Open Access
REVIEW
In vitro Mutagenesis for the Improvement of Agave Genus
Laboratorio de Biotecnología Vegetal, TecNM-Campus Tuxtla Gutiérrez, Tuxtla Gutiérrez, 29050, Mexico
* Corresponding Authors: Joaquín Adolfo Montes-Molina. Email: ; Carlos Alberto Lecona-Guzmán. Email:
Phyton-International Journal of Experimental Botany 2023, 92(7), 2065-2078. https://doi.org/10.32604/phyton.2023.028784
Received 07 January 2023; Accepted 31 March 2023; Issue published 29 May 2023
Abstract
Biotechnological techniques provide a viable alternative to help improve and increase the production of plant species of agricultural and economic importance, which have been affected over the years by climate change, increasing their susceptibility to pests and/or diseases, generating losses in production as well as a decrease in their regenerative and genetic diversity. The application of biotechnological techniques such as in vitro mutagenesis offers a viable option for the generation of crops that are resistant to the different factors caused by abiotic and biotic stress. In vitro mutagenesis has been used in an efficient way to generate genetic changes in different plant species. However, these methods have not been studied thoroughly in crops of agro-industrial interest, such as agave, which represents an economic resource of national importance and are considered as endemic species of Mexico. Therefore, this literary review aimed to focus on the studies that have been used for the genetic improvement of this species via mutagenesis techniques in plants in the agave genus. Therefore, the objective was to set a precedent for future genetic studies that aim to obtain more productive regenerants for various industries, such as food and pharmaceutical. It is also of great interest to compile information from basic research that helps understand and elucidate a model of possible defense mechanisms that are activated in the Agave genus.Keywords
Nomenclature
BA | 6-benzyl adenine |
DNA | Deoxyribonucleic acid |
EMS | Ethyl methanesulfonate |
IAA | Indoleacetic acid |
PAL | Phenylalanine ammonia lyase |
PEG | Polyethylene glycol |
PRs | Pathogenesis-related proteins |
ROS | Reactive oxygen species |
TIB | Temporary immersion Bioreactor |
Genetic improvement in plants began with the domestication of wild ancestors of maize (Zea mays L.) [1,2]. In agriculture, breeding by mutations plays a fundamental role in the development of new cultivars with an improvement in yield, quality, resistance and/or tolerance to biotic and abiotic stress through novel tools [3], whereby mutation is the main source of genetic variations in plants [4]. Numerous works have been reported in literature where mutagenesis has been implemented in plants. However, regarding the Agave genus, there are few reports using in vitro mutagenesis for genetic improvement, probably because it is a crop that presents problems in its traditional propagation and low genetic variability [5,6], as well as affectations caused by abiotic and biotic stresses. Genetic improvement programs in agave are relevant, as it is an important crop because of applications as a raw material in the traditional production of alcoholic beverages, food, and fibers [7,8]. Thus, the culture of plant tissues facilitates the use of in vitro mutagenesis [9] when carrying out genetic improvement in the study of plants of agricultural and economic interest.
2 Genetic Improvement in Plants
Genetic improvement is a tool that allows to increase genetic diversity in different plant species [10], which can cause new biological functions [11,12]. Biotechnological techniques, such as the implementation of Plant Tissue Culture (PTC), have facilitated the use of in vitro mutagenesis, the combination of these two techniques has shown the efficacy to induce variability in a short period of time. Research with cultures has been reported where mutagenesis has been used to induce useful features, such as quality improvement and resistance to diseases, this technique is used at various levels of tissue differentiation (cell cultures, callus, somatic embryos and seeds) [13].
Induced mutagenesis produces macromolecular changes, which lead to physiological and morphological alterations of plant genotypes [14,15]. The most important factors to be considered for in vitro induced mutagenesis are the selection of the mutagen, dose and exposure time, type of plant, as well as the determination of LD50. The latter has great relevance, as high concentrations and long periods of exposure can cause significant effects on plant material mortality [16,17].
Genetic hereditary variations created by mutagens are very efficient as the frequency of spontaneous mutations is minor [18,19]. It is well documented that induction of mutagenesis using in vitro culture techniques favors the development of improved cultivars in less time [19,20].
3 In vitro Cultures as a Tool for Agave Propagation
The culture of plant tissues is a tool of great importance that is fundamental for the propagation and improvement of plants of interest [21]. It is used as a feasible alternative for the in vitro production of disease and pest-free plants [22,23], rapid multiplication, genomic transformation, and production of various specialized metabolites of interest [24,25]. It is regarded as an important technique for large-scale micropropagation of plants [26], which are generally difficult to propagate using traditional methods [27,28]. It is also considered important for the conservation of important, threatened, or endangered species [29], promotes a fast and reliable system for obtaining genetically uniform and disease-free clone plants [30,31], without seasonal variations but rich in bioactive compounds [32].
Regarding the Agave genus, studies have been reported in recent years about the use of in vitro culture techniques, which are shown in Table 1.
On the other hand, it is well known that the use of temporary immersion systems increases the production and improves the quality of micropropagated explants. Some publications with the Agave genus are listed in Table 2.
Genetic transformation is one of the technologies used to reveal or modulate gene function [43]. Transformation by Agrobacterium tumefanciens is the most popular method for transferring genes of desirable characteristics to plants [44,45], this technique has proven to be a strategy with great potential for increasing specialized metabolites in different plants [46,47]. Suárez-González et al. [48] described the genetic manipulation of Agave salmiana via two transformation systems. By using mediation by A. tumefanciens and bioballistics, 31 transgenic plants with small roots using Agrobacterium were obtained, suggesting that the combination of callus of A. salmiana with A. tumefanciens is suitable for the transgenesis, which helps to tolerate diseases in agave plants and facilitate to overexpress endogenous genes, such as those related in the biosynthesis of fructans. The use of molecular markers has been shown to be useful for evaluating the genetic fidelity of plants [49], caused by transformation by Agrobacterium and by genetic variation experiments, such as somaclonal variation [50].
Somaclonal variations were described by Larkin et al. [51]. These variations refer to induced abnormalities that become genetic and heritable in crops [52,53], being a phenomenon observed in cells and tissues in vitro [54]. They are essential and of great importance for genetic improvement and for obtaining new cultivars, generating morphological and physiological changes, and for creating resistance or tolerance to biotic and abiotic stress [55]. Kashtwari et al. [9] described that the efficiency of mutation induction in vitro allows large populations to be managed.
The application of in vitro mutagenesis has been used more frequently for improving the quality and increasing to resistance in plants [56]. This has occurred by inducing heritable changes in the genetic constitution of cells through the alteration of their deoxyribonucleic acid (DNA) [57].
