Open Access
ARTICLE
The Function of GABA in Plant Cell Growth, Development and Stress Response
1
Shanghai Key Laboratory of Bio-Energy Crops, Research Center for Natural Products, Plant Science Center, School of Life Sciences,
Shanghai University, Shanghai, 200444, China
2
School of Applied Sciences, College of Health, Science and Society, University of the West of England, Bristol, UK
* Corresponding Author: Xiangyang Hu. Email:
(This article belongs to the Special Issue: Abiotic and Biotic Stress Tolerance in Crop)
Phyton-International Journal of Experimental Botany 2023, 92(8), 2211-2225. https://doi.org/10.32604/phyton.2023.026595
Received 14 September 2022; Accepted 24 April 2023; Issue published 25 June 2023
Abstract
Gamma-aminobutyric acid (GABA) is a ubiquitous four-carbon non-protein amino acid that is involved in various physiological processes of plant growth and development, such as root architecture, stem elongation, leaf senescence, pollen tube growth, fruit ripening, and seed germination. GABA is also related to plant stress responses, such as drought, salt, cold, and heat stresses. Regulation of GABA in plant stress responses is complex and involves multiple signaling pathways, including calcium and hormone signaling. This paper systematically reviews the synthesis, metabolic pathways and regulatory role of GABA in plants, which will provide new insights into the understanding of plant growth and stress responses and offer novel strategies for improving crop productivity and stress.Keywords
Gamma-aminobutyric acid (GABA) is a non-proteinogenic amino acid, that is widespread in vertebrates, plants and microorganisms. In 1950, it was discovered that GABA functions as a neurotransmitter and an inhibitory signal in the central nervous system, regulating neuronal excitability in mammals, insects, and some parasitic worms [1–8]. In plants, GABA was first identified in the tuber of potato (Solanum Tuberosum) over 70 years ago [9–13]. GABA metabolism in plants involves a short pathway that bypasses two steps of the tricarboxylic acid cycle (TCA). The first step involves the calcium-calmodulin (CaM) complex-regulated enzyme glutamate decarboxylase (GAD), which catalyzes the irreversible decarboxylation of glutamate to produce GABA. GABA is then transported to the mitochondria, where it is converted to succinate semialdehyde by GABA transaminase, using alpha-ketoglutarate (by GABA-TK) or pyruvate (by GABA-TP) as amino acid receptors. Succinate semialdehyde is subsequently reduced to succinate by succinate semialdehyde dehydrogenase (SSADH), and gets into the TCA cycle. This metabolic pathway, called the GABA shunt, is controlled by GAD, pyruvate and 2-oxoglutarate-dependent GABA transaminase (GABA-T) and SSA dehydrogenase (SSADH). GAD is considered the rate-limiting enzyme of GABA synthesis in plants, requiring pyridoxal phosphate (PLP) as a co-factor. GAD in plants contains a calmodulin (CaM) binding domain, and GAD activity is regulated by the concentration of Ca2+ and H+. Recent research indicates that the GABA branch is the main pathway for GABA synthesis in plants [3,4,6,14].
Another pathway for GABA synthesis is through the polyamine metabolic pathway, where arginine is converted into putrescine through a multi-step process. Putrescine is then converted to 4-aminobutyraldehyde by oxygen-dependent polyamine oxidase, or spermidine, which is subsequently degraded to 4-aminobutyraldehyde. The resulting 4-aminobutyraldehyde is then converted to NAD+-dependent 4-aminobutyraldehyde, which is further oxidized to GABA by aldehyde dehydrogenase. GABA then enters the TCA cycle and is degraded by GABA-T and SSADH [3,15]. The detailed pathway is illustrated in Fig. 1.
Figure 1: The metabolic pathway of GABA in plants. Left panel: the pathway of TCA circle for GABA biosynthesis; Right panel: the polyamine pathway for GABA biosynthesis. SSA: succinate semialdehyde; α-KG: α-ketoglutarate; SSADH: succinate semialdehyde dehydrogenase; PAO: polyamine oxidase
2 GABA as an Important Signaling Regulator in Plants
The adaptation of plants to unstable environments depends on their capacity to sense and respond to their surroundings, generating and transmitting corresponding signals to different parts of the plant, thereby triggering the changes necessary to optimize growth and defense responses. In animals, GABA has been studied for over 60 years as a signaling molecule. In mammals, GABA activates GABAA or GABAB receptors to open channels. GABAA receptors are chloride channels, while GABAB receptors regulate cation transport such as potassium (K+) and calcium (Ca2+) through G protein-coupled receptors. GABA also has an important role in the proliferation, differentiation, and migration of different types of cells in animals. In plants, GABA is primarily identified as a carbon and nitrogen metabolite. However, since the 1990s, numerous studies have demonstrated that GABA can act as a signaling molecule in plants. A breakthrough discovery was the identification of ALMTs (Aluminum-activated malate transporters) as a real GABA target protein. ALMTs encode an anion channel responsible for the Al-activated malate extrusion from root tips. ALMTs constitute a multi-gene protein family expressed differentially in plant tissues. Although plant action potential mainly relies on the activation of voltage-dependent anion channels, the anion concentrations are required to activate ALMTs. Inhibition of ALMTs by GABA results in hyperpolarization of the membrane potential and reduced excitability, similar to the effect of GABA on animal neurons [16,17]. It has been indicated that TaALMTs may act as both GABA and anion transporters in wheat. GABA and malate simultaneously interact with TaALMTs in a complicated pattern. Physiological and inhibitory experiments show that GABA can be imported by TaALMT1, which can be blocked by external malate and aluminon ions. However, GABA can also be expelled by TaALMT1 under acid environment, a process activated by aluminon ions, or presence of anions (such as malate or sulfate ions) on the outside of high alkali environments. This ensures the balance of malic acid outflow and intracellular GABA levels, sustaining the homeostasis of these molecules [7,17,18].
Besides ALMT-dependent ion transport, GABA is also believed to affect the flux of other ions. For instance, GABA treatment in tobacco is thought to increase the influx of Ca2+ through the pollen tube channel, which may play a role in guiding the pollen tube to the ovaries [19]. At present, although the application of exogenous GABA to plants significantly affects the plant transcriptome, the GABA-related signaling pathway that directly affects gene expression remains unidentified [20,21]. Interestingly, in bacteria such as Bacillus subtilis, the transcriptional activity of GabR protein is directly regulated by GABA. GabR is a chimeric protein containing a HTH DNA-binding domain and a long aminotransferase-like PLP-binding domain, which alone acts as a negative transcriptional factor to repress its own expression. However, in the presence of GABA, GabR specifically activates transcription of the gabTD operon, encoding enzymes of GABA catabolism that convert GABA to succinic-semialdehyde and then to succinate [22]. It is an interesting topic to explore whether similar protein motifs may exist in plant transcription factors that transduce GABA signaling.
3 Function of GABA during Plant Growth and Development
GABA has been demonstrated to play a role in the growth and development of various plant tissues and organs. However, most existing research focuses on GABA’s regulation of plant stress responses, with relatively few studies addressing its role in plant growth and development under normal conditions. According to the available literature, GABA influences the growth and development of plant stems, pollen tubes, and root architecture. Additionally, GABA plays a crucial role in regulating fruit ripening and responding to leaf senescence [6].
