Open Access
ARTICLE
Selenium: A Game Changer in Plant Development, Growth, and Stress Tolerance, via the Modulation in Gene Expression and Secondary Metabolite Biosynthesis
1
Department of Plant Breeding and Biotechnology, Faculty of Agriculture, University of Tabriz, Tabriz, 51368, Iran
2
Department of Biotechnology, Faculty of Agriculture, Azarbaijan Shahid Madani University, Tabriz, 51368, Iran
3
Department of Genetics and Plant Breeding, Bangladesh Agricultural University, Mymensingh, 2202, Bangladesh
* Corresponding Authors: Ali Bandehagh. Email: ; Mohammad Anwar Hossain. Email:
(This article belongs to the Special Issue: Selenium, Silicon and their Nanoparticles-mediated Environmental Stress Tolerance in Crop Plants)
Phyton-International Journal of Experimental Botany 2023, 92(8), 2301-2324. https://doi.org/10.32604/phyton.2023.028586
Received 27 December 2022; Accepted 06 April 2023; Issue published 25 June 2023
Abstract
The presence of selenium (Se) is not widely established as crucial for crops, although it is commonly recognized as an important nutrient for animals as well as humans. Even so, it is inevitably accepted that Se usually contributes positively to the life cycle of plants. Previous findings suggested that small amounts of Se seem to have a productive role in growth and production. As a result, Se is assumed to function in multiple ways, primarily by influencing a variety of biochemical and physiological functions. Also, Se also acts as a plant antioxidant and pro-oxidant and confers tolerance against different abiotic stresses, including salinity, drought, extreme temperature, and toxic metals/metalloids stresses. It reflects a defensive barrier against stress by increasing chlorophyll content synthesis, photosynthesis, oxygen supply, osmoprotectant concentration, and secondary metabolite acquisition. One other crucial role of Se is its ability to strengthen antioxidant performance in plants, thereby decreasing the concentration of reactive-oxygen-species (ROS). Furthermore, Se generates and modifies genes and proteins that respond situationally to stress, and the presence of high Se concentrations in the growth-medium can cause phytotoxic conditions via excessive ROS production, and through pro-oxidative Se occurrence, suppression of chlorophyll contents in the biosynthetic pathway, and the inhibition of plant developmental and normal physiological functions. Like a phytofortifier, the correct amount of Se can indeed enhance the nutrient quality of both crop and fodder production. Furthermore, crops have naturally developed ways to combat Se-deficiency and Se-toxicity. The current review focuses on recent advances in understanding the dynamics of Se, the positive and negative roles of Se in crop management, and its efficiency in countering abiotic stress.Keywords
Selenium (Se) is a toxic metalloid and a rare element. The Earth’s crust, a variety of ecosystems, aquatic systems, and soil profiles all contain significant levels of Se, but in varying proportions, depending on geographical characteristics [1–3]. One of the elements included in the category of micronutrients that are required in low contents for organisms to operate properly is Se. Selenium aids in the detoxification of heavy metals, the control of the immune and reproductive systems, and the defense of cells against excessive H2O2. Selenium causes selenoprotein production, which is important in the organism’s antioxidant defense system. Selenium is a major component of mammalian protein, often called selenoproteins [4]. There are 25 selenoproteins widely recognized and classified. Twelve of them are involved in redox homeostasis and are important for antioxidant capacity. Antioxidant selenoenzymes are the remaining proteins [5]. Glutathione peroxidase (GPX) and thioredoxin reductases (TrxRs) are essential selenoproteins. Selenoproteins form anti-oxidant barriers to defend the organism from harmful impacts such as ROS [6]. TrxRs are proteins that are in charge of cellular redox situation maintenance, prediction, and signaling [7]. Selenoprotein