The use of in vitro mutagenesis is a strategy that can accelerate breeding programs, as the resulting genetic variations can lead to the development of new varieties [10]. This method has been used mainly with the aim of obtaining better-adapted varieties through the alteration of traits, such as the period of maturity and flowering, seed size, resistance to diseases, yield components, as well as tolerance to abiotic and biotic stress [58,59].
To improve the frequency of mutations in vivo and in vitro random mutagenesis, as well as for expediting selections of desired traits, traditional mutation and in vitro mutagenesis employ various physical and chemical mutagens, which generate phenotypic and genotypic variations [60]. An adequate dose of mutagens, as well as exposure time, are important factors affecting in vitro mutagenesis, depending on the phase of tissue differentiation, species, and genotype [61].
6.1 Type of Mutagens Applied in vitro
In vitro mutations can be generated by physical means (gamma rays, ultraviolet light) or chemical (ethyl methanesulfonate, sodium azide, 5-azacitidine and salicylic acid) among others [62].
Physical mutagens consist in exposing parts of the plant to radiation, which causes the breakdown of the DNA chain, thus generating the loss of one or more nucleotides [63], causing modifications in DNA, cell membranes, lipids, enzymes, and other cellular constituents [64].
Chemical mutagens can be broadly classified into alkylating agents, intercalating agents, deaminating agents and base analogues. Alkylation causes the link-up of a hydrocarbon group with low molecular weight to the bases, which changes their pairing properties since true DNA replication depends on precise base mating. Base alkylation causes incorrect pairing and will therefore induce mutations [63].
The development of mutant plants has been described from in vitro mutagenesis techniques that have used both chemical and physical agents, whereby plants have been generated with desirable characteristics, some of these features include resistance to diseases, early maturity, and tolerance to stress biotic [65,66]. Among the most used mutagenic agents in monocotyledonous plants are those described in Table 3.
7 Biotic and Abiotic Stresses in Plants
Stress in a biological system induces a response with various negative morphological, physiological, and biochemical changes, which put the vital form of the organism at risk [73]. Plants grow in poor climatic conditions that prevent them from acquiring their full genetic capacity to grow and develop, which imposes a severe impact on their economic performance [74]. Plants are continuously exposed to a large amount of stress [75], which can be classified in abiotic stress and biotic stress. It is called abiotic stress, if it is caused by non-living factors such as drought, exposure to heavy metals, cold, heat and salinity. But if caused by pathogenic or parasitic organisms, such as fungi, viruses, bacteria, or insects it is called biotic stress [76]. These organisms can reduce the photosynthetic activity and crop yield [77] (Fig. 1).
Figure 1: Environmental factors that create stress in plants (biotic and abiotic) modified from Schulze et al. [78]
Abiotic stress, such as high salt content, extreme temperatures, and drought, can be unfavorable to plants because they cause oxidative stress, cause cell damage, and produce reactive oxygen species (ROS) [79,80], causes modifications in different physiological processes (stomata opening, photosynthesis, cell expansion, positive regulation of antioxidants and accumulation of organic solutes such as amino acids, polyamines and carbohydrates) [81]. In turn, these can cause a reduction in the yield of some crops depending on when this stress occurs [82,83].
Abiotic stress is also known to interfere with the transportation of extracellular and intracellular molecules, which are essential for optimal plant maintenance, development, and growth [84]. The plasma membrane is a barrier that separates and protects the plant’s internal cellular content from external environments and is important for receiving signals that are often mediated by ionic charge and solute fluctuations. These ion and solute concentrations received by the plasma membrane help during modulated absorption of inorganic nutrients and cause changes in secondary messenger concentrations that drive osmotic gradients essential for cell expansion [85,86]. Abiotic stress directly affects the components of the photosynthetic apparatus, causing a reduction in the photosynthetic efficiency of the plant, changes in the amount of CO2 and stomatal conductance, and the reduction of CO2 leads to a greater activity of the RUBISCO enzyme in the photorespiration process, as a result, it is seen an accumulation of salt and a decrease in stomatal conductance [81].
Biotic stress is induced by nematodes, fungi, bacteria viruses, invasion of parasitic plants and pest attacks [87], which cause severe yield losses or crop failures during infestation [83,88].
To overcome biotic stress, plants use multiple classes of proteins: catalytic enzymes involved in cell wall modifications, ROS, pathogenesis-related proteins and phytohormones, as well as post-translational factors, receptors, and receptor-like kinases [87,89–91]. The pathogen recognition by the plant generates disturbances in the potential of the cell membrane, which causes an increase in Ca2+ at the intracellular level, which acts as a second messenger to activate calcium-sensitive proteins and signal transduction pathways [92]. It has been described that plants generally produce responses mediated by Salicylic Acid to counteract the damage caused by biotrophic pathogens, while to combat necrotrophic agents presence, responses are mediated by Jasmonic Acid and Ethylene [93].
Biotic agents are classified into biotrophs, necrotrophs and hemibiotrophs, according to their type of association with the host plant. Biotrophic pathogens evade surveillance and/or suppress the defense response, whereas necrotrophic pathogens take advantage of natural wounds and openings to penetrate plant tissues, grow intercellularly and produce enzymes and/or toxins that cause tissue death and nutrient outflow. Finally, another group of pathogens behaves like biotrophs during the early stages of infection by bypassing the plant’s defense response. During the late stages of the disease, they display characteristics of necrotrophs, and the death of plant tissue occurs. These pathogens are called hemibiotrophs and include various fungi and bacteria [94,95].
8 Application of in vitro Mutagenesis in the Agave Genus
Agaves are a source of different products in the food and drug industry, production of biofuel, among other uses [96]. Its greatest application is the production of alcoholic beverages such as mezcal, bacanora, tequila, raicilla, pulque and comiteco [6,7,33,35].
There has been an increase in interest in the antioxidant, antimicrobial, antidiabetic, anti-inflammatory, antitumor properties of agave, among other factors.
However, there are few reports on the genetic improvement of species of the Agave genus, despite being a crop of agronomic and economic importance, and which has little natural regeneration and low genetic diversity. An example is the studies carried out by Trejo et al. [97], report genetic variations in seventeen agave varieties, classified into three clusters, describing that the high number of Agave races without differences in their morphological diversity or genetic reserves, can be explained by the plasticity of morphological traits and the events of gene flow.
On the other hand, regarding the application of in vitro mutagenesis in Agave, Díaz-Martínez et al. [98], described that regeneration process from different explants of Agave tequilana Weber var. Azul, it could reveal details about gene expression as a mechanism to cause the silencing of elements and avoid possible mutational effects.
