, with heating through electrical resistance. 1000 g of residue were placed in the oven at a temperature of 115°C, monitoring the mass until a constant weight was obtained. An Ohaus brand scale with a resolution of 0.1 g was used in the process. A fraction of the dry material (10 g) was subjected to manual grinding in an agate mortar until all the material passed through the 40 Tyler mesh. It was returned to the drying oven until a constant weight was obtained. 5.0 g of dry material of each species was placed in a crucible and exposed to the laboratory environment (28°C) for 48 h to determine each residue’s hygroscopic response. The apparent density was determined with the help of a 10 ml glass cylinder and a 0.1 g resolution scale.
The elemental chemical analysis (C-H-N-S-O) was determined in Leco Truspec micro model equipment, based on the guidelines of the ASTM D-5373-08 standard, with a grain size of 40 Tyler mesh of the passing material. The sample goes through a combustion oven at 1100°C for the analysis of CHN. The gases obtained are analyzed by infrared cells for the detection of CO2 and H2O. The N2 is analyzed by a TCD detector. For sulfur analysis, the furnace is brought to 1350°C, and SO2 is detected by an infrared sensor. The oxygen determination was made at 1250°C in a helium environment using the analysis module that is included in the equipment. Calorific power was determined using a Parr model 1341EB bomb calorimeter based on the manufacturer’s guidelines and the ASTM E-711-87 and DIN 51900 standards. The room temperature at the time of the test was around 17.5°C. A fuel mass of 1.0 and 2000.0 g of water were used.
In the same way, proximate analysis of the biomass was carried out, following the parameters of the technical standard ASTM E871, ASTM E872, and ASTM D 1102-84. For this, a Thermolyne model 1300 electric heating oven and a Dynamica model air performance model drying oven with electric heating were used. In order to improve the understanding of some results of this research, a methodology was established in order to know the “ease of ignition and combustion” of the fuels under study. These concepts are related to the variable time.
The procedure to measure the ease (time) of achieving ignition consisted of placing a biomass briquette on a laboratory asbestos mesh supported on a tripod. A Bunsen burner connected to a source of liquefied petroleum gas was placed under the mesh. The ignition was started, and the gas outlet knob was opened to its maximum capacity, thus beginning the measurement of the time until the ignition was reached, which was totaled when obtaining visualization of combustion smoke in a constant manner in the test tube. The 50 mm diameter specimen that was used was formed with 10.0 grams of dry material through 40 mesh Tyler, using a pressing pressure of 30 bars, in a Gabrielli brands hydraulic laboratory press.
The thermal gravimetric and heat flow analysis was carried out in TA Instrument brand model STD-Q600 equipment. A dry air atmosphere was used at 40 ml/min, heating at 5°C/min, and 5.0 mg of mass (40 Tyler mesh through) was loaded into an alumina crucible. The estimation of the structural phases of the biomass (hemicellulose, cellulose, and lignin) was carried out according to the first derivative profile of the mass loss thermogram, using the curve deconvolution process through the Origin software. In the same way, TA Universal Analysis software was used in the treatment of thermogravimetric and heat flow data.
The analysis of combustion gases was carried out using the Pfeiffer Vacuum mass model Omnistar GSD320 spectrometer, coupled with the previously described thermal analysis equipment, operating in the MID work mode, which allows monitoring of masses over time, but in qualitative mode, which monitored the following: mass 44, associated with the presence of carbon dioxide, mass 28, associated with the presence of carbon dioxide and carbon monoxide, and, finally, mass 31, associated with nitric oxide and other phases of the NOx group. It was not possible to make a clear identification of sulfur gas phases with the technique (mass 64). Therefore, no results are presented in this regard.
The steam generation test was carried out in equipment built for this purpose, where the container (capacity of 5 liters) with the loaded liquid water (1375 g) was supported on a metal plate, and below it, the combustion furnace was placed. The container had an orifice through which the generated steam could flow, which was connected to a container with an external cooling system under a coupling, in order to condense and recover the steam for later weighing. The combustion test was carried out for a period of 120 min with a maximum provision of 5000 g of fuel for the development of the test. The efficiency of the process was established by knowing the amount of vapor collected and the amount of fuel spent during the duration of the test. This same system was used to semi-quantitatively monitor the formation of NO and SO2 (in ppm) in some of the study residues (A, B, C and D) and in the mineral carbon used as reference. For this, a Madur brand model GA-21 Plus analyzer was used, making measurements every 10 s, during 55 min of testing.
3.1 Properties of Waste as Fuel
In order to make a preliminary recognition of the pruning residues, aspects such as the initial humidity of the residue, density of the material once it has dried, and the hygroscopic response of these residues once subjected to environmental humidity were analyzed. The results are presented in Table 1.
From the information presented in Table 1, the high moisture contents of these freshly-cut residues from the tree stand out. However, it is noteworthy that there is variability between species, highlighting the high concentration of water in the species Pithecellobium dulce, while on the opposite end, Ficus benjamina stands out. This information is relevant when carrying out drying processes based on the fact that the higher the humidity, the more energy is required for its elimination. Even so, it is evident that once the moisture is extracted, the residual or useful mass is significantly reduced. These values reported here can be considered good, since, for example, in a study carried out in Brazil, this initial humidity value reached 71% [20].