P (SePP) is an antioxidant present in blood plasma, and its activity is dependent on the amount of selenium in the animal tissue or plant cell [8]. Selenium has been shown to have anticarcinogenic properties [9]. In crops, the form of Se is either inorganic or organic [10]. Se is exclusively found in a few minerals and is rarely found in its basic form in nature [3]. It has been shown that Se is important for humans, animals, and some microbes. While its essentiality for plants is still debatable and unproven, it is nevertheless recognized as a positive component. Se can function optimally in countering the adverse impacts of abiotic stress and could enhance plant development and growth once applied at low amounts [11] and its useful role in low doses has indeed been thoroughly researched. It has reportedly benefited crop development by enhancing antioxidant enzyme activity [12] while making plants more resistant to multiple forms of abiotic stress, such as drought [13], UV-induced stress [14], cold [15], salinity [16], and metals/metalloids [17]. In addition, it potentially increases agricultural output, thereby demonstrating its significance in plant development and growth [18]. Similarly, Se has been successfully offered to plants in many situations and assimilated by them as an effective solution to the problem of Se-deficiency [19]. Also, Se enhances photosynthesis, promotes ion homeostasis, and initiates a series of downstream processes that aid in stress reduction. It promotes adaptability in the face of salt stress. Knowing the effect of Se, such as Se take-up, transport, and movement, and how it impacts various plant operations, would provide another opportunity to improve plant salt tolerance [20]. Much plant research on the defensive impacts of Se during drought stress suggested that the impacts of Se are related to its capacity to control the water condition of plants in a water shortage. According to Aissa et al. [21], adding 0.1 or 0.25 mM Se induced a 2%–6% rise in leaf water content, enhancing drought tolerance. At the optimal rate of water supply, the effect of Se on the amount of intracellular water was likewise less noticeable. In Fagopyrum esculentum, Tadina et al. [22] discovered that plants under water stress had considerably reduced the stomatal conductance (gs), but Se supplementation greatly enhanced gs. Selenium boosted PSII performance, which was ascribed to betterment in plant water status. In the study conducted by Sieprawska et al. [23], the rise in the content of anthocyanin, sugars, and proline in the application of Se in adequate dosages may suggest the interference of non-enzymatic substances synthesis [23]. The PSII system also showed a rise in chlorophyll concentration and photosynthetic quantum performance activities [24].
Furthermore, various forms of Se can be applied as soil amendments, e.g., selenate [SeO42−] or selenite [SeO32−]. According to the latest findings on tomatoes, using low Se concentrations improved antioxidative properties, including superoxide dismutase (SOD), GPX, and catalase (CAT) [25], glutathione reductase (GR), ascorbate peroxidase (APX), dehydroascorbate reductase (DHAR), SOD activity, and monodehydroascorbate reductase (MDHAR) in maize [26], as well as GPX and GR activities in wheat [27]. As a result of the Se supplementation, plant oxidative stress was decreased. Excessive Se, on the other hand, causes toxicity in crops, which ultimately results in chlorosis and necrosis, in addition to limited growth and decreased protein biosynthetic pathways [28]. So, more research is needed to see whether Se is essential for plant development and growth. Plants, on the other hand, may engage, accumulate, and adapt to Se, As a result, this review focuses on the potential beneficial or harmful impacts of Se on plant growth and development, and also Se absorption, transport, and metabolism. Also, the dynamics of Se absorption, allocation, and metabolic activity are discussed analytically to arrive at a comprehensive standpoint on the importance, function, and interactivity of Se.