Tejavathi et al. [99] reported genetic variability in Agave vera-cruz Miller plants, which were obtained through direct and indirect organogenesis and grown with arbuscular mycorrhizal fungi (AMF). Plants were also grown with tissues derived from exposure to gamma radiation at 15 Gy for 20 min in 2-min intervals and from exposure to ethyl methanesulfonate in intervals going from 0.1% to 1% for 1 to 5 h. It was observed that a distinct phenotypic variant deriving from indirect organogenesis presented more polymorphism, followed by samples treated with mutagens. Plants obtained of direct organogenesis and subsequently treated with AMF generated a separate subgroup that indicated an alteration. It was thus proposed that the use of in vitro culture methods, along mutagenesis and AMF symbiosis, are a tool in increasing the genetic variability of propagated plants.
On the other hand, Reyes-Zambrano et al. [69] reported that the use of ethyl methanesulfonate induced variations in the morphometric and morphological parameters of the seedlings obtained from Agave americana L., where 60% of the seedlings presented dwarfism and different foliar forms, with a lack of spines. Also, an increase in the fructan content of 30% with respect to the seedlings in the control treatment could be observed, along with an increase in PAL activity.
Studies carried out by Espino et al. [71], described the obtention of somatic embryos and mutant seedlings from tissue irradiated with gamma rays 60Co. It was mentioned that the radiation generated mutations that altered competition in the cells, thus decreasing embryogenic callus and the development of somatic embryos. For their part, Reyes-Zambrano et al. [100], described that chitinase activity increased significantly in Agave americana L. plants, infected with Fusarium oxysporum at 15 days after inoculation (DAI) regarding uninfected plants, while β-1, 3 glucanase activity showed no statistically significant difference with control plants the results suggest that, in response to F. oxysporum infection, A. americana L. activates chitinase-like PRs proteins.
Recently, Reyes-Zambrano et al. [101] reported obtaining somaclonal variants in Agave americana L., that were resistant to F. oxysporum. These resulted from the induction of mutations with EMS at a concentration of 200 mM during an exposure time of 2 h.
Currently, in vitro mutagenesis represents an effective biotechnological tool to be used in genetic improvement programs, this technique becomes more relevant when it is applied to species of agronomic importance such as those of the Agave genus, which are characterized by having little genetic variation and slow natural regenerative capacity.
In vitro mutagenesis in combination with plant tissue culture and large-scale micropropagation systems such as temporary immersion systems can be used to obtain plants with outstanding characteristics such as resistance to biotic factors and/or tolerance to abiotic factors, fiber production, increase in the content of fructans and other specialized secondary metabolites such as inulin, antioxidant compounds among others.
Funding Statement: The authors received no specific funding for this study.
Author Contributions: The authors confirm contribution to the paper as follows: Study conception and design: V-H S., L-G CA., M-M JA.; Supervision: G-M FA., L-G CA., R-Z SJ.; Translation and editing of the manuscript: V-H S., M-M JA., R-Z SJ. All authors reviewed the results and approved the final version of the manuscript.
Conflicts of Interest: The authors declare that they have no conflicts of interest to report regarding the present study.
References
1. Doebley, J. (2004). The genetics of maize evolution. Annual Review of Genetics, 38, 37–59. https://doi.org/10.1146/annurev.genet.38.072902.092425 [Google Scholar] [PubMed] [CrossRef]
2. Xiao, Y., Jiang, S., Cheng, Q., Wang, X., Yan, J. et al. (2021). The genetic mechanism of heterosis utilization in maize improvement. Genome Biology, 22(1), 1–29. https://doi.org/10.1186/s13059-021-02370-7 [Google Scholar] [CrossRef]
3. Abu-Ellail, F. F., Salem, K. F., Saleh, M. M., Alnaddaf, L. M., Al-Khayri, J. M. (2021). Molecular breeding strategies of beetroot (Beta vulgaris ssp. vulgaris var. conditiva Alefeld). In: Advances in plant breeding strategies: vegetable crops, pp. 157–212. Cham: Springer. https://doi.org/10.1007/978-3-030-66965-2_4 [Google Scholar] [CrossRef]
4. Jayaramachandran, M., Saravanan, S., Motilal, A., Prabhu, P. C., Hepziba, S. J. et al. (2020). Genetic improvement of a neglected and underutilised oilseed crop: Sesame (Sesamum indicum L.) through mutation breeding. The Nucleus, 63(3), 293–302. https://doi.org/10.1007/s13237-020-00329-w [Google Scholar] [CrossRef]
5. Aquino-Bolaños, T., Ruiz-Vega, J., Giron-Pablo, S., Pérez-Pacheco, R., Martínez-Tomas, S. et al. (2011). Interrelationships of the agave weevil Scyphophorus acupunctatus (GyllenhalErwinia carotovora (Dyeentomopathogenic agents and agrochemicals. African Journal of Biotechnology, 10(68), 15402–15406. https://doi.org/10.5897/ajbA11.666 [Google Scholar] [CrossRef]
6. Martinez-Rodriguez, A., Macedo-Raygoza, G., Huerta-Robles, A. X., Reyes-Sepulveda, I., Lozano-Lopez, J. et al. (2019). Agave seed endophytes: Ecology and impacts on root architecture, nutrient acquisition, and cold stress tolerance. In: Verma, S., White, J. J. (Eds.Seed endophytes, pp. 139–170. Cham: Springer. https://doi.org/10.1007/978-3-030-10504-4_8 [Google Scholar] [CrossRef]