Glutamate decarboxylase (GAD) is an enzyme that catalyzes the decarboxylation of glutamic acid into carbon dioxide (CO2) and gamma-aminobutyric acid (GABA). GAD is present in both prokaryotes and eukaryotes, but only plant GAD has evolved to bind specifically to calmodulin (CaM). The binding of CaM to GAD is essential for the enzyme’s activity [23]. For instance, transgenic tobacco plants overexpressing a truncated version of GAD, which lacks the CaM binding region, exhibit abnormal growth patterns. These plants demonstrate significantly elevated GABA levels and reduced glutamate content compared to non-transgenic counterparts. In contrast, transgenic tobacco overexpressing normal GAD exhibits indistinguishable growth and development from the wild-type line. Additional calcium chelator EGTA, or the CaM antagonist trifluoperazine can dramatically suppress the activity of GAD and the accumulation of GABA [14,23]. These findings suggest that the CaM/GAD complex regulates the dynamic level of GABA by sensing intracellular calcium signals for plant growth and development.
It is well established that ethylene induces a reduction in stem elongation, swelling of the hypocotyl, and a change in the direction of growth, referred to as the triple response [21]. Exogenous GABA treatment also strongly induces the release of ethylene in sunflower tissue. Ethylene synthesis in higher plants requires the catalysis of two enzymes, 1-aminocyclopropane-1-carboxylic acid (ACC) synthase and ACC oxidase [24]. In most plant tissues, ACC synthase is considered the rate-limiting step in ethylene biosynthesis. GABA-induced ethylene production in sunflower is primarily achieved by upregulating the transcriptional abundance of ACC synthase. Conversely, GABA-induced production of ethylene can be repressed by additional ACC inhibitors, such as AVG or AIBA, confirming that ACC is mainly responsible for ethylene biosynthesis after GABA stimulation [25].
Recent studies have provided compelling evidence indicating that GABA gradients play a key role in the normal growth and guidance of pollen tubes in female tissues, enabling precise targeting of pollen tubes for the delivery of sperm cells [26]. It has been observed that the Arabidopsis gene pop2 mediates the guidance of pollen tubes and is essential for self-fertilization of diploid germ cells [26]. Through the study of wild-type Arabidopsis and pop2 mutants, we found that the pop2 mutation resulted in an elevation in endogenous GABA content of Arabidopsis thaliana, leading to physiological defects such as pollen tube elongation, directional induction, and fertilization. Upon exogenous application of GABA, the pop2 mutant showed a similar response to that of the wild-type, but the response was more sensitive. When the pollen of the pop2 mutant was transplanted on the pistil of the wild type plant with a low GABA concentration, the pollen grew normally, but the length of the pollen tube was inhibited in the presence of high GABA concentrations in the pistil of the pop2 mutant. Meanwhile, a substantial amount of GABA accumulated in the pistils of pop2 mutants, inhibiting the extension of some pollen tubes, indicating that the endogenous concentration of GABA affects the normal growth and extension of pollen tubes, with low concentrations of GABA promoting the growth and induction of pollen tubes, while high concentrations of GABA inhibit them [15,27].
Adventitious roots are critical for plant growth and adaptation to various environmental conditions. The formation of adventitious roots is regulated by both exogenous and endogenous factors, including hormones as well as carbon/nitrogen metabolism, which is also associated with gamma-aminobutyric acid (GABA) shunting [28–30]. Previous studies have demonstrated that inhibition of GABA-T activity by a specific inhibitor (vigabatrin; VGB) resulted in increased endogenous GABA content and decreased primary root growth in Brassica napus [31]. Moreover, the transgenic poplar overexpressing PagGAD2 to elevate endogenous GABA level, direct application of exogenous GABA, or inhibition of GABA degradation also negatively regulate adventitious root formation and growth, which is closely related to changes in carbon/nitrogen metabolism and hormonal signaling. These findings further confirm the critical role of GABA in adventitious root formation [32]. Another study found that exogenous GABA can significantly increase the content of endogenous GABA in Arabidopsis thaliana, leading to the inhibition of hypocotyl and primary root growth, as well as leaf chlorosis [33,34]. Through microarray data analysis, the authors showed that the expression of genes encoding cell wall secretion-related proteins decreased in response to GABA in plant tissues, and the types of genes whose expression was reduced in pop2 mutants [35], similar to those in wild-type plants treated with exogenous GABA [32]. Furthermore, GABA can promote the formation of plant mycorrhiza. GABA is involved in ectomycorrhizal symbiosis by increasing synthesis and inhibiting mycorrhizal degradation [36], suggesting a significant impact on the growth and development of plant roots.
Fruit ripening is often accompanied by a series of complex physiological and biochemical processes, and ethylene is produced in large amounts during ripening. It is then sensed by a series of receptors such as ERS and ETR, and the downstream ethylene-related genes are regulated through signal cascades, leading to physiological and biochemical changes, such as the conversion of starch into sugar and the degradation of polyphenols [37–39]. The biosynthesis of ethylene is regulated by enzymes such as ACS and ACO, and GABA has been shown to induce the ACC synthase gene expression, a precursor of ethylene synthesis, which promotes ethylene synthesis. Studies have also found that GABA can induce banana fruit ripening by upregulating the MaGAD1 gene and positively regulating ethylene biosynthesis [40]. These findings suggest that GABA accumulation during fruit ripening could accelerate the ripening process without affecting fruit quality.
Senescence is the final step in leaf development, leading to organ death through degradation and recycling of organic matter. During senescence, nutrients such as proteins, lipids, and nucleic acids synthesized in leaves are degraded and redistributed to growth parts and reproductive organs for fruit and seed development [41]. GABA has been shown to play a role in enhancing leaf viability under stress conditions, as evidenced by the GABA transaminase knock out mutants pop2–1 and pop2–3, which showed precocious leaf senescence, decreased leaf photosynthesis capabilities, reduced chlorophyll content in leaves and lowered GABA levels under stress. Conversely, ion leakage and malondialdehyde (MDA) level in stress-induced leaves of the wild type were reduced. This evidence illustrates the function of GABA in enhancing leaf viability under stress conditions [42].
About 70% of plant nitrogen is stored in chloroplasts, and nitrogen is a vital metabolic nutrient in senescent leaves. The main forms of inorganic nitrogen available to plants are NO3− and NH4+, which are crucial for the glutamine synthase/glutamate synthase (GS/GOGAT) cycle in nitrogen assimilation. C abiotic stress during leaf senescence can inhibit the GS/GOGAT cycle. Thereby limiting chlorophyll synthesis [43]. Non-protein amino acids, such as proline (an antioxidant) and gamma-aminobutyric acid (GABA), accumulate under various abiotic stresses and enhance stress tolerance, including during high temperatures [44,45]. GABA, proline, and nitrogen have been found to regulate and inhibit heat-induced leaf senescence by preventing chlorophyll degradation through the inhibition of chlorophyllase activity [46]. Plant peptide hormone phytosulfokine alpha (PSKα) delays leaf senescence by reinforcing SUMO1/SUMO E3 ligase SIZ1 signaling pathways in cut rose flowers. This process is accompanied by the accumulation of endogenous GABA, which enhances the activities of intracellular molecular chaperones and protein repair systems while suppressing ethylene biosynthesis and chlorophyll degradation [47,48]. Overall, GABA plays an essential role in plant senescence by improving leaf viability under stress conditions, preventing chlorophyll degradation, and enhancing stress tolerance.
Several recent studies have indicated that plant growth regulators (PGRs) may induce physiological tolerance in plants subjected to adverse environmental conditions, including drought. Abiotic stress factors, such as the drought, extreme temperature, nutrient deficiency, salinity, and heavy metal, as well as biotic stress factors, such as diseases, pests, and weeds, pose significant threats to food security and plant production. Consequently, investigating plant growth regulators is of considerable importance. Among the PGRs that have been tested on various plant species, GABA has emerged as a promising multifaceted protector, swiftly inducing plant responses to multiple abiotic stresses, such as wounding tolerance or irritation, hormonal toxicity, flooding, extreme temperatures, heat shock, salt, and/or drought stress [21,30,49–51].