2 Multidimensional Functionality of Se, Affecting Plant Development, Growth, and Stress Tolerance
By the beginning of this century, Se was already found to have advantageous functions in plants that can accumulate huge amounts of this element [19]. In many contexts, Se works in a dose-dependent manner [29]. Fig. 1 depicts some of Se’s positive and negative impacts, although the available literature lacks complete information on the exact necessity of Se for plant metabolism and cellular maintenance [11]. Fayram [30] identified three levels of biological action by Se: (i) detection of Se concentration needed for growth and development; (ii) adequate density that may be saved to preserve homeostatic operations; and (iii) toxic concentrations. In lettuce plants (Lactuca sativa) and ryegrass (Lolium perenne), Se exerted beneficial effects at low concentrations (0.1 mg kg−1), whereas higher levels (>10 mg kg−1 and 1.0 mg kg−1) led to toxicity in lettuce and ryegrass, respectively [31]. Regarding the antioxidative properties of Se which counterbalance oxidative stress, plants have been observed to grow healthily by the effect of Se at low doses [21]. Seed germination is also another process that benefits from supplementary Se. According to Puccinelli et al. [32], excessive Se concentrations (>29 mg kg−1 soil) had adverse effects on the seed germination of (Raphanus sativus) radishes and (Solanum lycopersicum) tomatoes. In contrast, using SeO32− as a seed priming agent increased the growth of Momordica charantia seedlings. Lapaz et al. [33] provided evidence that adding Se to the nutrient solution enhanced carbohydrate concentration in early-age leaves and stolons of potato plants, but that was not linked to a higher photoassimilate yield because the supplementary Se did not change the net photosynthesis [34]. Following Se supplementation, Hasanuzzaman et al. [35] reported substantial increases in the expansion and synthesizing of carotenoids and chlorophyll activity (chl a, b) in ornamental gerberas (5–20 mg L−1).
Figure 1: Selenium can bring advantages or disadvantages to plants, depending on the dose of supplementation. Low doses usually benefit plants, whereas high doses tend to have the opposite effect. The negative effects often result from an excessive presence of Se in the nutrient solution or the soil. Nonetheless, exact thresholds of advantageous/disadvantageous concentrations largely remain undiscovered in the case of many plant species
Se could indeed boost agricultural output by having a significant influence on cellular metabolism [18]. Similarly, the problem of Se-deficiency can be addressed in many plants by adequate supplements of Se [19]. Furthermore, several forms of Se have the capacity to serve as efficient fertilizers (e.g., selenite [SeO32−] and selenate [SeO42−]). In response to Se supplementation, plants reportedly developed lower levels of oxidative stress. Excessive Se, on the other hand, causes toxicity in crops, which ultimately results in chlorosis and necrosis, in addition to constrained development and weakened protein biosynthetic pathways [28]. Several other research findings have demonstrated that Se, at low doses, promotes crop production (Table 1). In a relevant study, it was observed that treating chili pepper plants with 5 μM Se caused the architectural volume of roots to expand, compared to the control treatment, and, also, the relative-water-content (RWC) increased by 13% [36]. Likewise, the application of Se (3 and 5 μM) increased the leaf area by 25%, resulting in greater plant growth and biomass. According to Chauhan et al. [17], rice crops improved in terms of production and growth, as they benefited from small doses of Se.
There are indications that Se has anti-senescence properties, assisting with the maintenance of cell components and actions, thereby improving plant longevity [37]. The inclusion of Se in nutrient solutions can promote plant development and growth. Research by Wang et al. [38] and Hemmati et al. [18] showed that optimizing the availability of Se in arable lands seems to be a feasible and effective way to enhance production. Lettuce seedlings in trays grew more vigorously in response to different doses of Se (two concentrations of 0, 30 mg.dm−3), compared to the control group [2]. Furthermore, positive impacts of Se were observed in the absorption and utilization of essential nutritional components, even in the case of crops grown on different forms of media [39].