7. Nava-Cruz, N. Y., Medina-Morales, M. A., Martinez, J. L., Rodriguez, R., Aguilar, C. N. (2015). Agave biotechnology: An overview. Critical Reviews in Biotechnology, 35(4), 546–559. https://doi.org/10.3109/07388551.2014.923813 [Google Scholar] [PubMed] [CrossRef]
8. Martínez-Herrera, R. E., Rutiaga-Quiñones, O. M., Alemán-Huerta, M. E. (2021). Integration of agave plants into the polyhydroxybutyrate (PHB) production: A gift of the ancient aztecs to the current bioworld. Industrial Crops and Products, 174, 114188. https://doi.org/10.1016/j.indcrop.2021.114188 [Google Scholar] [CrossRef]
9. Kashtwari, M., Wani, A. A., Dhar, M. K., Jan, S., Kamili, A. N. (2018). Development of an efficient in vitro mutagenesis protocol for genetic improvement of saffron (Crocus sativus L.). Physiology and Molecular Biology of Plants, 24(5), 951–962. https://doi.org/10.1007/s12298-018-0576-6 [Google Scholar] [PubMed] [CrossRef]
10. Nasri, F., Zakizadeh, H., Vafaee, Y., Mozafari, A. A. (2021). In vitro mutagenesis of Chrysanthemum morifolium cultivars using ethylmethanesulphonate (EMS) and mutation assessment by ISSR and IRAP markers. Plant Cell, Tissue and Organ Culture, 1–17. https://doi.org/10.1007/s11240-021-02163-7 [Google Scholar] [CrossRef]
11. Mukai, T., Lajoie, M. J., Englert, M., Söll, D. (2017). Rewriting the genetic code. Annual Review of Microbiology, 71, 557–577. https://doi.org/10.1146/annurev-micro-090816-093247 [Google Scholar] [PubMed] [CrossRef]
12. Shelake, R. M., Pramanik, D., Kim, J. Y. (2019). Evolution of plant mutagenesis tools: A shifting paradigm from random to targeted genome editing. Plant Biotechnology Reports, 13(5), 423–445. https://doi.org/10.1007/s11816-019-00562-z [Google Scholar] [CrossRef]
13. Bhat, S., Sharma, S., Sharma, K. V. (2017). Effect of in vitro mutagenesis on in vivo growth characteristics of strawberry cv. camarosa. International Journal of Current Microbiology and Applied Sciences, 6(8), 3406–3417. https://doi.org/10.20546/ijcmas.2017.607.408 [Google Scholar] [CrossRef]
14. Laskar, R. A., Laskar, A. A., Raina, A., Khan, S., Younus, H. (2018). Induced mutation analysis with biochemical and molecular characterization of high yielding lentil mutant lines. International Journal of Biological Macromolecules, 109(4), 167–179. https://doi.org/10.1016/j.ijbiomac.2017.12.067 [Google Scholar] [PubMed] [CrossRef]
15. Ghori, N. H., Ghori, T., Imadi, S. R., Gul, A. (2020). Improving crop health and productivity: Appraisal of induced mutations and advanced molecular genetic tools. In: Hasanuzzaman, M. (Ed.Agronomic crops. Singapore: Springer. https://doi.org/10.1007/978-981-15-0025-1_20 [Google Scholar] [CrossRef]
16. Gottschalk, W., Wolff, G. (2012). Induced mutations in plant breeding. Springer Science & Business Media, pp. 16–17. Springer. https://doi.org/10.1007/978-981-15-0025-1_20 [Google Scholar] [CrossRef]
17. Bermúdez-Caraballoso, I., Cruz-Martín, M., Concepción-Hernández, M. (2020). Biotechnological tools for the development of Foc TR4-resistant or -tolerant Musa spp. cultivars. In: Chong, P., Newman, D., Steinmacher, D. (Eds.Agricultural, forestry and bioindustry biotechnology and biodiscovery, pp. 403–431. Cham: Springer. https://doi.org/10.1007/978-3-030-51358-0_20 [Google Scholar] [CrossRef]
18. Wattoo, J. I., Aslam, K., Shah, S. M., Shabir, G., Sabar, M. et al. (2012). Ethyle methane sulphonate (EMS) induced mutagenic attempts to create genetic variability in Basmati rice. Journal of Plant Breeding and Crop Science, 4(7), 101–105. https://doi.org/10.5897/JPBCS11.080 [Google Scholar] [CrossRef]
19. Singh, H., Khar, A., Verma, P. (2021). Induced mutagenesis for genetic improvement of Allium genetic resources: A comprehensive review. Genetic Resources and Crop Evolution, 68(7), 2669–2690. https://doi.org/10.1007/s10722-021-01210-8 [Google Scholar] [CrossRef]
20. Ahirwar, R. (2016). Ethyl methane sulphonate (EMS) induced translocation and inversion heterozygote in Allium cepa L. Cytologia, 81(2), 149–153. [Google Scholar]
21. Qarachoboogh, A. F., Alijanpour, A., Hosseini, B., Shafiei, A. (2021). Efficient and reliable propagation and rooting of foetid juniper (Juniperus foetidissima Willd.as an endangered plant under in vitro condition. In Vitro Cellular & Developmental Biology-Plant, 1–8. https://doi.org/10.1007/s11627-021-10239-4 [Google Scholar] [CrossRef]
22. Cardoso, J. C., Sheng Gerald, L. T., Teixeira da Silva, J. A. (2018). Micropropagation in the twenty-first century. Plant Cell Culture Protocols, 17–46. https://doi.org/10.1007/978-1-4939-8594-4_2 [Google Scholar] [PubMed] [CrossRef]
23. Vásquez-Hernández, S., Cruz-Cruz, C. A., Santiago-Santiago, M., Bello- Bello, J. J. (2021). Evaluation of different antioxidants during in vitro establishment of allspice (Pimenta dioica L. MerrillA recalcitrant species. Agro Productividad, 14(11), 149–157. https://doi.org/10.32854/agrop.v14i11.2167 [Google Scholar] [CrossRef]
24. Debnath, M., Malik, C. P., Bisen, P. S. (2006). Micropropagation: A tool for the production of high quality plant-based medicines. Current Pharmaceutical Biotechnology, 7(1), 33–49. https://doi.org/10.1007/s00425-018-2910-1 [Google Scholar] [CrossRef]
25. Espinosa-Leal, C. A., Puente-Garza, C. A., García-Lara, S. (2018). In vitro plant tissue culture: Means for production of biological active compounds. Planta, 248(1), 1–18. https://doi.org/10.1007/s00425-018-2910-1 [Google Scholar] [PubMed] [CrossRef]
26. Choudhary, P., Kataria, V. (2022). In vitro culture in combination with aeroponics is an efficient means of mass propagation of Sarcostemma acidum: A rare medicinal plant of Indian arid zone. In Vitro Cellular & Developmental Biology-Plant, 1–10. https://doi.org/10.1007/s11627-021-10245-6 [Google Scholar] [CrossRef]