Water availability is a crucial factor influencing plant productivity; consequently, when water is insufficient in the soil and atmospheric conditions cause a continuous loss of water, drought stress occurs. Water shortage is one of the most pressing issues in plant biology, as it affects plant growth and development, and productivity. Climate change and human activities exacerbate drought disasters, directly limiting crop growth, grain yield, and significantly impacting economic development and food security [49]. The stomatal pore aperture regulation is a key determinant of plant productivity and drought resilience, and profoundly impacts global carbon and water cycling [52]. GABA has been found to inhibit stomatal opening under light by negatively regulating the aluminum activated malate transporter, ALMT9. Additionally, GABA can reduce the anion flowing into the vacuole mediated by ALMT9, thus inhibiting the stomatal opening, enhancing water use efficiency, and increase drought resistance in plant. When a mutation is present in the GAD2 gene, the activity of ALMT9 increases, which leads to the increase of stomatal conductance and water loss, thus making the plant more sensitive to drought. Under drought conditions, increased GABA content reduces ALMT9 activity, which helps decrease stomatal conductance, improve water use efficiency, and enhance drought resistance in plants [53]. As a result, GABA synthesis and accumulation in leaves contribute to plant drought resistance by inhibiting stomatal opening through the inhibition of ALMT9 [38,52]. It is known that peroxidase (POD) and catalase (CAT) enzymes play a role in scavenging hydrogen peroxide (H2O2), while superoxide dismutase (SOD) is involved in the dismutation of superoxide radicals (O2−) to H2O2 and molecular oxygen (O2) in plant cells. In carrot, exogenous GABA spraying may be an effective production strategy to scavenge overproduced H2O2 under water-deficient conditions by increasing CAT activity [54].
Flooding or oxygen deprivation is a prevalent environmental stress that reduces crop yields. Global warming helps to an increase in flooding events [55]. Short-term exposure to flooding causes physiological changes in plants, such as decreased oxidative phosphorylation, a shift to anaerobic respiration, reduced nucleoside triphosphates, and increased NADH to NAD+ ratios. Furthermore, hypoxia disrupts the normal physiological metabolism of plants by reducing available energy [56]. Studies have established that GABA may regulate cytoplasmic pH, maintain nitrogen/carbon flux, the tricarboxylic acid cycle, or increase antioxidant capacity to enhance flood stress tolerance in tobacco [57]. In Arabidopsis, the GAD gene family composes of five members. It has been found that tobacco GAD is also the main enzyme responsible for GABA biosynthesis under flood stress because inhibition of GAD activity by methylparaben (MPA) or dithiobis nitrobenzoic acid (DTNB) also significantly reduced GABA accumulation under flood stress [58] (Fig. 2).
Figure 2: GABA as the central hub enhance plant tolerance to biotic stress and various environmental stress, including chilling, saline, heat, flooding and drought stress. We also propose the possible mechanism for GABA in enhancing plant tolerance to different environment and biotic stress in the bright blue box
4.2 Chilling and High Temperature
Heat stress is emerging as a major determinant of the production potential of various cool-season and summer crops due to gradual global and local temperature increases. Heat stress impairs plant growth and development, leading to significant changes in phenology, morphology, physiology, biochemistry, and gene expression, ultimately inhibiting the productive potential of affected crops [50,59].
Mung bean (Vigna radiata L.) is a nutrient-rich summer legume that often faces heat stress during its reproductive phase, resulting in significantly reduced flowers, poor pod setting, reduced pod size, pod and seed numbers, and seed yield. Studies have shown that treating heat-stressed mung bean plants with GABA can increase carbon fixation and assimilation, enhance sucrose synthesis in leaves, and possibly transport sucrose into flowers to maintain reproductive function, thereby increasing flower and pod retention [60]. Thus, this shows the effectiveness of GABA as a thermal protectant. Furthermore, application of GABA to heat-stressed plants improved leaf water status, potentially due to the upregulation of enzymes that synthesize osmotic substances like proline and trehalose. Additionally, GABA also helps decrease oxidative damage to reproductive components in heat-stressed plants [49,61].
The temperature of the earth’s surface is highly variable, changing with the seasons, and during the day and night, and being influenced by weather. Plants must adapt to these fluctuations to survive and thrive. In plants, low-temperature (usually ranging from 0°C to 15°C) during germination and early seedling growth can have a significant impact on plant productivity, causing membrane damage, reactive oxygen species (ROS) production, protein denaturation, and accumulation of toxic compounds at the cellular level [50,62]. Low temperatures at an early stage may result in reduced germination, poor seedling establishment, stunted seedlings, yellowing, or withering [62]. In response to low temperatures, several plants have evolved acclimation mechanisms to increase their antioxidant capacity. One such mechanism involves GABA, which has been shown to alleviate low-temperature stress in plants such as tomato seedlings. Exogenous application of GABA helps maintain membrane stability by reducing the accumulation of malondialdehyde (MDA), a byproduct of lipid peroxidation and an indicator of plant oxidative stress, suggesting that exogenous GABA can help alleviate low-temperature stress in tomato seedlings by maintaining membrane stability [63]. At the same time, the experiment also suggested that the supplementation of exogenous GABA reduced the accumulation of H2O2, thereby reducing lipid peroxidation in cold-stressed seedlings [64].
In extreme environments, excessive accumulation of reactive oxygen species (ROS) (such as superoxide anions, hydroxyl radicals, singlet oxygen, and hydrogen peroxide) can cause cellular damage, programmed cell death (PCD), and reduced plant productivity [65]. ROS are produced in various plant cell compartments, including the chloroplast, mitochondria, peroxisomes, and plasma membrane. Optimal levels of ROS are normally maintained by antioxidant defenses, so signals are transmitted to the nucleus through redox reactions, for example, using mitogen-activated protein kinase (MAPK) pathways in various cellular mechanisms to increase tolerance to various abiotic stresses [49,65]. Exogenous GABA application has been shown to increase the activity of catalase (CAT), superoxide dismutase (SOD), and ascorbate peroxidase (APX) enzymes, thereby reducing H2O2 accumulation in plants [66]. For example, hypoxia and respiratory inhibitors trigger the production of H2O2 in grape buds, while GABA can increase the H2O2 level by upregulating the expression of VvCAT2 or stimulating the TCA cycle, while upregulating the expression of VvAOX53 [67]. Similarly, it has been reported that exogenous application of GABA to barley seedlings also alleviates stress caused by Al3+ oxidative damage, increases antioxidant enzyme activity, and reduces ROS levels [68]. Concerning the physiological mechanism underlying hypoxia-induced increases in GABA content, research suggests that hypoxia stress initially reduces the availability of O2, impairing mitochondria ATP production. This can reduce the pumping activity of H+-ATPase, leading to membrane depolarization and activation of the GORK channel, resulting in K+ loss from the cytosol. Hypoxia stress also induces the accumulation of H2O2 in cells, activating ROS-inducible Ca2+ uptake channels and triggering a self-amplifying ROS-Ca2+ hub. This process leads to a rapid increase in H2O2 levels and the activation of K+-permeable NSCCs, causing a further decline in the cellular K+ pool. Ultimately resulting in the loss of cell vitality and programmed cell death (PCD). Hypoxia-induced increases in GABA levels can restore membrane potential (MP) through pH-dependent regulation of H+-ATPase and/or by activating the GABA shunt pathway and TCA cycle to generate more energy. Elevated GABA levels can also better regulate the ROS-Ca2+ center through the transcription control of RBOH gene, thereby preventing excessive H2O2 accumulation. Finally, GABA can function as a ligand to directly modulate the opening probability and conductance of the K+ efflux GORK channel [66].