The apparent density of the residues was determined in the dry and ground material to reduce the amount of air that was present. The results of Table 1 show variability between species, revealing that the densest material is from Licania tomentosa, followed by Azadirachta indica. On the opposite end of the spectrum, the lightest residues are those of Leucaena leucocephala and Prosopis juliflora. This information is relevant during the transportation of these biomasses to the processing site, where it is less favorable when the material that has to be transported has a lower density. All these density results can be considered in the very light category (<0.5) according to the classification used in Reference number [33], which was a study carried out in Brazil. The fundamental reason for this behavior has to do with the high content of leaves present in pruning residues.
In relation to the hygroscopic response, the test was carried out in order to establish the effects of rehydration and its effects on the drying operation. As previously mentioned in the methodological section, the residues were dried until constant weight was obtained and then exposed to environmental laboratory conditions. Table 1 shows that all species showed a response to rehydration. The highest hygroscopic response was obtained in the species Terminalia catappa and Pithecellobium dulce. These results show that there is no need to dry the residues until all the moisture is eliminated. Due to this behavior, most species’ equilibrium is reached when the humidity is around 11%; therefore, there is no need to reduce humidity further during the drying stage, unless immediate use is made of the biomass without exposing it to its environmental conditions. This fact impacts the calorific value since part of the energy released by the biomass is lost in the heating and evaporation of said humidity during the combustion process. The hygroscopic response seems to have a certain relationship with the presence of cellulose in biomass residues, as has been described in the scientific literature [34].
The elemental chemical composition is another one of the relevant characterization parameters in solid fuels, as in the case of vegetable biomass. The results that were obtained are presented in Table 2.
From the information in Table 2, it is evident that sulfur and nitrogen are present in all the residues, with the concentration of the latter being higher. The identification of the concentration of these elements is very relevant in solid fuels since their release in the gas phase is very likely during combustion, NOx and SOx being the main compounds released from stoichiometry, which have a negative impact on the biosphere. From the scientific literature, it is known that nitric oxide (NO) is the most common phase during combustion. Even so, the formation of NO2 and N2O is also possible, the latter of these gases having the greatest impact on global warming of the planet. In the case of NO and NO2, their release into the atmosphere leads to the formation of acid rain rich in nitric acid. In the case of SOx, its best-known compounds are SO2 and SO3, and their release into the atmosphere is responsible for the formation of acid rain rich in sulfuric acid. Due to its corrosive nature, acid rain is very detrimental to vegetation cover, soil acidification, and even the degradation of certain construction materials associated with cement [36,37].
In the information in Table 2, data from a mineral coal from the same area where the biomass was collected has been included as a comparative element, considering the possible replacement of this fuel by the biomass under study. It may be observed that, in terms of sulfur, all the residues have a lower content than coal, a fact that can be considered positive since it would give biomass an advantage as a substitute fuel. In the case of nitrogen, the situation is more complex since most of the species exceed the concentration found in the mineral coal. Notably, Licania tomentosa is the only species with a lower content of this element. In Section 3.2, there is a further discussion on the formation of these nitrogen compounds on a laboratory scale. Finally, it is important to highlight the results obtained compared with other biomasses. Vassilev and co-workers [38] have compiled information on various biomass species from which one can establish that the values obtained are within the biomass mean, noting that the biomasses with the lowest nitrogen content are associated with material from the stem area (<0.7%) and those with the highest content related to the areas of branches and leaves (up to 3.4%).
Regarding the carbon content of the residues (see Table 2), when comparing the results with the reference coal, it is evident that these have a lower content of this element and a higher content of oxygen. This fact allows us to infer that there is a lower amount of oxidizable elements that provide energy during combustion, which will be reflected in the calorific value of the residue. A positive aspect of this situation is the lower generation of carbon dioxide per kilogram of biomass in relation to the reference fossil fuel. As for hydrogen, there is no evidence of a significant difference with respect to coal. Even so, it is important to highlight the higher hydrogen contents in the species Prosopis juliflora and Azadirachta indica, considering a possible gasification process. The high oxygen content in biomass could become very interesting in gasification processes, considering the formation of carbon monoxide, and studies should continue in this regard, in order to demonstrate its technical and economic feasibility.
Elemental analysis becomes a very valuable input to characterize solid fuels using the van Krevelen diagram [39]. The technique is based on atomically relating the content of hydrogen and carbon and that of oxygen and carbon. The results for the materials studied are presented below in Fig. 1.
Figure 1: Van Krevelen diagram of the materials under study. A: Licania tomentosa; B: Azadirachta indica; C: Ficus benjamina; D: Terminalia catappa; E: Leucaena leucocephala; F: Prosopis juliflora; G: Pithecellobium dulce; H: Mineral coal from the area
From the information shown in Fig. 1, the classification of the study residues within the category of biomass is corroborated. Even so, the residues of Licania tomentosa and Azadirachta indica, with their higher carbon contents, manage to enter the area of influence of the materials known as Peats. The classification for the mineral coal that was studied fits quite well in the coal area of the Van Krevelen diagram. This tool also makes it possible to recognize some structural parameters within the area established for biomass. Values on the far right are related to high cellulose contents, while those on the left are richer in lignin. The central part of the area corresponds to wood-type materials. Based on the aforementioned, it can be inferred that Prosopis juliflora is the residue with the highest cellulose content, while Licania tomentosa and Azadirachta indica are the materials with the highest lignin content. In the same way, the tool offers other support elements (direction of the arrows), such as determining the hydrophilic or hydrophobic character of the material, which, in the case of the materials under study, shows a good correlation with the initial humidity data shown in Table 1, thus explaining the higher humidity values found in Pithecellobium dulce, Prosopis juliflora, and Leucaena leucocephala.
Another very relevant parameter in the characterization of solid fuels is proximate analysis, the resulting information which allows a greater explanation of the behavior of calorific power and reactivity during combustion. The results that were obtained are presented in Table 3.