27. Bayraktar, M., Hayta, S., Parlak, S., Gurel, A. (2015). Micropropagation of centennial tertiary relict trees of Liquidambar orientalis Miller through meristematic nodules produced by cultures of primordial shoots. Trees, 29(4), 999–1009. https://doi.org/10.1007/s00468-015-1179-2 [Google Scholar] [CrossRef]
28. Ekmekçigil, M., Bayraktar, M., Akkuş, Ö., Gürel, A. (2019). High-frequency protocorm-like bodies and shoot regeneration through a combination of thin cell layer and RITA® temporary immersion bioreactor in Cattleya forbesii Lindl. Plant Cell, Tissue and Organ Culture, 136(3), 451–464. https://doi.org/10.1007/s11240-018-1526-2 [Google Scholar] [CrossRef]
29. Khamushi, M., Dehestani-Ardakani, M., Zarei, A., Kamali Aliabad, K. (2019). An efficient protocol for micropropagation of old cypress of Abarkuh (Cupressus sempervirens var. horizontalis [Mill.]) under In vitro condition. Plant Cell, Tissue and Organ Culture, 138(3), 597–601. https://doi.org/10.1007/s11240-019-01645-z [Google Scholar] [CrossRef]
30. Jha, T. B. (2005). Micropropagation. In: Universities Press (Eds.Plant tissue culture: Basic and applied, pp. 44–56. [Google Scholar]
31. Ulvrova, T., Vitamvas, J., Cepkova, P. H., Eliasova, K., Janovska, D. et al. (2021). Micropropagation of an ornamental shrub Disanthus cercidifolius Maxim. and assessment of genetic fidelity of regenerants using ISSR and flow cytometry. Plant Cell, Tissue and Organ Culture, 144(3), 555–566. https://doi.org/10.1007/s11240-020-01978-0 [Google Scholar] [CrossRef]
32. Dreger, M., Gryszczyńska, A., Szalata, M., Wielgus, K. (2020). Micropropagation and HPLC-DAD, UPLC MS/MS analysis of oenothein B and phenolic acids in shoot cultures and in regenerated plants of fireweed (Chamerion angustifolium (L.) Holub). Plant Cell, Tissue and Organ Culture, 143(3), 653–663. [Google Scholar]
33. Reyes-Zambrano, S. J., Lecona-Guzmán, C. A., Barredo-Pool, F. A., Calderón, J. D. A., Abud-Archila, M. et al. (2016). Plant growth regulators optimization for maximize shoots number in Agave americana L. by indirect organogenesis. Gayana Botanica, 73(1), 124–131. [Google Scholar]
34. Millán-Soto, G., Gutiérrez, A., Esqueda, M., Gardea, A. A., Tiznado, M. E. et al. (2016). Respiratory metabolism of Agave angustifolia Haw. clonal lines at different temperatures. Plant Cell, Tissue and Organ Culture, 125(1), 71–80. https://doi.org/10.1007/s11240-015-0930-0 [Google Scholar] [CrossRef]
35. Lecona-Guzmán, C. A., Reyes-Zambrano, S., Barredo-Pool, F. A., Abud-Archila, M., Montes-Molina, J. A. et al. (2017). In vitro propagation of Agave americana by indirect organogenesis. HortScience, 52(7), 996–999. https://doi.org/10.21273/HORTSCI10498-16 [Google Scholar] [CrossRef]
36. Aguilar Jiménez, D. (2018). Micropropagation and acclimatization of Maguey Pitzometl (Agave marmorata Roezl) in the poblana Mixteca. Revista Colombiana de Biotecnología, 20(2), 124–131. [Google Scholar]
37. Nikam, T. D., Mulye, K. V., Chambhare, M. R., Nikule, H. A., Ahire, M. L. (2019). Reduction in hyperhydricity and improvement in in vitro propagation of commercial hard fibre and medicinal glycoside yielding Agave sisalana Perr. ex Engelm by NaCl and polyethylene glycol. Plant Cell, Tissue and Organ Culture, 138(1), 67–78. https://doi.org/10.1007/s11240-019-01603-9 [Google Scholar] [CrossRef]
38. Reyes-Díaz, J. I., Arzate-Fernández, A. M., Piña-Escutia, J. L., Norman-Mondragón, T. H. (2020). The effect of inositol, pyridoxine and thiamine on somatic embryogenesis of Agave angustifolia. Tropical and Subtropical Agroecosystems, 23(1), 1–6. [Google Scholar]
39. Monja-Mio, K. M., Olvera-Casanova, D., Herrera-Herrera, G., Herrera-Alamillo, M.Á., Sánchez-Teyer, F. L. et al. (2020). Improving of rooting and ex vitro acclimatization phase of Agave tequilana by temporary immersion system (BioMINT™). In Vitro Cellular & Developmental Biology-Plant, 56(5), 662–669. https://doi.org/10.1007/s11627-020-10109-5 [Google Scholar] [CrossRef]
40. Chávez-Ortiz, L. I., Morales-Domínguez, J. F., Rodríguez-Sahagún, A., Pérez-Molphe-Balch, E. (2021). In vitro propagation of Agave guiengola Gentry using semisolid medium and temporary immersion bioreactors. Phyton-International Journal of Experimental Botany, 90(3), 1003–1013. https://doi.org/10.32604/phyton.2021.012862 [Google Scholar] [CrossRef]
41. Martinez-Martinez, S. Y., Arzate-Fernandez, A. M., Álvarez-Aragon, C., Martínez-Velasco, I., Norman-Mondragon, T. (2021). Regeneration of Agave marmorata Roelz plants in temporary immersion systems, via organogenesis and somatic embryogenesis. Tropical and Subtropical Agroecosystems, 24(3). https://doi.org/10.56369/tsaes.3472 [Google Scholar] [CrossRef]
42. Monja-Mio, K. M., Olvera-Casanova, D., Herrera-Alamillo, M.Á., Sánchez-Teyer, F. L., Robert, M. L. (2021). Comparison of conventional and temporary immersion systems on micropropagation (multiplication phase) of Agave angustifolia Haw.‘Bacanora’. 3 Biotech, 11(2), 1–8. https://doi.org/10.1007/s13205-020-02604-8 [Google Scholar] [PubMed] [CrossRef]
43. Shimizu-Sato, S., Tsuda, K., Nosaka-Takahashi, M., Suzuki, T., Ono, S. et al. (2020). Agrobacterium-mediated genetic transformation of wild Oryza species using immature embryos. Rice, 13(1), 1–13. https://doi.org/10.1186/s12284-020-00394-4 [Google Scholar] [PubMed] [CrossRef]
44. Hamilton, C. M., Frary, A., Lewis, C., Tanksley, S. D. (1996). Stable transfer of intact high molecular weight DNA into plant chromosomes. Proceedings of the National Academy of Sciences of the United States of America, 93(18), 9975–9979. https://doi.org/10.1073/pnas.93.18.9975 [Google Scholar] [PubMed] [CrossRef]