2.1 Benefits of Se for Plant Tolerance to Stress
Advancements in agriculture are frequently held back by the prevalence of abiotic stresses. Thus, scientists are continuously attempting to create modern techniques to combat the limits caused. Exogenous protectants are becoming more common for imparting stress resistance (Table 2). Selenium could indeed reduce the likelihood of membrane destruction by enhancing the photosynthetic machinery, increasing the direct quenching of ROS, and boosting enzymatic/non-enzymatic systems that promote plant-based defense through antioxidants (Table 2) [17]. Since Se can perform antioxidant roles in plants, while enhancing crop yield, it is capable of making plants tolerant to several types of abiotic stress. Through a chain of reactions and interactions, Se induces an increase in antioxidant activity and the defense system which strive to maintain plant physiological parameters in their normal state, resulting in a positive impact on crop yield. Several aspects of action by Se, however, remain unknown. Se causes cell death by increasing lipid peroxidation, α-tocopherol concentrations, and, in particular, GPX activity. Thus, systemic defense is strengthened to assist plants in mitigating the effects of ROS and reducing the amount of damage they cause (Fig. 2) [11]. Haghighi et al. [44] investigated Se-absorption and antioxidant-defense reactions in rice, discovering that a small amount of Se (5 mg kg−1) increased the yield and growth of rice by contributing to the functions of glutathione (GSH), ascorbate (AsA), Peroxidase (POD), CAT, and SOD [52]. Under cadmium stress, adding Se (1 M SeO42−) to the nutrient solution of tomatoes enhanced their functions and antioxidative-defense enzymatics, such as APX (26%) and CAT (21%), thereby improving the ability of tomatoes to tolerate cadmium stress. The GSH biosynthetic pathway was also advanced by Se treatment (33%) [50]. In this regard, treating tomatoes with elemental Se (40 M) resulted in enhanced tolerance to drought and increased activities of SOD (56%), APX (44%), and CAT (27%) [53]. Tolerant plants, treated with Se, reportedly synthesized more antioxidants of the non-enzymatic type, such as α-tocopherol (36%), GSH (107%), and AsA (up to 131%).
Figure 2: A schematic representation of how Se contributes to the defensive line of antioxidants against abiotic stress. Optimum doses of Se can enhance plant yield, growth, and health by assisting in ROS-scavenging and antioxidant activity, as evidenced by a dotted box illustrated above “metabolic adaptation”. On the other side of the spectrum, plants can grow less and produce smaller amounts of yield when Se is applied in high doses, resulting in less ROS-scavenging and a weakened antioxidant defense, as demonstrated by the dotted box on the right and the arrows that face downwards. In sum, low doses of Se have a protective role in the integrity of plants, whereas high doses are usually detrimental to plant health
2.2 Benefits of Selenium for Plant Tolerance to Salinity
Salinity has an impact on agricultural output by impeding plant development and growth. The available literature contains many case studies on the significance of Se in mitigating the adverse effects of salt stress on plants (Table 2). However, there is a lack of sufficient information on how Se mediates the balancing mechanism of plant tolerance to excessive salt.
2.3 Benefits of Selenium for Plant Tolerance to Drought and Extreme Temperatures
Numerous tests have been performed to investigate the effectiveness of Se in giving plants the ability to tolerate dry conditions (Table 2). Hasanuzzaman et al. [58] treated rapeseed seedlings with Se (25 μM; Na2SeO4) as they were exposed to different drought intensities (10% or 20% PEG). Drought stress had no significant effect on GSH, GSSG, H2O2, or MDA accumulation in stressed plants that were treated with Se. Even so, at 10% PEG, there was a rise in the AsA concentration, as well as in the MDHAR and GR activities. Curiously, there were increases in glutathione S-transferase (GST), GPX, DHAR, and Gly I activities under both levels of drought stress, whereas the activities of CAT and Gly II declined [58]. A study on olive plants showed that foliar spraying with Na2SeO4 (50 mg or 150 mg Se L−1) increased plant productivity and photosynthetic rates while retaining the RWC at a high level [60]. Particularly, Se promoted the activity of APX, GPX, and CAT, thereby preventing the peroxidation of lipids.
Selenium can inhibit ROS production, increase cellular viability, boost antioxidant activity, and uphold reproductive integrity in vitro and in vivo under extreme temperatures (Table 2). Malerba et al. [73] discovered that Se significantly improved cellular viability by partly preventing the cytoplasm from shrinking, reducing the O2• output, and ameliorating the effects of heat shock (50°C, 5 min) by controlling the peroxidation of lipids in tobacco cell cultures. Since Se was observed to be involved in defending oilseed rape seedlings from oxidative stress, Hasanuzzaman et al. [11] showed that high temperatures (HT) at 38°C impacted the molecular participants of GSH-AsA biosynthesis, resulting in increased ROS generation [11]. Seedlings supplemented with 25 μM Na2SeO4 had more enzymatic activities and managed to accumulate more AsA and GSH. As a result, small supplements of Se caused greater tolerance to oxidative-stress caused by intermittent HT. In small amounts, Se (2 mg L−1 Na2SeO4) protected wheat from the damages of HT (38°C). As a spray treatment, Se increased crop growth, photosynthetic pigments, and the total amount of phenols [63]. The growth of cucumber seedlings, their photosynthetic activity and chlorophyll formation were suppressed by HT (40°C/30°C day/night), while the damage caused by oxidative stress became more prominent. However, the use of 8 μM Se at the flowering-initiation phase demonstrated higher photosynthetic rates, more osmolytes, and a greater level of antioxidant activity, thereby defending the crops against oxidative stress and bringing the possibility of optimal yields, compared to the case of untreated crops. Thus, the fruit weight and the total number of fruits per plant increased by 43% and 35%, respectively, in Se-treated crops, especially in comparison with HT-treated crops without the Se treatment [64].