From the information in Table 3, it is noticeable that pruning residues, despite being subjected to a drying process, still maintain greater moisture than mineral coal. These humidity values further increase due to the hygroscopic response already presented in Table 1, a fact that brings with it a reduction in the lower calorific value. In relation to the ash content, a variation in the results is evident, highlighting the high concentration in Ficus benjamina and Terminalia catappa, while Leucaena leucocephala is the residue with the lowest content. This fact also has an impact on the calorific value of the residue, as can be seen in Table 4.
Regardless of the biomass species studied, it could be stated that a lower ash content will be obtained after combustion compared to the reference mineral coal.
As for the volatile fraction and fixed carbon, there are significant differences compared to mineral coal. The higher contents of hydrogen and oxygen presented in Table 2 are related to the high fraction of volatiles present in biomass residues. This last fact is valuable when considering a gasification process. On the other hand, when thinking about a combustion process to generate steam, for example, the high concentration of volatiles could become counterproductive if the boiler is not well designed since the energy contained in the biomass could be released very quickly, and much of it would be wasted through flue gases. In this last situation, Licania tomentosa and Leucaena leucocephala would be more favorable due to their higher fixed carbon content. This last situation is reflected in the greater presence and durability of the embers in the combustion chamber. Compared with other results reported in the scientific literature [10,38,40], similar values of volatile fractions are evident with respect to other types of biomasses, especially those related to agricultural and herbaceous residues.
The data collected from the proximal analysis is very valuable since it allows the authors to establish an indicator of thermostability [39], which is estimated from the values of fixed carbon and volatile content. The results obtained are presented below in Fig. 2.
Figure 2: Thermostability index of the materials under study
From the information in Fig. 2, it can be inferred that the most thermally stable material is mineral coal, and the most unstable is the residue of Prosopis juliflora. Within the group of biomass residues, the most stable is Leucaena leucocephala, followed by Licania tomentosa. This condition is manifested by the highest carbon content and, most likely, by the highest concentration of more stable structural phases, such as cellulose and/or or lignin. This parameter is very valuable regarding the durability of the fuel during its combustion. In order to complement these results, Table 4 shows the characterization results associated with calorific value, ease of ignition, and ease of combustion.
From Table 4, it can be observed that there are differences in the calorific value data of the different residues, with the best result being leucaena leucocephala, and the lowest values presented by ficus benjamina and terminalia catappa. Here, the relationship between the ash content and the calorific value is evident. Regarding the influence of humidity, the best values of lower calorific value are observed in those residues with lower moisture content, such as Pithecellobium dulce and Prosopis juliflora. These values obtained for waste are very low compared to those of mineral coal and do not reach 60% of the energy supplied by the latter. In relation to other biomasses, the calorific value results, although not high, are not far from those reported in scientific literature, which shows values between 14000 kJ/kg and 23000 kJ/kg, where a large part of the values are around 17000 kJ/kg [10,38,40].
In the case of pruning residues, the information available by species is scarce. Even so, in Reference number [17], values of a calorific value lower than 14702 kJ/kg are reported for pruning residues of Lagerstroemia indica, 15556 kJ/kg for those of Cassia fistula, 15413 kJ/kg for Ligustrum lucidum, and 16267 kJ/kg for Schinus molle, among other species, all of them in the same range of the values found for the species under study.
The ease of ignition is a parameter that was analyzed to evaluate the behavior of the fuel during the burning stage, to generate energy, and to be compared with the results of the thermostability index. Table 4 shows that Azadirachta indica and Prosopis juliflora have greater ease of ignition, a result that is in tune with the information presented in Fig. 2. This parameter is valuable in batch production systems where an ignition cycle of the combustion furnace is continuously generated since soot and carbon monoxide formation is avoided, as is the case with mineral coal.
In order to have a better understanding of the phenomenon of combustion of waste, a thermogravimetric and heat flow analysis was carried out, the results obtained are presented in Fig. 3 and Table 5.
Figure 3: Thermal profiles of the studied residues. (a) Weight loss, (b) Derivate weight loss, (c) Heat Flow. A: Licania tom.; B: Azadirachta indica; C: Ficus benj.; D: Terminalia catappa; E: Leucaena leuc.; F: Prosopis juliflora; G: Pithecellobium dulce
From the information in Fig. 3b, three relevant events located between 200°C and 500°C are evident, which are associated with the oxidation of the most relevant structural components of the biomass according to its thermal stability, that is, hemicellulose, cellulose, and lignin [41–44]. These same events can be seen in Fig. 3c according to their concavity (downward), correspond to exothermic phenomena. In the same way, although they are not visible to the naked eye, two small upward concavity events (endothermic) close to 100°C and 600°C were evident. It is also important to highlight the extractives, which are usually in low concentrations (less than 5%, but with particular cases reaching 15% or more) in accordance with scientific literature, and which, in many cases, tend to oxidize in the same breakdown region as hemicellulose [42–45]. It is evident in all cases that the event with the highest intensity (Figs. 3b and 3c) corresponds to hemicellulose (with the possible influence of the signal due to the presence of extractives). This could be related to the high presence of leaves instead of wood fractions, which are richer in cellulose, as has been evidenced in the scientific literature [46–48]. The high intensity of the event associated with lignin in the residues of the species azadirachta indica and terminalia catappa is noteworthy. From the information in Fig. 3, it is also evident that prosopis juliflora is the residue where heat release starts faster, while licania tomentosa is the one that shows greater stability. This result correlates quite well with the volatile fraction and fixed carbon results shown in Table 3. In the same way, scientific literature reports the effect that some extractives tend to have, which promotes or decreases the biomass degradation temperature. However, this paper did not study this effect [42,44,45].