GABA can serve both as a metabolite and a signaling molecule. When functioning as a metabolite, GABA is converted into succinate through the action of enzymes γ-aminotransferase and succinyl semialdehyde dehydrogenase (SSADH) [69]. Four T-DNA insertion mutants of SSADH (ssadh mutants) exhibit dwarf phenotypes, characterized by necrotic lesions, blanched leaves, reduced leaf area, lower chlorophyll content, shorter hypocotyls, and smaller flowers. GHB (γ-hydroxybutyrate, a by-product of SSA) has been reported to be five times higher in ssadh mutants than in wild-type Arabidopsis. Treatment with γ-vinyl-γ-aminobutyric acid, a specific inhibitor of GABA-T/POP2, or a mutation in the POP2 gene prevented ROS accumulation, inhibited cell death, and promoted growth in ssadh mutants [70].
Soil salinity, caused by elevated levels of sodium chloride (NaCl), significantly impacts crop yields worldwide [71,72]. In fact, salt stress is regarded as the most severe factor affecting crops yields, negatively influencing growth rate, strength and health. Saline conditions are particularly prevalent in irrigated areas, mainly due to the use of brackish irrigation. Globally, over 45 million hectares of irrigated land (about 20% of total irrigated areas) have been affected by saline conditions, with an estimated 1.5 million hectares being lost from cultivation annually [49,72]. Soil salinity causes plant salinity stress characterized by reduced photosynthesis and growth [73]. Impaired photosynthesis is partly due to the toxic effects of high salt concentrations on the photosynthetic machinery, which limits carbon assimilation and ultimately hinders plant growth [73]. To cope with salt stress, plants can employ different physiological strategies, such as ion exclusion, osmotic tolerance, and tissue tolerance [74]. These strategies are mediated by various respiration mechanisms, leading to a wide range of respiratory responses observed in salt-treated plants [75]. Ultimately, the nature of the respiration substrate will have a huge impact on the overall metabolic homeostasis of the plant, especially in regulating the balance between growth, compatible solute biosynthesis, and energy production.
Both glutamate dehydrogenase (GDH) and succinic semialdehyde dehydrogenase (SSADH) are a part of the gamma-aminobutyric acid (GABA) shunt pathway, providing alternative carbon sources for the tricarboxylic acid (TCA) cycle. Research has shown that GABA shunting plays a more significant role in respiratory metabolism under salt stress [76]. Increased GABA shunt activity provides an alternative carbon supply to the TCA cycle through succinate production, bypassing the mitochondrial pyruvate dehydrogenase complex (mtPDC) and mitochondrial 2-oxoglutarate dehydrogenase complex (mtOGDC) steps of the TCA cycle [77]. This alternate pathway can help plants maintain metabolic homeostasis and better adapt to salt stress conditions.
4.5 Carbon and Nitrogen Balance
The efficient assimilation of carbon (C) and nitrogen (N) is critical to optimal plant growth, productivity, and yield. GABA is considered central to carbon and nitrogen metabolism, with a strong functional link between the GABA shunt and the TCA cycle. Studies have shown that when the activities of succinyl-CoA ligase (SCOAL) in transgenic tomato and α-ketoglutarate dehydrogenase (AKGDH) in potatoes are inhibited, the TCA cycle activity decreases, but the GABA shunt activity increases to compensate for this decrease to a certain extent [78]. Another extent study found that GABP is a transporter that transports GABA in plants and exists on the mitochondrial membrane. When this protein is mutated, the content of GABA in mitochondria is reduced, and the activity of the tricarboxylic acid TCA cycle is significantly increased [28]. The balance and coordination between carbon metabolism and nitrogen metabolism is an important support for the normal growth and development of plants, and is achieved through a cyclic mechanism [79]. Exogenous GABA application has been demonstrated to increase nonstructural carbohydrates and TCA intermediates in poplar seedling stems under low nitrogen conditions. Additionally, GABA significantly attenuates the low-nitrogen-induced increase in leaf antioxidant enzymes, suggesting that GABA affects the C:N ratio of poplar growth by reducing energy costs under nitrogen-deficient conditions [80]. Understanding the role of GABA in carbon and nitrogen metabolism can contribute to the development of strategies for enhancing plant growth, especially under stress conditions, ultimately improving crop productivity.
Plants encounter various pathogens and pests during their growth and development. GABA levels in plants have been demonstrated to increase with biotic stress, playing a positive role in resisting pathogen invasion [79]. In the incompatible combination of Xanthomonas campestris (Xanthomonas campestris pv. vesicatoria, Xcv) and pepper (Capsicum annuum), the expression of arginine decarboxylase (ADC) was induced and GABA content was increased. Silencing CaADC1 in pepper leaves drastically compromised the accumulation of NO and H2O2, leading to enhanced avirulent Xcv growth during infection [81]. Applying exogenous GABA can inhibit the growth of pathogenic bacteria on ADC-silenced plants. It is known that ADC is responsible for catalyzing the conversion of arginine to agmatine and is the rate-limiting enzyme of putrescine synthesis pathway, which can be converted to GABA. Thus, ADC is suggested to regulate pepper’s resistance to Xanthomonas campestris by modulating GABA accumulation [81]. However, increased GABA content has not always been beneficial for plants in resisting pathogens. Studies have shown that R. solanacearum, the cause of bacterial wilt, secretes the effector protein RipI, which interacts with plant glutamate decarboxylase (GADs). GADs are activated and catalyze the biosynthesis of GABA by calmodulin γ-Biosynthesis of aminobutyric acid (GABA). R. solanacearum can effectively replicate with GABA as nutrient and ultimately lead to plant withering and death [82].
GABA accumulations also play a crucial role in plant resistance to insect feeding. When aphids (Heliothis virescens) climbed on tobacco leaves, leaf GABA rapidly increased five-fold, while rose-colored moth (Choristoneura rosaceana) larvae caused an increase in soybean leaf GABA content by eleven-fold [83]. In transgenic tobacco plants overexpressing the GAD gene of Petunia hybrida, the GABA content increases, and resistance to the root-knot nematode (Meloidogyne hapla) is 50% higher than that of wild-type tobacco plants [84]. These results suggest a positive correlation between GABA accumulation and plant resistance to insect feeding. The increase of GABA may disturb insect physiology by inhibiting the Cl-channels targeted by GABA in insect nerve, thereby inhibiting insect growth [9].
5 Conclusions and Future Perspectives
Since GABA was first discovered in potato tubers in 1949, it has gained increasing attention for its role in plant growth, development, and stress response. GABA accumulates in response to various biotic and abiotic stresses and has been shown to regulate adventitious root growth, primary root growth, and seed germination (as seen in Fig. 2). However, it is not yet clear how plants balance high GABA levels and growth under stress conditions, how plant hormones interact with GABA, and how GABA signaling operates in plants. Researchers need to identify the components that perceive GABA levels, the downstream components of GABA signal transduction pathways, and the interactions between GABA and other signaling molecules, such as nitric oxide (NO) and hydrogen (H2).
It has been reported that hydrogen-rich water (HRW) treatment may significantly improve the germination rate and growth rate of black barley (Hordeum distichum), as well as enriching its nutrients, phytochemicals, and secondary metabolites, such as GABA [85]. This perhaps indicates that H2 might have an interaction with GABA.
Future research directions for GABA in plants should focus on understanding its complex roles, signaling pathways, and interactions with other molecules. As GABA affects both abiotic and biotic stress responses, studying GABA in various plant species, including crop plants, is a valuable pursuit. Ongoing and future research in this area will likely provide answers to these questions and further our understanding of GABA’s roles in plants.