Selenium partially helps protect crops from the effects of low temperatures (LT). A dose-dependent application of Se assisted cucumbers in becoming less prone to chilling-injury [74]. When exposed to LT, Se-treated cucumber plants showed resistance to the kind of oxidative stress that resulted from LT. Iqbal et al. [75] discovered that Se largely exerts its effects depending on the dose of the treatment, with a negative effect at larger doses. Responses of wheat seedlings to exogenous selenium supply under cold stress. They discovered that applying Se at 0.5 or 1.0 mg kg−1 enhanced chlorophyll content and seedling biomass, whereas its dose at 2.0 and 3.0 mg kg−1 enhanced the amount of chlorophyll only. Furthermore, the effectiveness of antioxidant activity varied in response to high or low levels of Se application, although it was reported that the 1.0 and 2.0 mg kg−1 dose was partly successful in mitigating the effects of LT on lipid peroxidation. Huang et al. [15] discovered that using Se treatments as a foliar spray reduced the adverse impact of LT on strawberries. In a time-dependent manner, while photosynthetic parameters were rendered suboptimal because of chilling-stress, and as the antioxidant activity of enzymes decreased, the role of supplementary Se (5 mg L−1) improved the condition of LT-affected plants, compared to the control group.
2.4 Benefits of Selenium for Plant Tolerance to Toxic Metals/Metalloids
The physiological performance of various plants reportedly increased in response to Se application, as they demonstrated enhanced levels of resistance to toxic levels of metals and metalloids (Table 2). Se has reportedly protected plants by causing the roots to reduce their absorption of metalloids and metals that may become toxic, limiting the extent to which they are mobile in reaching aerial organs of plants [39]. When Se is added to heavy metal-containing media, it may come into contact with the subcellular distribution and chemical variants of heavy metals that enter the roots and with the elements in the cellular walls of roots [76]. Surprisingly, Se creates an encouraging scenario for Fe absorption, invigorating the roots to absorb more of it, and, thus, providing more for the requirements of photosynthesis and chloroplast-driven processes [39]. Furthermore, when plants gain access to supplementary Se, they become more capable of synthesizing phytochelatin (PC) and compounds that can counter toxicity. Se can bind to other metals and form complex molecular structures that aid in the reduction of toxic symptoms caused by unwanted metals/metalloids [66]. In a relevant study, Se (2 μM) reduced the toxic effects of cadmium in oilseed rape by regulating, CAT, SOD, GPX, and APX activities and limiting the peroxidation of lipids [68]. In a report it was shown that Se had a significant role in reducing MDA-based substances, while enhancing the activities of MDHAR, APX, GR, DHAR, CAT, and GPX in plants, increasing the amounts of GSH and AsA, and leveling up the GSH/GSSG ratio in Cd-stressed oilseed rape [11]. Se contributes to the suppression of cadmium translocation, making it difficult for this element to reach the aerial parts of plants and hampering its bioavailability to plant cells [66].
Applying exogenous SeO32− (5, 10, and 15 mg kg−1) significantly reduced the accumulation of lead- and cadmium-based compounds in rapeseed, and, ultimately, hampered the mobility of these compounds in the xylem [77]. Furthermore, SeO32− reduced the amount of oxidative damage caused by cadmium and lead, through the discouragement of ROS production and lipid peroxidation. In faba beans, lead-induced stress was countered by small supplementary quantities of SeO32− (1.5 or 6 μM) [46].