The group of endothermic events is related to the presence of humidity and the decomposition of the inorganic fraction (carbonates) of the residue. The presence of humidity is shown in the results of the proximal analysis, while the confirmation of the presence of carbonates is based on other results carried out on the ash of the residue (such as X-ray diffraction and thermal analysis, not shown here), where the decomposition of the carbonated phase in this range of temperatures was confirmed.
As previously mentioned in the methodological section, the thermogravimetric analysis was performed using the Origin software (including curve deconvolution for natural polymers) to determine the representativeness of each event, as shown in Table 5. This table shows that in all the residues, there is moisture loss, although the values differ from those shown in the next analysis. This can be explained due to the very small quantity of the sample used in the analysis. The ash content must be considered as the sum of the residual ash column and the mass loss column due to decomposed ash. The results obtained also correlate with those in Table 3. The data in the “Decomposed Ash” column can also provide information on the stability/reactivity of the ash at high temperatures.
As for the representativeness of the structural phases (natural polymers), the experimental conditions reported in this work were the ones that allowed for the greatest separation of each decomposition event for analysis. Even though an additional signal was expected for the extractives around 200°C [42,49], only a small shoulder was evident in the first derivative profile of Terminalia cattapa at 227°C. Consequently, this demonstrates the difficulty of recognizing if the first exothermic event shown in Fig. 3b is only hemicellulose or if it contains information associated with the decomposition of extractives. The deconvolution treatment that was used in this effort, which allowed a better fit, was modeling only three events, which is why Table 5 shows only three columns for the exothermic events, where one of them has been called “Hemicellulose + Extractives”. This was carried out as such because, when consulting scientific literature [46–48,50], it was demonstrated that although there are cases where hemicellulose is greater than cellulose, the reported values are not as high as those quantified here for the first exothermic event. Therefore, one may think that the value presented in the aforementioned column actually contains information from more than one phase. If the influence of the extractives was ruled out, the Leucaena leucocephala and Pithecellobium dulce residues would be those with the highest hemicellulose content.
Regarding cellulose, Table 5 shows that the highest contents are in Licania tomentosa, Prosopis juliflora, and Pithecellobium dulce, and its greater representativeness could be associated with its branching characteristics, which, during cutting, lead to the pruning of coarser branches that are richer in this phase [51]. Lignin is the phase with the least presence in structural polymers. However, it is interesting to highlight the high content of this phase in the Azadirachta indica and Terminalia cattapa residues, considering applications other than combustion [52]. These results that were obtained correlate well with the analysis carried out using the Van Krevelen Diagram (Fig. 1), where, on the one hand, the highest cellulose content in Prosopis juliflora is revealed, and on the other hand, the high lignin values can be observed. In this case, the residue de Azadirachta indica is the most correct in the analysis that was carried out.
Finally, and based on the benefits of thermogravimetric analysis, the ignition temperature and the recalcitrance index (R50) of the biomass residues under study were estimated. These two parameters are also very valuable in the characterization of biomass for energy purposes. For the first of them, the values were estimated with the tangent method, using the profiles of mass loss and the first derivative of mass loss [53], and in the case of R50, its estimation was carried out following the guidelines described in Reference number [39]. The results that were obtained are presented below in Table 6 and Fig. 4.
Figure 4: Recalcitrance index of the materials under study
The information in Table 6 shows the differences and similarities that exist within the different residues and the reference coal in relation to their ignition temperature. In the case of mineral coal, the result obtained is not far from that reported in Reference number [54], which was around 450°C. In the case of biomasses, these values are considered to be within the range reported for other biomasses such as Masson Pine (241°C), Chinese Fir (267°C), Slash Pine (256°C), and Poplar (271°C) [53]. These results that were obtained again correlate with those obtained in the previous sections, where it is shown that Prosopis juliflora is the species with the greatest ease of ignition, while those that take the longest to reach this state are Licania tomentosa and Terminalia catappa.
Regarding R50, its estimation is useful in order to know the thermal stability toward oxidation of the fuel, compared to that of graphite. According to the values that were obtained, the R50 presents three classification ranges, Class A (R50 ≥ 0.7), Class B (0.5 ≤ R50 ≤ 0.7), and Class C (R50 < 0.5). A smaller value in this parameter indicates that less energy is required to overcome the binding energy during the thermal oxidation process [39]. From the information in Fig. 4, it can be seen that coal is the material with the highest R50, while Prosopis juliflora is the one with the lowest value. All the biomass residues have values that are located within the type C classification. Licania tomentosa and Terminalia catappa are the residues with the highest recalcitrance index, data that is quite well related to that which was obtained with the ignition temperature, once again highlighting which residues have higher or lower activation energy during the thermal oxidation process.
3.2 Analysis of Combustion Gases that Were Formed
The last laboratory-scale characterization test was carried out on the flue gases using the mass spectrometry technique, highlighting that only carbon dioxide and nitric oxide could be tracked with certainty, while the carbon monoxide signal results from the combination with CO2. The results obtained are presented in Fig. 5.