Funding Statement: This work was supported by Start-Up Funding from Shanghai University and the National Natural Science Foundation of China (Grant No. 31970289).
Author Contributions: The authors confirm contribution to the paper as follows: Xiangyang Hu designed the research and editing the manuscript; Yue Jin obtained the related references and wrote the manuscript; Lulu Zhi, Xin Tang and Yilin Chen also particulated into the writing process of the manuscript and drawed the figures together; John T Hancock edited the language expression. All authors reviewed the results and approved the final version of the manuscript.
Conflicts of Interest: The authors declare that they have no conflicts of interest to report regarding the present study.
References
1. Bouché, N., Fromm, H. (2004). GABA in plants: Just a metabolite? Trends in Plant Science, 9(3), 110–115. https://doi.org/10.1016/j.tplants.2004.01.006 [Google Scholar] [PubMed] [CrossRef]
2. Bowery, N., Enna, S. J., Olsen, R. W. (2004). Six decades of GABA. Biochemical Pharmacology, 68(8), 1477–1478. https://doi.org/10.1016/j.bcp.2004.07.033 [Google Scholar] [PubMed] [CrossRef]
3. Bown, A. W., Shelp, B. J. (2016). Plant GABA: Not just a metabolite. Trends in Plant Science, 21(10), 811–813. https://doi.org/10.1016/j.tplants.2016.08.001 [Google Scholar] [PubMed] [CrossRef]
4. Fromm, H. (2020). GABA signaling in plants: Targeting the missing pieces of the puzzle. Journal of Experimental Botany, 71(20), 6238–6245. https://doi.org/10.1093/jxb/eraa358 [Google Scholar] [PubMed] [CrossRef]
5. Zhou, H. L., Chen, H. Y., Bao, D. P., Tan, Y. S., Zhong, Y. J. et al. (2022). Recent advances of γ-aminobutyric acid: Physiological and immunity function, enrichment, and metabolic pathway. Frontiers in Nutrition, 9, 1076223. https://doi.org/10.3389/fnut.2022.1076223 [Google Scholar] [PubMed] [CrossRef]
6. Xu, B., Sai, N., Gilliham, M. (2021). The emerging role of GABA as a transport regulator and physiological signal. Plant Physiology and Biochemistry, 187(4), 2005–2016. https://doi.org/10.1093/plphys/kiab347 [Google Scholar] [PubMed] [CrossRef]
7. Ramesh, S. A., Tyerman, S. D., Gilliham, M., Xu, B. (2017). γ-aminobutyric acid (GABA) signalling in plants. Cellular and Molecular Life Sciences, 74(9), 1577–1603. https://doi.org/10.1007/s00018-016-2415-7 [Google Scholar] [PubMed] [CrossRef]
8. Liao, H. S., Chung, Y. H., Hsieh, M. H. (2022). Glutamate: A multifunctional amino acid in plants. Plant Sciences, 318, 111238. https://doi.org/10.1016/j.plantsci.2022.111238 [Google Scholar] [PubMed] [CrossRef]
9. Casida, J. E. (1993). Insecticide action at the GABA-gated chloride channel: Recognition, progress, and prospects. Archives of Insect Biochemistry and Physiology, 22(1–2), 13–23. https://doi.org/10.1002/(ISSN)1520-6327 [Google Scholar] [CrossRef]
10. Dreyer, I., Gomez-Porras, J. L., Riaño-Pachón, D. M., Hedrich, R., Geiger, D. (2012). Molecular evolution of slow and quick anion channels (SLACs and QUACs/ALMTs). Frontiers in Plant Science, 3, 263. https://doi.org/10.3389/fpls.2012.00263 [Google Scholar] [PubMed] [CrossRef]
11. Owens, D. F., Kriegstein, A. R. (2002). Is there more to GABA than synaptic inhibition? Nature Reviews Neuroscience, 3(9), 715–727. https://doi.org/10.1038/nrn919 [Google Scholar] [PubMed] [CrossRef]
12. Shelp, B. J., Mullen, R. T., Waller, J. C. (2012). Compartmentation of GABA metabolism raises intriguing questions. Trends in Plant Science, 17(2), 57–59. https://doi.org/10.1016/j.tplants.2011.12.006 [Google Scholar] [PubMed] [CrossRef]
13. Xu, B., Sai, N., Gilliham, M. (2021). The emerging role of GABA as a transport regulator and physiological signal. Plant Physiology, 187(4), 2005–2016. https://doi.org/10.1093/plphys/kiab347 [Google Scholar] [PubMed] [CrossRef]
14. Baum, G., Chen, Y., Arazi, T., Takatsuji, H., Fromm, H. (1993). A plant glutamate decarboxylase containing a calmodulin binding domain. Cloning, sequence, and functional analysis. Journal of Biological Chemsitry, 268(26), 19610–19617. [Google Scholar] [PubMed]
15. Ma, H. (2003). Plant reproduction: GABA gradient, guidance and growth. Current Biology, 13(21), R834–836. https://doi.org/10.1016/j.cub.2003.10.015 [Google Scholar] [PubMed] [CrossRef]
16. Long, Y., Tyerman, S. D., Gilliham, M. (2020). Cytosolic GABA inhibits anion transport by wheat ALMT1. New Phytologist, 225(2), 671–678. https://doi.org/10.1111/nph.16238 [Google Scholar] [PubMed] [CrossRef]
17. Ramesh, S. A., Kamran, M., Sullivan, W., Chirkova, L., Okamoto, M. et al. (2018). Aluminum-activated malate transporters can facilitate GABA transport. The Plant Cell, 30(5), 1147–1164. https://doi.org/10.1105/tpc.17.00864 [Google Scholar] [PubMed] [CrossRef]
18. Ramesh, S. A., Tyerman, S. D., Xu, B., Bose, J., Kaur, S. et al. (2015). GABA signalling modulates plant growth by directly regulating the activity of plant-specific anion transporters. Nature Communication, 6(1), 7879. https://doi.org/10.1038/ncomms8879 [Google Scholar] [PubMed] [CrossRef]
19. Yu, G. H., Zou, J., Feng, J., Peng, X. B., Wu, J. Y. et al. (2014). Exogenous γ-aminobutyric acid (GABA) affects pollen tube growth via modulating putative Ca2+-permeable membrane channels and is coupled to negative regulation on glutamate decarboxylase. Journal of Experimental Botany, 65(12), 3235–3248. https://doi.org/10.1093/jxb/eru171 [Google Scholar] [PubMed] [CrossRef]
20. Ji, J., Yue, J., Xie, T., Chen, W., Du, C. et al. (2018). Roles of γ-aminobutyric acid on salinity-responsive genes at transcriptomic level in poplar: Involving in abscisic acid and ethylene-signalling pathways. Planta, 248(3), 675–690. https://doi.org/10.1007/s00425-018-2915-9 [Google Scholar] [PubMed] [CrossRef]
21. Kaspal, M., Kanapaddalagamage, M. H., Ramesh, S. A. (2021). Emerging roles of γ aminobutyric Acid (GABA) gated channels in plant stress tolerance. Plants, 10(10), 2178. https://doi.org/10.3390/plants10102178 [Google Scholar] [PubMed] [CrossRef]
22. Belitsky, B. R. (2004). Bacillus subtilis GabR, a protein with DNA-binding and aminotransferase domains, is a PLP-dependent transcriptional regulator. Journal of Molecular Biology, 340(4), 655–664. https://doi.org/10.1016/j.jmb.2004.05.020 [Google Scholar] [PubMed] [CrossRef]