45. Liu, Y., Liu, G., Yang, Y., Niu, S., Yang, F. et al. (2017). Establishment of an efficient plant regeneration culture protocol and achievement of successful genetic transformation in Jatropha curcas L. Acta Biologica Hungarica, 68(4), 428–442. https://doi.org/10.1556/018.68.2017.4.8 [Google Scholar] [PubMed] [CrossRef]
46. Georgiev, M. I., Agostini, E., Ludwig-Müller, J., Xu, J. (2012). Genetically transformed roots: From plant disease to biotechnological resource. Trends in Biotechnology, 30(10), 528–537. https://doi.org/10.1016/j.tibtech.2012.07.001 [Google Scholar] [PubMed] [CrossRef]
47. Hiebert-Giesbrecht, M. R., Avilés-Berzunza, E., Godoy-Hernández, G., Peña-Rodríguez, L. M. (2021). Genetic transformation of the tropical vine Pentalinon andrieuxii (Apocynaceae) via Agrobacterium rhizogenes produces plants with an increased capacity of terpenoid production. In Vitro Cellular & Developmental Biology-Plant, 57(1), 21–29. https://doi.org/10.1007/s11627-020-10101-z [Google Scholar] [CrossRef]
48. Suárez-González, E. M., Suárez, P. A. P., Cruz-Rubio, J. M., Martínez-Gallardo, N. A., Hernández, I. C. et al. (2016). Differential fructan accumulation and expression of fructan biosynthesis, invertase and defense genes is induced in Agave tequilana plantlets by sucrose or stress-related elicitors. Agri Gene, 2, 17–28. https://doi.org/10.1016/j.aggene.2016.09.003 [Google Scholar] [CrossRef]
49. Al-Mayahi, A. M. W. (2022). The effect of polyamines and silver thiosulphate on micropropagation of date palm followed by genetic stability assessment. World Journal of Microbiology & Biotechnology, 38, 124. https://doi.org/10.1007/s11274-022-03305-5 [Google Scholar] [PubMed] [CrossRef]
50. Utsumi, Y., Utsumi, C., Tanaka, M., Okamoto, Y., Takahashi, S. et al. (2021). Agrobacterium-mediated cassava transformation for the Asian elite variety KU50. Plant Molecular Biology, 1–12. https://doi.org/10.1007/s11103-021-01212-1 [Google Scholar] [PubMed] [CrossRef]
51. Larkin, P. J., Scowcroft, W. R. (1981). Somaclonal variation—A novel source of variability from cell cultures for plant improvement. Theoretical and Applied Genetics, 60(4), 197–214. https://doi.org/10.1007/BF02342540 [Google Scholar] [PubMed] [CrossRef]
52. Chawla, H. (2011). Plant tissue culture. In: CRC Press (Eds.Introduction to plant biotechnology, pp. 3–156. https://doi.org/10.1201/9781315275369 [Google Scholar] [CrossRef]
53. Siddique, I., Yadav, V. (2021). Cytokinin influence on micropropagation system of Dianthus caryophyllus L. In: Siddique, I. (Ed.Propagation and genetic manipulation of plants, pp. 33–41. Singapore: Springer. https://doi.org/10.1007/978-981-15-7736-9_3v [Google Scholar] [CrossRef]
54. Pawełkowicz, M. E., Skarzyńska, A., Mróz, T., Bystrzycki, E., Pląder, W. (2021). Molecular insight into somaclonal variation phenomena from transcriptome profiling of cucumber (Cucumis sativus L.) lines. Plant Cell, Tissue and Organ Culture, 145(2), 239–259. https://doi.org/10.1007/s11240-020-02005-y [Google Scholar] [CrossRef]
55. Cao, Z., Sui, S., Cai, X., Yang, Q., Deng, Z. (2016). Somaclonal variation in ‘Red Flash’caladium: Morphological, cytological and molecular characterization. Plant Cell, Tissue and Organ Culture, 126(2), 269–279. https://doi.org/10.1007/s11240-016-0996-3 [Google Scholar] [CrossRef]
56. Xu, L., Najeeb, U., Naeem, M. S., Wan, G. L., Jin, Z. L. et al. (2012). In vitro mutagenesis and genetic improvement. In: Gupta, S. (Ed.) Technological innovations in major world oil crops, pp. 151–173. New York, NY: Springer. https://doi.org/10.1007/978-1-4614-0827-7_6 [Google Scholar] [CrossRef]
57. García-Velasco, R., Portal-González, N., Santos-Bermúdez, R., Rodríguez-García, A., Companioni-González, B. (2021). Mejoramiento genético para la resistencia a marchitez por Fusarium en banano. Revista mexicana de fitopatología, 39(1), 122–146. https://doi.org/10.18781/r.mex.fit.2008-2 [Google Scholar] [CrossRef]
58. Yaqoob, M. (2001). Induced mutation studies in some mungbean (Vigna radiata L.) wilczek cultivars. Online Journal of Biological Sciences, 1, 805–808. [Google Scholar]
59. Martirena-Ramírez, A., Veitía, N., García, L. R., Collado, R., Torres, D. et al. (2018). Dosis óptima de radiaciones Gamma para la regeneración de plantas in vitro de Phaseolus vulgaris L. cultivar ‘BAT-93’. Biotecnología Vegetal, 18(1), 21–29. [Google Scholar]
60. Türkoğlu, A., Tosun, M., Haliloğlu, K. (2022). Mutagenic effects of sodium azide on in vitro mutagenesis, polymorphism and genomic instability in wheat (Triticum aestivum L.). Molecular Biology Reports, 1–10. https://doi.org/10.1007/s11033-022-07896-y [Google Scholar] [PubMed] [CrossRef]
61. Jankowicz-Cieslak, J., Till, B. J. (2016). Chemical mutagenesis of seed and vegetatively propagated plants using EMS. Current Protocols in Plant Biology, 1(4), 617–635. https://doi.org/10.1002/cppb.20040 [Google Scholar] [PubMed] [CrossRef]
62. Masoabi, M., Lloyd, J., Kossmann, J., van der Vyver, C. (2018). Ethyl methanesulfonate mutagenesis and in vitro polyethylene glycol selection for drought tolerance in sugarcane (Saccharum spp.). Sugar Tech, 20(1), 50–59. https://doi.org/10.1007/s12355-017-0524-8 [Google Scholar] [CrossRef]
63. Kashtwari, M., Mansoor, S., Wani, A. A., Najar, M. A., Deshmukh, R. K. et al. (2021). Random mutagenesis in vegetatively propagated crops: Opportunities, challenges and genome editing prospects. Molecular Biology Reports, 1–2. https://doi.org/10.1007/s11033-021-06650-0 [Google Scholar] [PubMed] [CrossRef]