Figure 5: Mass spectrometry profiles. (a) Carbon dioxide, (b) Carbon monoxide/Carbon dioxide, (c) Nitric oxide. A: Licania tom.; B: Azadirachta indica; C: Ficus benj.; D: Terminalia catappa; E: Leucaena leuc.; F: Prosopis juliflora; G: Pithecellobium dulce; H: Mineral coal
Fig. 5a shows the presence of events associated with the formation of CO2. According to the thermal analysis (Fig. 3b), the formation of carbon dioxide events should be noticeable around 280°C, 390°C, and 435°C, associated with the oxidation of hemicellulose, cellulose, and lignin that was previously evidenced. However, this behavior is unclear in all the profiles shown in the figure. For example, the low signal for the residues of Prosopis juliflora (F) and Pithecellobium dulce (G) could indicate incomplete combustion under the air conditions used in the experiment. In relation to the signal that was formed, Fig. 5a also shows the absence of the CO2 formation event below 400°C in some residues, knowing in advance (Fig. 3b) that hemicellulose and cellulose oxidation occurs in that temperature range. Instead, in most cases, a formation event above 400°C is observed, which would be associated with the oxidation of lignin [40–43]. In accordance with the above, one may think that there are residues that are more easily oxidized, such as Licania tomentosa and A. indica, while others are more difficult, requiring a greater amount of oxygen to achieve complete combustion. In the case of the structural phases, the results that were obtained suggest the need to make adjustments in the oxidation gas flow in order to guarantee complete combustion and, incidentally, achieve all the release of energy from the fuel, which, in this case, is the plant biomass.
Fig. 5b shows the joint signal of the formation of CO2 and CO (mass 28), where the formation of a signal near 250°C is evident, which does not correspond to the formation of carbon dioxide in Fig. 5a. This fact proves the incomplete combustion in some species, ratifying what has already been expressed for CO2. This situation is problematic due to the great loss of energy and the polluting role of carbon monoxide.
Regarding the formation of nitrogen oxides, Fig. 5c shows changes in the analyzed signal (slope) after 200°C. It is notorious that the formation appears at different temperatures depending on the type of residue. There are even materials in which the change is not noticeable, as in the case of Leucaena leucocephala (E) and Pithecellobium dulce (G), which could suggest that the formation of this gas phase is not so significant. On the other hand, the most intense signal occurs in Licania tomentosa and Ficus benjamina. These results are quite contradictory if one looks at the amount of nitrogen that each residue has according to the information in Table 2, where precisely Leucaena leucocephala and Pithecellobium dulce are the species with the highest content of this element. An explanation for this behavior would be related to the greater or lesser formation of carbon monoxide. The formation of carbon monoxide limits or inhibits the formation of nitric oxide, as seen in the Pithecellobium dulce signals. The opposite effect can be seen in Licania tomentosa, where greater combustion occurs, as evidenced in Fig. 5a, due to the high CO2 signal. In this case, since there is less formation of carbon monoxide, the formation of nitric oxide increases. However, additional studies are required to prove this phenomenon. In order to evaluate the effectiveness of waste as fuel, a steam generation test was carried out, the results of which are presented in Table 7.
3.3 Combustion Test for Steam Generation
In order to evaluate the effectiveness of waste as fuel, a steam generation test was carried out, the results of which are presented in Table 7.
Table 7 shows the lowest amount of steam obtained with Leucaena leucocephala, despite the fact that this residue was the one that produced the highest calorific value. This behavior would be related to what was described in the previous section, where the greater difficulty of this residue to achieve complete combustion is mentioned, which means that part of the energy of the fuel is lost in the combustion gases due to the formation of carbon monoxide, since a good flame was not observed during burning. On the other hand, the high steam generation efficiency of Prosopis juliflora is interesting. In this case, a very good flame was observed during burning, which could be related to the high volatile fraction established in the proximate analysis (Table 3) and the ease of ignition per the information presented in Table 4. These results are also related to the amount of ash and unburnt material shown in Table 7, where it is evident that leucaena leucocephala shows the highest residue values, wherein a large part of it must be a residual organic material, product of its difficulty to burn. In short, the behavior in the steam generation is favored following the following order: Prosopis juliflora > Pithecellobium dulce > Azadirachta indica > Ficus benjamina > Licania tomentosa > Terminalia catappa > Leucaena leucocephala.
Finally, the NO and SO2 formation follow-up test was carried out during the steam generation tests for four of the most representative species in terms of waste produced in the area of influence of this study, Licania tomentosa, Azadirachta indica, Ficus benjamina, and Terminalia catappa being the selected species. As was expressed in the methodological section, 340 measurements were made during 55 min of burning the fuel, in order to see the evolution of the concentration of these gases over time. The results that were obtained are shown in Figs. 6 and 7.
Figure 6: Evolution of SO2 formation during the combustion test
Figure 7: Evolution of NO formation during the combustion test
Regarding the formation of SO2, the information in Fig. 6 shows that the presence of this phase in the combustion gases is quite low, which is why its detection has been difficult in the mass spectrometry tests coupled with the thermal analyzer, where only 5 mg was used. It is evident that the formation is not continuous. Even in residues such as A. Indica, the presence of this gas phase was not detected. The formation events that are evidenced in Fig. 6 are related to the moments in which biomass is fed to the combustion furnace. It is notorious that mineral coal and Terminalia catappa residues are the ones with the highest SO2 formation. When making an average of the 340 measurements carried out, it was established that the concentration in ppm for Licania tomentosa was 2.06, Azadirachta indica 0.00, Ficus benjamina 0.39, Terminalia catappa 3.79 and for mineral coal 6.67. This result shows that, indeed, these biomasses contribute to reducing the amount of SOx released into the atmosphere in the event that this kind of waste is used as a substitute fuel for mineral coal in steam generation boilers.