23. Baum, G., Lev-Yadun, S., Fridmann, Y., Arazi, T., Katsnelson, H. et al. (1996). Calmodulin binding to glutamate decarboxylase is required for regulation of glutamate and GABA metabolism and normal development in plants. EMBO Journal, 15(12), 2988–2996. https://doi.org/10.1002/j.1460-2075.1996.tb00662.x [Google Scholar] [CrossRef]
24. Fatma, M., Asgher, M., Iqbal, N., Rasheed, F., Sehar, Z. et al. (2022). Ethylene signaling under stressful environments: Analyzing collaborative knowledge. Plants, 11(17), 2211. https://doi.org/10.3390/plants11172211 [Google Scholar] [PubMed] [CrossRef]
25. Kathiresan, A., Tung, P., Chinnappa, C. C., Reid, D. M. (1997). Gamma-Aminobutyric acid stimulates ethylene biosynthesis in sunflower. Plant Physiology, 115(1), 129–135. https://doi.org/10.1104/pp.115.1.129 [Google Scholar] [PubMed] [CrossRef]
26. Palanivelu, R., Brass, L., Edlund, A. F., Preuss, D. (2003). Pollen tube growth and guidance is regulated by POP2, an Arabidopsis gene that controls GABA levels. Cell, 114(1), 47–59. https://doi.org/10.1016/S0092-8674(03)00479-3 [Google Scholar] [PubMed] [CrossRef]
27. Wilhelmi, L. K., Preuss, D. (1996). Self-sterility in Arabidopsis due to defective pollen tube guidance. Science, 274(5292), 1535–1537. https://doi.org/10.1126/science.274.5292.1535 [Google Scholar] [PubMed] [CrossRef]
28. Michaeli, S., Fait, A., Lagor, K., Nunes-Nesi, A., Grillich, N. et al. (2011). A mitochondrial GABA permease connects the GABA shunt and the TCA cycle, and is essential for normal carbon metabolism. Plant Journal, 67(3), 485–498. https://doi.org/10.1111/j.1365-313X.2011.04612.x [Google Scholar] [PubMed] [CrossRef]
29. Yue, J., Du, C., Ji, J., Xie, T., Chen, W. et al. (2018). Inhibition of α-ketoglutarate dehydrogenase activity affects adventitious root growth in poplar via changes in GABA shunt. Planta, 248(4), 963–979. https://doi.org/10.1007/s00425-018-2929-3 [Google Scholar] [PubMed] [CrossRef]
30. Pei, L., Zhao, Y., Shi, X., Chen, R., Yan, J. et al. (2022). The role of γ-aminobutyric acid (GABA) in the occurrence of adventitious roots and somatic embryos in woody plants. Plants, 11(24), 3512. https://doi.org/10.3390/plants11243512 [Google Scholar] [PubMed] [CrossRef]
31. Deleu, C., Faes, P., Niogret, M. F., Bouchereau, A. (2013). Effects of the inhibitor of the γ-aminobutyrate-transaminase, vinyl-γ-aminobutyrate, on development and nitrogen metabolism in Brassica napus seedlings. Plant Physiology and Biochemistry, 64(5), 60–69. https://doi.org/10.1016/j.plaphy.2012.12.007 [Google Scholar] [PubMed] [CrossRef]
32. Xie, T., Ji, J., Chen, W., Yue, J., Du, C. et al. (2020). GABA negatively regulates adventitious root development in poplar. Journal of Experimental Botany, 71(4), 1459–1474. https://doi.org/10.1093/jxb/erz520 [Google Scholar] [PubMed] [CrossRef]
33. Renault, H., El Amrani, A., Berger, A., Mouille, G., Soubigou-Taconnat, L. et al. (2013). γ-aminobutyric acid transaminase deficiency impairs central carbon metabolism and leads to cell wall defects during salt stress in Arabidopsis roots. Plant, Cell and Environment, 36(5), 1009–1018. https://doi.org/10.1111/pce.12033 [Google Scholar] [PubMed] [CrossRef]
34. Renault, H., El Amrani, A., Palanivelu, R., Updegraff, E. P., Yu, A. et al. (2011). GABA accumulation causes cell elongation defects and a decrease in expression of genes encoding secreted and cell wall-related proteins in Arabidopsis thaliana. Plant And Cell Physiology, 52(5), 894–908. https://doi.org/10.1093/pcp/pcr041 [Google Scholar] [PubMed] [CrossRef]
35. Renault, H., Roussel, V., El Amrani, A., Arzel, M., Renault, D. et al. (2010). The Arabidopsis pop2-1 mutant reveals the involvement of GABA transaminase in salt stress tolerance. BMC Plant Biology, 10(1), 20. https://doi.org/10.1186/1471-2229-10-20 [Google Scholar] [PubMed] [CrossRef]
36. Sebastiana, M., Gargallo-Garriga, A., Sardans, J., Pérez-Trujillo, M., Monteiro, F. et al. (2021). Metabolomics and transcriptomics to decipher molecular mechanisms underlying ectomycorrhizal root colonization of an oak tree. Scientific Reports, 11(1), 8576. https://doi.org/10.1038/s41598-021-87886-5 [Google Scholar] [PubMed] [CrossRef]
37. Busatto, N., Tadiello, A., Trainotti, L., Costa, F. (2017). Climacteric ripening of apple fruit is regulated by transcriptional circuits stimulated by cross-talks between ethylene and auxin. Plant Signaling & Behavior, 12(1), e1268312. https://doi.org/10.1080/15592324.2016.1268312 [Google Scholar] [PubMed] [CrossRef]
38. Cheng, P., Yue, Q., Zhang, Y., Zhao, S., Khan, A. et al. (2023). Application of γ-aminobutyric acid (GABA) improves fruit quality and rootstock drought tolerance in apple. Journal of Plant Physiology, 280(2), 153890. https://doi.org/10.1016/j.jplph.2022.153890 [Google Scholar] [PubMed] [CrossRef]
39. Yu, Y. B., Adams, D. O., Yang, S. F. (2022). Reprint of: 1-Aminocyclopropanecarboxylate synthase, a key enzyme in ethylene biosynthesis. Archives of Biochemistry and Biophysics, 726, 109238. https://doi.org/10.1016/j.abb.2022.109238 [Google Scholar] [PubMed] [CrossRef]
40. Hu, W., Yang, X. Y., Li, M. Y., Wang, Z., Xu, B. Y. (2009). Cloning and expression analysis of MaGAD1 gene from banana fruit. Acta Botanica Boreali-Occidentalia Sinica, 29, 429–434. [Google Scholar]
41. Ansari, M. I., Hasan, S., Jalil, S. U. (2014). Leaf senescence and GABA shunt. Bioinformation, 10(12), 734–736. https://doi.org/10.6026/97320630010734 [Google Scholar] [PubMed] [CrossRef]
42. Jalil, S. U., Ahmad, I., Ansari, M. I. (2017). Functional loss of GABA transaminase (GABA-T) expressed early leaf senescence under various stress conditions in Arabidopsis thaliana. Current Plant Biology, 9, 11–22. [Google Scholar]
43. Goel, P., Singh, A. K. (2015). Abiotic stresses downregulate key genes involved in nitrogen uptake and assimilation in Brassica juncea L. PLoS One, 10(11), e0143645. https://doi.org/10.1371/journal.pone.0143645 [Google Scholar] [PubMed] [CrossRef]
44. Ghosh, U. K., Islam, M. N., Siddiqui, M. N., Cao, X., Khan, M. A. R. (2022). Proline, a multifaceted signalling molecule in plant responses to abiotic stress: Understanding the physiological mechanisms. Plant Biology, 24(2), 227–239. https://doi.org/10.1111/plb.13363 [Google Scholar] [PubMed] [CrossRef]