64. Maghuly, F., Bado, S., Jankowicz-Cieslak, J., Laimer, M., Jankowicz-Cieslak, J. et al. (2017). Chemical and physical mutagenesis in Jatropha curcas. In: Jankowicz-Cieslak, J., Laimer, M. (Eds.Biotechnologies for plant mutation breeding, pp. 21–38. Cham: Springer. https://doi.org/10.1007/978-3-319-45021-6_2 [Google Scholar] [CrossRef]
65. Patade, V. Y., Suprasanna, P., Bapat, V. A. (2008). Effects of salt stress in relation to osmotic adjustment on sugarcane (Saccharum officinarum L.) callus cultures. Plant Growth Regulation, 55(3), 169–173. https://doi.org/10.1007/s10725-008-9270-y [Google Scholar] [CrossRef]
66. Dalvi, S. G., Tawar, P. N., Suprasanna, P., Dixit, G. B., Prasad, D. T. (2021). EMS-based in vitro mutagenesis and mutant screening for smut resistance with agronomic traits in sugarcane. Sugar Tech, 23(4), 854–864. https://doi.org/10.1007/s12355-020-00931-x [Google Scholar] [CrossRef]
67. Ángeles-Espino, A., Virgen-Calleros, G., Valencia-Botín, J., Ramírez-Serrano, C., Paredes-Gutiérrez, L. (2016). Nondestructive evaluation of pathogenicity of Cercospora agavicola on agave azul tequilero plantlets irradiated with gamma rays Co60. Acta Universitaria, 26(6), 3–10. [Google Scholar]
68. Motero Paredes, T., Vitoria Narvaez, M., Vitoria Fernández, E. (2018). Induction of polyploidy with colchicine in vitro plants of Aloe vera (L.). Revista Colombiana de Biotecnología, 20(1), 97–105. [Google Scholar]
69. Reyes-Zambrano, S. J., Ramírez-Merchant, M. L., Arias-Castro, C., Rodríguez-Mendiola, M. A., Lecona-Guzmán, C. A. et al. (2019). Morphometric and biochemical changes in Agave americana L. plantlets induced by ethyl methanesulfonate. Phyton-International Journal of Experimental Botany, 88(3), 277–284. https://doi.org/10.32604/phyton.2019.06504 [Google Scholar] [CrossRef]
70. Gómez, D., Hernández, L., Martínez, J., Escalante, D., Zevallos, B. E. et al. (2019). Sodium azide mutagenesis within temporary immersion bioreactors modifies sugarcane in vitro micropropagation rates and aldehyde, chlorophyll, carotenoid, and phenolic profiles. Acta Physiologiae Plantarum, 41(7), 1–7. https://doi.org/10.1007/s11738-019-2911-0 [Google Scholar] [CrossRef]
71. Espino, A. A., Botín, A. J. V., Serrano, C. R., Calleros, G. V., Barrios, E. P. et al. (2020). Indirect somatic embryogenesis on mutants of Agave tequilana weber cultivar blue induced with CO60 gamma rays. Tropical and Subtropical Agroecosystems, 23(3), 1–9. [Google Scholar]
72. Sharma, V., Thakur, M., Tomar, M. (2020). In vitro selection of gamma irradiated shoots of ginger (Zingiber officinale Rosc.) against Fusarium oxysporum f. sp. zingiberi and molecular analysis of the resistant plants. Plant Cell, Tissue and Organ Culture, 143(2), 319–330. https://doi.org/10.1007/s11240-020-01919-x [Google Scholar] [CrossRef]
73. Sewelam, N., Kazan, K., Schenk, P. M. (2016). Global plant stress signaling: Reactive oxygen species at the cross-road. Frontiers in Plant Science, 7, 187. https://doi.org/10.3389/fpls.2016.00187 [Google Scholar] [PubMed] [CrossRef]
74. Rai, K. K., Rai, N., Rai, S. P. (2018). Recent advancement in modern genomic tools for adaptation of Lablab purpureus L to biotic and abiotic stresses: Present mechanisms and future adaptations. Acta Physiologiae Plantarum, 40(9), 1–29. https://doi.org/10.1007/s11738-018-2740-6 [Google Scholar] [CrossRef]
75. Sharma, P., Sharma, M. M. M., Malik, A., Vashisth, M., Singh, D. et al. (2021). Rhizosphere, rhizosphere biology, and rhizospheric engineering. In: Mohamed, H. I., El-Beltagi, H. E. D. S., Abd-Elsalam, K. A. (Eds.Plant growth-promoting microbes for sustainable biotic and abiotic stress management, pp. 577–624. Cham: Springer. https://doi.org/10.1007/978-3-030-66587-6_21 [Google Scholar] [CrossRef]
76. Anzano, A., Bonanomi, G., Mazzoleni, S., Lanzotti, V. (2021). Plant metabolomics in biotic and abiotic stress: A critical overview. Phytochemistry Reviews, 1–2. https://doi.org/10.1007/s11101-021-09786-w [Google Scholar] [CrossRef]
77. Doğru, A. (2021). Effects of heat stress on photosystem II activity and antioxidant enzymes in two maize cultivars. Planta, 253(4), 1–15. https://doi.org/10.1007/s00425-021-03611-6 [Google Scholar] [PubMed] [CrossRef]
78. Schulze, E. D., Beck, E., Buchmann, N., Clemens, S., Müller-Hohenstein, K. et al. (2019). General themes of molecular stress physiology. In: Plant ecology, pp. 9–55. Berlin, Heidelberg: Springer. https://doi.org/10.1007/978-3-662-56233-8_2 [Google Scholar] [CrossRef]
79. Czarnocka, W., Karpiński, S. (2018). Friend or foe? Reactive oxygen species production, scavenging and signaling in plant response to environmental stresses. Free Radical Biology and Medicine, 122, 4–20. https://doi.org/10.1016/j.freeradbiomed.2018.01.011 [Google Scholar] [PubMed] [CrossRef]
80. Ma, L., Zhang, Y., Meng, Q., Shi, F., Liu, J. et al. (2018). Molecular cloning, identification of GSTs family in sunflower and their regulatory roles in biotic and abiotic stress. World Journal of Microbiology and Biotechnology, 34(8), 1–12. https://doi.org/10.1007/s11274-018-2481-0 [Google Scholar] [PubMed] [CrossRef]
81. Jain, A., Joshi, G., Chauhan, C., Das, S. (2018). Abiotic stress response in plants: A cis-regulatory perspective. In: Zargar, S., Zargar, M. (Eds.Abiotic stress-mediated sensing and signaling in plants: An omics perspective, pp. 185–203. Singapore: Springer. https://doi.org/10.1007/978-981-10-7479-0_6 [Google Scholar] [CrossRef]
82. Akram, R., Fahad, S., Masood, N., Rasool, A., Ijaz, M. et al. (2019). Plant growth and morphological changes in Rice under abiotic stress. In: Mirza, H., Masayuki, F., Kamrun, N., Jiban, K. B. (Eds.Advances in rice research for abiotic stress tolerance, vol. 4, pp. 69–85. Woodhead. https://doi.org/10.1016/B978-0-12-814332-2.00004-6 [Google Scholar] [CrossRef]