Regarding the formation of NO, which has been one of the most worrisome aspects that were analyzed during the presentation of the results of the elemental analysis in Table 2 and the identification of its presence by means of mass spectrometry (Fig. 5c), it has been corroborated in this last test (Fig. 7) that, indeed, nitric oxide is formed. The formation keeps the same dynamics as with SOx, evidencing increases during fuel feeding, due to the start of oxidation of the organic phase. In Fig. 7, one can see that all fuels, including coal, form this nitrogen oxide. By doing the same exercise as with the SOx, of averaging the data collected from the 340 measurements, a concentration value in ppm of 39.5 was obtained for Licania tomentosa, 58.9 for Azadirachta indica, of 57.5 for Ficus benjamina, of 76.6 for Terminalia catappa and of 72.4 for mineral coal, which shows that, despite the fact that there is a higher nitrogen content in these biomasses, this element does not necessarily form NOx. It is possible that it is transformed into N2, or that it remains in the solid phase together with the ashes.
The results that were obtained suggest that the pruning residues studied here have the potential for use in the generation of thermal energy through the combustion process. Despite having a large number of leaves, these materials manage to have calorific values between 14170 and 16928 kJ/kg (52% compared to the reference coal), values similar to or better than other currently available biomasses. As important data, the high moisture content of recently cut residues stands out, with values ranging from 37% to 66%. This leads to a significant reduction in the useful mass for combustion. From the chemical composition, it was evidenced that these residues contain a lower sulfur content and, in some cases, also a lower nitrogen content, which, when compared with the mineral coal of the area, offers an important advantage in environmental matters since it was evidenced that, during combustion, there is less formation of polluting gaseous phases such as NO (between 18% and 45% reduction) and SO2 (between 43% and 100% reduction). Similarly, lower carbon contents were observed (between 30.6% and 38.7%) compared to the reference coal, which translates into a lower release of carbon dioxide per kilogram of fuel. The ash contents of these waste materials were lower than those generated for mineral coal in all cases, which is beneficial in terms of solid waste generation (a reduction between 3% and 70%). The proximal analysis showed that there is a weakness in the high representativeness of the volatile component, due to the higher rate of decomposition and heat delivery in a combustion process, which is reflected in the shorter ignition and combustion times that were obtained, as well as in the higher “hemicellulose + extractives” contents according to the thermogravimetric analysis. It was also demonstrated that there are species that are more or less easily oxidized and form carbon dioxide, thus affecting the amount of energy released. The combustion test carried out to generate steam showed that the efficiency of the process depends on several variables, where calorific value is not necessarily the most relevant parameter, as shown by the results obtained with Leucaena leucocephala. Prosopis juliflora proved to be the residue with the highest potential to be used as a substitute for mineral coal. However, more appropriate combustion treatments (for example, briquettes) are needed to increase the performance obtained in this study, which was only 28% compared to that of the reference fuel.
Acknowledgement: The authors express their gratitude to the Universidad Libre and the Universidad Nacional de Colombia for the support provided for the development of this research.
Funding Statement: The authors thank the Ministry of Science, Technology and Ipnnovation of Colombia through the “Fondo Francisco José de Caldas” National Financing Fund for Science, Technology and Innovation for the financing provided for the development of the project (Project 120885272102, Call 852 of 2019).
Author Contributions: The authors declare that all the efforts made for the construction of this work were carried out jointly or in groups, it is not possible to discriminate the role performed by each of the researchers.
Conflicts of Interest: The authors declare that they have no conflicts of interest to report regarding the present study.
References
1. Bakare, M. S., Abdulkarim, A., Zeeshan, M., Shuaibu, A. N. (2023). A comprehensive overview on demand side energy management towards smart grids: Challenges, solutions, and future direction. Energy Informatics, 6(1), 1–59. [Google Scholar]
2. Sharvini, S. R., Noor, Z. Z., Chong, C. S., Stringer, L. C., Yusuf, R. O. (2018). Energy consumption trends and their linkages with renewable energy policies in East and Southeast Asian Countries: Challenges and opportunities. Sustainable Environment Research, 28(6), 257–266. [Google Scholar]
3. Oliveira, J., Trindade, T. C. G. (2018). Renewable energy sources. In: Sustainability performance evaluation of renewable energy sources: The case of Brazil. Switzerland, Cham: Springer. [Google Scholar]
4. Henry, A., Prasher, R., Majumdar, A. (2020). Five thermal energy grand challenges for decarbonization. Nature Energy, 5(9), 635–637. [Google Scholar]