45. Hayat, S., Hayat, Q., Alyemeni, M. N., Wani, A. S., Pichtel, J. et al. (2012). Role of proline under changing environments: A review. Plant Signaling & Behavior, 7(11), 1456–1466. https://doi.org/10.4161/psb.21949 [Google Scholar] [PubMed] [CrossRef]
46. Li, Z., Peng, Y., Huang, B. (2018). Alteration of transcripts of stress-protective genes and transcriptional factors by γ-aminobutyric acid (GABA) associated with improved heat and drought tolerance in creeping bentgrass (Agrostis stolonifera). International Journal of Molecular Sciences, 19(6), 1623. https://doi.org/10.3390/ijms19061623 [Google Scholar] [PubMed] [CrossRef]
47. Wang, D., Huang, H., Jiang, Y., Duan, X., Lin, X. et al. (2022). Exogenous phytosulfokine α (PSKα) alleviates chilling injury of banana by modulating metabolisms of nitric oxide, polyamine, proline, and γ-aminobutyric acid. Journal of Agricultural and Food Chemistry, 380(1–2), 132179. https://doi.org/10.1016/j.foodchem.2022.132179 [Google Scholar] [PubMed] [CrossRef]
48. Aghdam, M. S., Ebrahimi, A., Sheikh-Assadi, M. (2021). Phytosulfokine α (PSKα) delays senescence and reinforces SUMO1/SUMO E3 ligase SIZ1 signaling pathway in cut rose flowers (Rosa hybrida cv. Angelina). Scientific Reports, 11(1), 23227. https://doi.org/10.1038/s41598-021-02712-2 [Google Scholar] [PubMed] [CrossRef]
49. Yuan, D., Wu, X., Gong, B., Huo, R., Zhao, L. et al. (2023). GABA metabolism, transport and their roles and mechanisms in the regulation of abiotic stress (hypoxia, salt, drought) resistance in plants. Metabolites, 13(3), 347. https://doi.org/10.3390/metabo13030347 [Google Scholar] [PubMed] [CrossRef]
50. Balfagón, D., Gómez-Cadenas, A., Rambla, J. L., Granell, A., de Ollas, C. et al. (2022). γ-aminobutyric acid plays a key role in plant acclimation to a combination of high light and heat stress. Plant Physiology, 188(4), 2026–2038. https://doi.org/10.1093/plphys/kiac010 [Google Scholar] [PubMed] [CrossRef]
51. Aghdam, M. S., Flaherty, E. J., Shelp, B. J. (2022). γ-aminobutyrate improves the postharvest marketability of horticultural commodities: Advances and prospects. Frontiers in Plant Science, 13, 884572. https://doi.org/10.3389/fpls.2022.884572 [Google Scholar] [PubMed] [CrossRef]
52. Xu, B., Long, Y., Feng, X., Zhu, X., Sai, N. et al. (2021). GABA signalling modulates stomatal opening to enhance plant water use efficiency and drought resilience. Nature Communications, 12(1), 1952. https://doi.org/10.1038/s41467-021-21694-3 [Google Scholar] [PubMed] [CrossRef]
53. Blokhina, O., Virolainen, E., Fagerstedt, K. V. (2003). Antioxidants, oxidative damage and oxygen deprivation stress: A review. Annals of Botany, 91(2), 179–194. https://doi.org/10.1093/aob/mcf118 [Google Scholar] [PubMed] [CrossRef]
54. Bashir, R., Riaz, H. N., Anwar, S., Parveen, N., Khalilzadeh, R. et al. (2021). Morpho-physiological changes in carrots by foliar γ-aminobutyric acid under drought stress. Brazilian Journal of Botany, 44(1), 57–68. https://doi.org/10.1007/s40415-020-00676-7 [Google Scholar] [CrossRef]
55. Bailey-Serres, J., Voesenek, L. A. (2008). Flooding stress: Acclimations and genetic diversity. Annual Review of Plant Biology, 59(1), 313–339. https://doi.org/10.1146/annurev.arplant.59.032607.092752 [Google Scholar] [PubMed] [CrossRef]
56. Wang, C., Fan, L., Gao, H., Wu, X., Li, J. et al. (2014). Polyamine biosynthesis and degradation are modulated by exogenous gamma-aminobutyric acid in root-zone hypoxia-stressed melon roots. Plant Physiology and Biochemistry, 82, 17–26. https://doi.org/10.1016/j.plaphy.2014.04.018 [Google Scholar] [PubMed] [CrossRef]
57. Zhang, X., Lin, H. M., Hu, H., Hu, X., Hu, L. (2016). Gamma-aminobutyric acid mediates nicotine biosynthesis in tobacco under flooding stress. Plant Diversity, 38(1), 53–58. https://doi.org/10.1016/j.pld.2016.05.004 [Google Scholar] [PubMed] [CrossRef]
58. Breitkreuz, K. E., Allan, W. L., Van Cauwenberghe, O. R., Jakobs, C., Talibi, D. et al. (2003). A novel gamma-hydroxybutyrate dehydrogenase: Identification and expression of an Arabidopsis cDNA and potential role under oxygen deficiency. Journal of Biological Chemistry, 278(42), 41552–41556. https://doi.org/10.1074/jbc.M305717200 [Google Scholar] [PubMed] [CrossRef]
59. Xu, M., Yang, Q., Bai, G., Li, P., Yan, J. (2022). Polyamine pathways interconnect with GABA metabolic processes to mediate the low-temperature response in plants. Frontiers in Plant Science, 13, 1035414. https://doi.org/10.3389/fpls.2022.1035414 [Google Scholar] [PubMed] [CrossRef]
60. Kaur, R., Bains, T. S., Bindumadhava, H., Nayyar, H. (2015). Responses of mungbean (Vigna radiata L.) genotypes to heat stress: Effects on reproductive biology, leaf function and yield traits. Scientia Horticulturae, 197, 527–541. [Google Scholar]
61. Priya, M., Sharma, L., Kaur, R., Bindumadhava, H., Nair, R. M. et al. (2019). GABA (γ-aminobutyric acidas a thermo-protectant, to improve the reproductive function of heat-stressed mungbean plants. Scientific Reports, 9(1), 7788. https://doi.org/10.1038/s41598-019-44163-w [Google Scholar] [PubMed] [CrossRef]
62. Aghdam, M. S., Asghari, M., Farmani, B., Mohayeji, M., Moradbeygi, H. (2012). Impact of postharvest brassinosteroids treatment on PAL activity in tomato fruit in response to chilling stress. Scientia Horticulturae, 144, 116–120. https://doi.org/10.1016/j.scienta.2012.07.008 [Google Scholar] [CrossRef]
63. Malekzadeh, P., Khara, J., Heydari, R. (2014). Alleviating effects of exogenous Gamma-aminobutiric acid on tomato seedling under chilling stress. Physiology and Molecular Biology of Plants, 20(1), 133–137. https://doi.org/10.1007/s12298-013-0203-5 [Google Scholar] [PubMed] [CrossRef]
64. Ansari, M. I., Jalil, S. U., Ansari, S. A., Hasanuzzaman, M. (2021). GABA shunt: A key-player in mitigation of ROS during stress. Plant Growth Regulation, 94(2), 131–149. https://doi.org/10.1007/s10725-021-00710-y [Google Scholar] [CrossRef]
65. Hasanuzzaman, M., Bhuyan, M., Zulfiqar, F., Raza, A., Mohsin, S. M. et al. (2020). Reactive oxygen species and antioxidant defense in plants under abiotic stress: Revisiting the crucial role of a universal defense regulator. Antioxidants, 9(8), 681. https://doi.org/10.3390/antiox9080681 [Google Scholar] [PubMed] [CrossRef]