83. Dixit, S., Singh, U. M., Singh, A. K., Alam, S., Venkateshwarlu, C. et al. (2020). Marker assisted forward breeding to combine multiple biotic-abiotic stress resistance/tolerance in rice. Rice, 13(1), 1–15. https://doi.org/10.1186/s12284-020-00391-7 [Google Scholar] [PubMed] [CrossRef]
84. Vishwakarma, K., Mishra, M., Patil, G., Mulkey, S., Ramawat, N. et al. (2019). Avenues of the membrane transport system in adaptation of plants to abiotic stresses. Critical Reviews in Biotechnology, 39(7), 861–883. https://doi.org/10.1080/07388551.2019.1616669 [Google Scholar] [PubMed] [CrossRef]
85. Wang, F., Deng, M., Xu, J., Zhu, X., Mao, C. (2018). Molecular mechanisms of phosphate transport and signaling in higher plants. Seminars in Cell & Developmental Biology, 74, 114–122. https://doi.org/10.1016/j.semcdb.2017.06.013 [Google Scholar] [CrossRef]
86. Tan, P., Du, X., Shang, Y., Zhu, K., Joshi, S. et al. (2021). Ion transporters and their exploration for conferring abiotic stress tolerance in plants. Plant Growth Regulation, 1–23. https://doi.org/10.1007/s10725-021-00762-0 [Google Scholar] [CrossRef]
87. Vo, K. T. X., Rahman, M. M., Rahman, M. M., Trinh, K. T. T., Kim, S. T. et al. (2021). Proteomics and metabolomics studies on the biotic stress responses of rice: An update. Rice, 14(1), 1–16. https://doi.org/10.1186/s12284-021-00461-4 [Google Scholar] [PubMed] [CrossRef]
88. Hasan, M. M., Rafii, M. Y., Ismail, M. R., Mahmood, M., Rahim, H. A. et al. (2015). Marker-assisted backcrossing: A useful method for rice improvement. Biotechnology & Biotechnological Equipment, 29(2), 237–254. https://doi.org/10.1080/13102818.2014.995920 [Google Scholar] [PubMed] [CrossRef]
89. Wu, X., Gong, F., Cao, D., Hu, X., Wang, W. (2016). Advances in crop proteomics: PTMs of proteins under abiotic stress. Proteomics, 16(5), 847–865. https://doi.org/10.1002/pmic.201500301 [Google Scholar] [PubMed] [CrossRef]
90. Wu, Y., Mirzaei, M., Haynes, P. A. (2017). Proteomics of rice-our most valuable food crop proteomics. In: Proteomics in food science, pp. 17–33. Academic Press. https://doi.org/10.1016/B978-0-12-804007-2.00002-3 [Google Scholar] [CrossRef]
91. Meng, Q., Gupta, R., Min, C. W., Kwon, S. W., Wang, Y. et al. (2019). Proteomics of rice—Magnaporthe oryzae interaction: What have we learned so far? Frontiers in Plant Science, 10, 1383. https://doi.org/10.3389/fpls.2019.01383 [Google Scholar] [PubMed] [CrossRef]
92. Santamaria, M. E., Martínez, M., Cambra, I., Grbic, V., Diaz, I. (2013). Understanding plant defence responses against herbivore attacks: An essential first step towards the development of sustainable resistance against pests. Transgenic Research, 22, 697–708. https://doi.org/10.1007/s11248-013-9725-4 [Google Scholar] [PubMed] [CrossRef]
93. Singh, P., Mukhopadhyay, K. (2021). Comprehensive molecular dissection of TIFY Transcription factors reveal their dynamic responses to biotic and abiotic stress in wheat (Triticum aestivum L.). Scientific Reports, 11, 9739. https://doi.org/10.1038/s41598-021-87722-w [Google Scholar] [PubMed] [CrossRef]
94. Dickinson, C. H., Lucas, J. A. (1998). Plant disease. In: Wiley, J., Sons (Eds.Plant pathology and plant pathogens. [Google Scholar]
95. Anaya-Lang, A. L. (2016). Ecología química y alelopatía : una introducción. In: Anaya Lang, A. L., Espinosa García, F. J., Roger, R., Manuel, J. (Eds.Ecología química y alelopatía : avances y perspectivas, pp. 15–68. [Google Scholar]
96. Rodríguez-Garay, B. (2016). Somatic embryogenesis in Agave spp. In: Loyola-Vargas, V., Ochoa-Alejo, N. (Eds.Somatic embryogenesis: fundamental aspects and applications, pp. 267–282. Cham: Springer. https://doi.org/10.1007/978-3-319-33705-0_16 [Google Scholar] [CrossRef]
97. Trejo, L., Reyes, M., Cortés-Toto, D., Romano-Grande, E., Muñoz-Camacho, L. L. (2020). Morphological diversity and genetic relationships in pulque production agaves in Tlaxcala, Mexico, by means of unsupervised learning and gene sequencing analysis. Frontiers in Plant Science, 11, 524812. https://doi.org/10.3389/fpls.2020.524812 [Google Scholar] [PubMed] [CrossRef]
98. Díaz-Martínez, M., Nava-Cedillo, A., Guzmán-López, J. A., Escobar-Guzmán, R., Simpson, J. (2012). Polymorphism and methylation patterns in Agave tequilana Weber gvar.‘Azul’ plants propagated asexually by three different methods. Plant Science, 185, 321–330. https://doi.org/10.1016/j.plantsci.2012.01.004 [Google Scholar] [PubMed] [CrossRef]
99. Tejavathi, D. H., Gayathramma, K., Nijagunaiah, R. (2012). Genetic variability by in vitro mutagenesis and arbuscular mycorrhizal fungal symbiosis among micropropagated plants of Agave vera-cruz miller. Bioremediation, Biodiversity and Bioavailability, 6, 665–669. [Google Scholar]
100. Reyes-Zambrano, S. J., Lecona-Guzmán, C. A., Gutiérrez-Miceli, F. A., Santana-Buzzy, N., Islas-Flores, I. (2020). Scanning electron microscopy and enzymatic analysis in Agave americana during Fusarium oxysporum infection. Revista Mexicana de Fitopatología, 38(3), 408–419. https://doi.org/10.18781/r.mex.fit.2005-3 [Google Scholar] [CrossRef]
101. Reyes-Zambrano, S. J., Lecona-Guzmán, C. A., Gutiérrez-Miceli, F. A., Ruiz-Valdiviezo, V. M., Vargas-Díaz, A. A. (2021). Selection and analysis of polymorphisms in somaclonal variants of Agave americana resistant to Fusarium oxysporum via an ethyl methanesulphonate treatment. Phyton-International Journal of Experimental Botany, 90(6), 1727–1739. https://doi.org/10.32604/phyton.2021.016171 [Google Scholar] [CrossRef]
Cite This Article
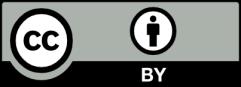