5. Stančin, H., Mikulčić, H., Wang, X., Duić, N. (2020). A review on alternative fuels in future energy system. Renewable and Sustainable Energy Reviews, 128, 109927. [Google Scholar]
6. Ludin, N. A., Mustafa, N. I., Hanafiah, M. M., Ibrahim, M. A., Teridi, M. A. M. et al. (2018). Prospects of life cycle assessment of renewable energy from solar photovoltaic technologies: A review. Renewable and Sustainable Energy Reviews, 96, 11–28. [Google Scholar]
7. Olabi, A. G., Abdelkareem, M. A. (2022). Renewable energy and climate change. Renewable and Sustainable Energy Reviews, 158, 112111. [Google Scholar]
8. Shortall, R., Davidsdottir, B., Axelsson, G. (2015). Geothermal energy for sustainable development: A review of sustainability impacts and assessment frameworks. Renewable and Sustainable Energy Reviews, 44, 391–406. [Google Scholar]
9. Falnes, J. (2007). A review of wave-energy extraction. Marine Structures, 20(4), 185–201. [Google Scholar]
10. Vassilev, S. V., Vassileva, C. G., Vassilev, V. S. (2015). Advantages and disadvantages of composition and properties of biomass in comparison with coal: An overview. Fuel, 158, 330–350. [Google Scholar]
11. Tanger, P., Field, J. L., Jahn, C. E., DeFoort, M. W., Leach, J. E. (2013). Biomass for thermochemical conversion: Targets and challenges. Frontiers in Plant Science, 4, 218. [Google Scholar] [PubMed]
12. Perea-Moreno, M. A., Samerón-Manzano, E., Perea-Moreno, A. J. (2019). Biomass as renewable energy: Worldwide research trends. Sustainability, 11(3), 863. [Google Scholar]
13. Danner, H., Braun, R. (1999). Biotechnology for the production of commodity chemicals from biomass. Chemical Society Reviews, 28(6), 395–405. [Google Scholar]
14. Mussatto, S. I., Yamakawa, C. K., van der Maas, L., Dragone, G. (2021). New trends in bioprocesses for lignocellulosic biomass and CO2 utilization. Renewable and Sustainable Energy Reviews, 152, 111620. [Google Scholar]
15. Parikka, M. (2004). Global biomass fuel resources. Biomass and Bioenergy, 27(6), 613–620. [Google Scholar]
16. Nunes, L. J. R., Causer, T. P., Ciolkosz, D. (2020). Biomass for energy: A review on supply chain management models. Renewable and Sustainable Energy Reviews, 120, 109658. [Google Scholar]
17. Maccarini, A. C., Bessa, M. R., Errera, M. R. (2020). Energy valuation of urban pruning residues feasibility assessment. Biomass and Bioenergy, 142, 105763. [Google Scholar]
18. Correia, G. R., Machado, J. C., Almeida, S. S., Santos, L. A. C. (2022). Produção científica sobre resíduos de poda urbana: Uma análise cienciométrica. Revista Brasileira de Geografia Física, 15(4), 1701–1714. [Google Scholar]
19. de Meira, A. M., Nolasco, A. M., Klingenberg, D., de Souza, E. C., Dias Júnior, A. F. (2021). Insights into the reuse of urban forestry wood waste for charcoal production. Clean Technologies and Environmental Policy, 23, 2777–2787. [Google Scholar]
20. Smith, A., Alesi, L. S., Varanda, L. D., Silva, D., Santos, L. R. et al. (2019). Production and evaluation of briquettes from urban pruning residue and sugarcane bagasse. Revista Brasileira de Engenharia Agrícola e Ambiental, 23, 138–143. [Google Scholar]
21. Cruz-Estrada, R. H., Guillén-Mallette, J., Cupul-Manzano, C. V., Balam-Hernández, J. I. (2020). Potential use of waste from tree pruning and recovered plastic to obtain a building material: Case study of Merida, Mexico. Waste Management & Research, 38(11), 1222–1230. [Google Scholar]
22. Pérez-Arévalo, J. J., Velázquez-Martí, B. (2018). Evaluation of pruning residues of Ficus benjamina as a primary biofuel material. Biomass and Bioenergy, 108, 217–223. [Google Scholar]
23. Biocca, M., Gallo, P., Sperandio, G. (2022). Potential availability of wood biomass from urban trees: Implications for the sustainable management of maintenance yards. Sustainability, 14(18), 11226. [Google Scholar]
24. Lago, A., Sanz, M., Gordón, J. M., Fermoso, J., Pizarro, P. et al. (2022). Enhanced production of aromatic hydrocarbons and phenols by catalytic co-pyrolysis of fruit and garden pruning wastes. Journal of Environmental Chemical Engineering, 10(3), 107738. [Google Scholar]
25. Springer, T. L. (2012). Biomass yield from an urban landscape. Biomass and Bioenergy, 37, 82–87. [Google Scholar]
26. Gelves, J., Rozo, S., Monroy, R., Romero, Y. (2022). Análisis de la generación de residuos de podas en el área metropolitana de Cúcuta (Colombia). Mundo Fesc, 11, 14–22. [Google Scholar]
27. Gelves, J., Rozo, S., Monroy, R., Romero, Y., Dorkis et al. (2022). Residuos de poda en el Área Metropolitana de Cúcuta: Potencial energético de algunas especies presentes en el territorio. Investigación, desarrollo tecnológico e innovación en ingeniería y administración. Colombia: Editorial Servicio Nacional de Aprendizaje. [Google Scholar]
28. Yánez-Iñiguez, L., Urgilés-Urgilés, E., Zalamea-León, E., Barragán-Escandón, A. (2020). Potencial de los residuos forestales para la contribución a la matriz energética urbana. La granja. Revista de Ciencias de la Vida, 32(2), 42–53. [Google Scholar]
29. Corzo, G. T. (2006). Manejo del arbolado urbano en Bogotá. Colombia Forestal, 9(19), 187–205. https://www.redalyc.org/pdf/4239/423941362011.pdf [Google Scholar]
30. Janssen, R., Rutz, D. D. (2011). Sustainability of biofuels in Latin America: Risks and opportunities. Energy Policy, 39(10), 5717–5725. [Google Scholar]