66. Wu, Q., Su, N., Huang, X., Cui, J., Shabala, L. et al. (2021). Hypoxia-induced increase in GABA content is essential for restoration of membrane potential and preventing ROS-induced disturbance to ion homeostasis. Plant Communications, 2(3), 100188. https://doi.org/10.1016/j.xplc.2021.100188 [Google Scholar] [PubMed] [CrossRef]
67. Vergara, R., Parada, F., Rubio, S., Pérez, F. J. (2012). Hypoxia induces H2O2 production and activates antioxidant defence system in grapevine buds through mediation of H2O2 and ethylene. Journal of Experimental Botany, 63(11), 4123–4131. https://doi.org/10.1093/jxb/ers094 [Google Scholar] [PubMed] [CrossRef]
68. Song, H., Xu, X., Wang, H., Wang, H., Tao, Y. (2010). Exogenous γ-aminobutyric acid alleviates oxidative damage caused by aluminium and proton stresses on barley seedlings. Journal of the Science of Food and Agriculture, 90(9), 1410–1416. https://doi.org/10.1002/jsfa.3951 [Google Scholar] [PubMed] [CrossRef]
69. Bouché, N., Fait, A., Bouchez, D., Møller, S. G., Fromm, H. (2003). Mitochondrial succinic-semialdehyde dehydrogenase of the γ-aminobutyrate shunt is required to restrict levels of reactive oxygen intermediates in plants. Proceedings of the National Academy of Sciences of the United States of America, 100(11), 6843–6848. https://doi.org/10.1073/pnas.1037532100 [Google Scholar] [PubMed] [CrossRef]
70. Fait, A., Yellin, A., Fromm, H. (2005). GABA shunt deficiencies and accumulation of reactive oxygen intermediates: Insight from Arabidopsis mutants. FEBS Letters, 579(2), 415–420. https://doi.org/10.1016/j.febslet.2004.12.004 [Google Scholar] [PubMed] [CrossRef]
71. Munns, R., Tester, M. (2008). Mechanisms of salinity tolerance. Annual Review of Plant Biology, 59(1), 651–681. https://doi.org/10.1146/annurev.arplant.59.032607.092911 [Google Scholar] [PubMed] [CrossRef]
72. Mushtaq, N. U., Saleem, S., Rasool, A., Shah, W. H., Hakeem, K. R. et al. (2021). Salt stress threshold in millets: Perspective on cultivation on marginal lands for biomass. Phyton-International Journal of Experimental Botany, 90(1), 51–64. https://doi.org/10.32604/phyton.2020.012163 [Google Scholar] [CrossRef]
73. Chen, T. W., Kahlen, K., Stützel, H. (2015). Disentangling the contributions of osmotic and ionic effects of salinity on stomatal, mesophyll, biochemical and light limitations to photosynthesis. Plant, Cell and Environment, 38(8), 1528–1542. https://doi.org/10.1111/pce.12504 [Google Scholar] [PubMed] [CrossRef]
74. Tyerman, S. D., Munns, R., Fricke, W., Arsova, B., Barkla, B. J. et al. (2019). Energy costs of salinity tolerance in crop plants. New Phytologist, 221(1), 25–29. https://doi.org/10.1111/nph.15555 [Google Scholar] [PubMed] [CrossRef]
75. Jacoby, R. P., Taylor, N. L., Millar, A. H. (2011). The role of mitochondrial respiration in salinity tolerance. Trends in Plant Science, 16(11), 614–623. https://doi.org/10.1016/j.tplants.2011.08.002 [Google Scholar] [PubMed] [CrossRef]
76. Feng, D., Gao, Q., Sun, X., Ning, S., Qi, N. et al. (2023). Effects of foliage-applied exogenous γ-aminobutyric acid on seedling growth of two rice varieties under salt stress. PLoS One, 18(2), e0281846. https://doi.org/10.1371/journal.pone.0281846 [Google Scholar] [PubMed] [CrossRef]
77. Che-Othman, M. H., Jacoby, R. P., Millar, A. H., Taylor, N. L. (2020). Wheat mitochondrial respiration shifts from the tricarboxylic acid cycle to the GABA shunt under salt stress. New Phytologist, 225(3), 1166–1180. https://doi.org/10.1111/nph.15713 [Google Scholar] [PubMed] [CrossRef]
78. Studart-Guimarães, C., Fait, A., Nunes-Nesi, A., Carrari, F., Usadel, B. et al. (2007). Reduced expression of succinyl-coenzyme A ligase can be compensated for by up-regulation of the γ-aminobutyrate shunt in illuminated tomato leaves. Plant Physiology, 145(3), 626–639. https://doi.org/10.1104/pp.107.103101 [Google Scholar] [PubMed] [CrossRef]
79. Seifikalhor, M., Aliniaeifard, S., Hassani, B., Niknam, V., Lastochkina, O. (2019). Diverse role of γ-aminobutyric acid in dynamic plant cell responses. Plant Cell Reports, 38(8), 847–867. https://doi.org/10.1007/s00299-019-02396-z [Google Scholar] [PubMed] [CrossRef]
80. Chen, W., Meng, C., Ji, J., Li, M. H., Zhang, X. et al. (2020). Exogenous GABA promotes adaptation and growth by altering the carbon and nitrogen metabolic flux in poplar seedlings under low nitrogen conditions. Tree Physiology, 40(12), 1744–1761. https://doi.org/10.1093/treephys/tpaa101 [Google Scholar] [PubMed] [CrossRef]
81. Kim, N. H., Kim, B. S., Hwang, B. K. (2013). Pepper arginine decarboxylase is required for polyamine and γ-aminobutyric acid signaling in cell death and defense response. Plant Physiology, 162(4), 2067–2083. https://doi.org/10.1104/pp.113.217372 [Google Scholar] [PubMed] [CrossRef]
82. Xian, L., Yu, G., Wei, Y., Rufian, J. S., Li, Y. et al. (2020). A bacterial effector protein hijacks plant metabolism to support pathogen nutrition. Cell Host & Microbe, 28(4), 548–557.e7. https://doi.org/10.1016/j.chom.2020.07.003 [Google Scholar] [PubMed] [CrossRef]
83. Bown, A. W., Hall, D. E., MacGregor, K. B. (2002). Insect footsteps on leaves stimulate the accumulation of 4-aminobutyrate and can be visualized through increased chlorophyll fluorescence and superoxide production. Plant Physiology, 129(4), 1430–1434. https://doi.org/10.1104/pp.006114 [Google Scholar] [PubMed] [CrossRef]
84. McLean, M. D., Yevtushenko, D. P., Deschene, A., Cauwenberghe, O. R. V., Makhmoudova, A. et al. (2003). Overexpression of glutamate decarboxylase in transgenic tobacco plants confers resistance to the northern root-knot nematode. Molecular Breeding, 11(4), 277–285. https://doi.org/10.1023/A:1023483106582 [Google Scholar] [CrossRef]
85. Guan, Q., Ding, X. W., Jiang, R., Ouyang, P. L., Gui, J. et al. (2019). Effects of hydrogen-rich water on the nutrient composition and antioxidative characteristics of sprouted black barley. Journal of Agricultural and Food Chemistry, 299(3), 125095. https://doi.org/10.1016/j.foodchem.2019.125095 [Google Scholar] [PubMed] [CrossRef]
Cite This Article
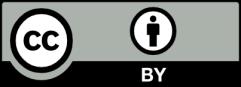