31. Bauen, A. (2005). Biomass in Europe. Bioenergy-realizing the potential. Netherlands: Elsevier. [Google Scholar]
32. Bhattacharya, S. C. (2002). Biomass energy in Asia: A review of status, technologies, and policies in Asia. Energy for Sustainable Development, 6(3), 5–10. [Google Scholar]
33. Riesco Muñoz, G., Imaña Encinas, J., Elías de Paula, J., (2019). Densidad de la madera de 59 especies del orden Sapindales procedentes de bosques naturales brasileños. Madera y Bosques, 25(2), e2521817. [Google Scholar]
34. Lovikka, V. A., Rautkari, L., Maloney, T. C. (2018). Changes in the hygroscopic behavior of cellulose due to variations in relative humidity. Cellulose, 25(1), 87–104. [Google Scholar]
35. Valderrama, G., Pérez, F. (1987). Evaluación de las propiedades de los carbones de Norte de Santander. Colombia: Ingeominas-Carbocol. [Google Scholar]
36. Williams, A., Jones, J. M., Ma, L., Pourkashanian, M. (2012). Pollutants from the combustion of solid biomass fuels. Progress in Energy and Combustion Science, 38(2), 113–137. [Google Scholar]
37. Asghar, U., Rafiq, S., Anwar, A., Iqbal, T., Ahmed, A. et al. (2021). Review on the progress in emission control technologies for the abatement of CO2, SOx and NOx from fuel combustion. Journal of Environmental Chemical Engineering, 9(5), 106064. [Google Scholar]
38. Vassilev, S. V., Baxter, D., Andersen, L. K., Vassileva, C. G. (2010). An overview of the chemical composition of biomass. Fuel, 89(5), 913–933. [Google Scholar]
39. Zhu, X., He, M., Xu, Z., Luo, Z., Gao, B. et al. (2022). Combined acid pretreatment and co-hydrothermal carbonization to enhance energy recovery from food waste digestate. Energy Conversion and Management, 266, 115855. [Google Scholar]
40. Ebeling, J. M., Jenkins, B. M. (1985). Physical and chemical properties of biomass fuels. Transactions of the ASAE, 28(3), 898–902. [Google Scholar]
41. Saldarriaga, J. F., Aguado, R., Pablos, A., Amutio, M., Olazar, M. et al. (2015). Fast characterization of biomass fuels by thermogravimetric analysis (TGA). Fuel, 140, 744–751. [Google Scholar]
42. Poletto, M., Zattera, A. J., Santana, R. M. (2012). Structural differences between wood species: Evidence from chemical composition, FTIR spectroscopy, and thermogravimetric analysis. Journal of Applied Polymer Science, 126(S1), 337–344. [Google Scholar]
43. Tenorio, C., Moya, R. (2013). Thermogravimetric characteristics, its relation with extractives and chemical properties and combustion characteristics of ten fast-growth species in Costa Rica. Thermochimica Acta, 563, 12–21. [Google Scholar]
44. Moya, R., Rodríguez-Zúñiga, A., Puente-Urbina, A. (2017). Thermogravimetric and devolatilisation analysis for five plantation species: Effect of extractives, ash compositions, chemical compositions and energy parameters. Thermochimica Acta, 647, 36–46. [Google Scholar]
45. Sebio-Puñal, T., Naya, S., López-Beceiro, J., Tarrío-Saavedra, J., Artiaga, R. (2012). Thermogravimetric analysis of wood, holocellulose, and lignin from five wood species. Journal of Thermal Analysis and Calorimetry, 109(3), 1163–1167. [Google Scholar]
46. Rosales-Castro, M., Honorato-Salazar, J. A., Santos-García, A. B., Pérez-López, M., Colotl-Hernández, G. et al. (2016). Composición química de las hojas y ramas de Cedrela odorata L. de dos plantaciones forestales como fuente de materia prima lignocelulósica. Madera y Bosques, 22(2), 131–146. [Google Scholar]
47. Kulić, G., Radojičić, V. (2011). Analysis of cellulose content in stalks and leaves of large leaf tobacco. Journal of Agricultural Sciences, 56(3), 207–215. [Google Scholar]
48. Petisco, C., Vázquez de Aldana, B. R., Zabalgogeazcoa, I., Mediavilla, S., García Criado, B. (2005). Determinación de lignina y celulosa en hojas de plantas leñosas mediante NIRS: Comparación de métodos estadísticos. https://digital.csic.es/bitstream/10261/10138/1/6.pdf?iframe=true&width=80%25&height=80%25 [Google Scholar]
49. Shebani, A. N., Van Reenen, A. J., Meincken, M. (2008). The effect of wood extractives on the thermal stability of different wood species. Thermochimica Acta, 471(1–2), 43–50. [Google Scholar]
50. Díez, D., Urueña, A., Piñero, R., Barrio, A., Tamminen, T. (2020). Determination of hemicellulose, cellulose, and lignin content in different types of biomasses by thermogravimetric analysis and pseudocomponent kinetic model (TGA-PKM method). Processes, 8(9), 1048. [Google Scholar]
51. Kartal, F., Özveren, U. (2021). An improved machine learning approach to estimate hemicellulose, cellulose, and lignin in biomass. Carbohydrate Polymer Technologies and Applications, 2, 100148. [Google Scholar]
52. Cassoni, A. C., Costa, P., Vasconcelos, M. W., Pintado, M. (2022). Systematic review on lignin valorization in the agro-food system: From sources to applications. Journal of Environmental Management, 317, 115258. [Google Scholar] [PubMed]
53. Jia, G. (2021). Combustion characteristics and kinetic analysis of biomass pellet fuel using thermogravimetric analysis. Processes, 9(5), 868. [Google Scholar]
54. Li, X. G., Ma, B. G., Xu, L., Hu, Z. W., Wang, X. G. (2006). Thermogravimetric analysis of the co-combustion of the blends with high ash coal and waste tyres. Thermochimica Acta, 441(1), 79–83. [Google Scholar]
Cite This Article
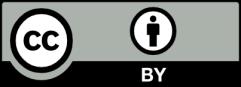