According to Cartes et al. [42], SeO32− has been effective in neutralizing the toxicity of aluminum (Al) in ryegrass roots. It was discovered that Se contributed to the increase in POD activity, along with greater O2•− dismutations into H2O2, but it significantly reduced the output of thio-barbituric acid-reactive substances (TBARS). SeO32− functioned as an efficient neutralizer of toxic metals by promoting the synthesis of GSH and thiols, while decreasing the peroxidation of lipids [42].
3 Se-Mediated Regulation of Gene Expression
As genes are regulated by transcription factors, they mediate adjustments and adaptability to various environmental variables. Throughout this context, plant cells respond to both external and internal signals via orchestrated signal perception and transduction systems that involve a variety of elements, the most significant of which include effectors, plant hormones, ionic transporters, Ca+2, and kinases [25]. A transcriptional protein could indeed partly regulate transcriptions of downstream-genes, reconfiguring the growth of plants and biochemical reactions [25]. As a result, transcription factors make excellent target genes for orchestrating vital processes. The WRKY family of proteins both indirectly or directly participates in the down- or up-regulation of plant physiology and targets genes that are tasked with molecular defense at times of stress [26,27]. The transcription factor WRKY1 is responsible for the regulation of formative and stress-induced responses from gene clusters [25,28]. Among transcription factors, those of 4CL and PAL regulate catalytic reactions and synthesize important proteins when producing significant amounts of secondary-metabolites (SMs). Moreover, salicylic acid is a significant signaling agent generated by PAL’s catalytic action [29]. Among the critical methods required for the control of gene expression is the regulatory oversight of the evolving environment of chromatin.
Throughout the gene-protein relationship, DNA fragments are methylatedas a significant involvement in mediated functions, aiding in the reconfiguration of hetero-chromatin to euchromatin and translating them to a variety of molecular signals. High biological activities in plant extracts have indeed been credited to sophistication in the phytochemistry of plant cells that produce a plethora of valuable metabolic pathways for terpenoids and phenolic substances [30]. Using Se as a supplement reportedly resulted in linear upregulations of the WRKY1 gene. Similarly, the Se treatment significantly increased the transcription of 4CL and PAL gene clusters, as implicated in SMs production and charging the output of crucial SMs in plant defense, particularly salicylic acid which signals multiple reactions in the plant cell. The main component of signaling pathways and events is a WRKY1 transcription factor, which is preceded more by regulatory oversight of downstream-defending genes assigned to disease prevention [13,25]. In this regard, WRKY1 has transcriptional elements that support pathways signaled by salicylic acid [41]. An overexpression of WRKY1 in grapevine tissues was reportedly attributed to upregulations of a gene family encoding jasmonic-acid and, as a result, an improvement in stress tolerance [41]. This gene also played a role in secondary metabolism (SM) and metabolic control [25]. One research, in particular, showed that using Se supplements ultimately induced a transcription factor aimed at bZIP and WRKY1 synthesis in peppers [10]. Similarly, exposing plant cells to Se was associated with a shift in the transcriptional template of the heat-shock factor [12]. In addition, while responding to the Se treatment, upregulations occurred in rosmarinic acid synthase (RAS) and hydroxyphenyl pyruvate reductase (HPPR) in lemon balm [78]. Numerous studies have identified Se-associated modifications in endogenous proteins as a critical contributing factor [3,10,15,42,78]. Low amounts of Se-induced nitrate reductase activity are substantial indicators of Se-induced modifications in metabolic activities, whereas high doses of Se disturbed nitrate-reductase activity, leading to proline absorption. In one study, Se was linked to changes in the functionality of nitrate-reductase [10,12,43,78]. The presence of proline was dynamically increased by Se supplements when used as sprays on lettuce foliage [10,15]. It leveled up nitrogen metabolic activity in lettuce by enhancing the functions of nitrate-reductase and the biosynthetic pathway of glutamate [43]. Changes in NO levels caused by Se have been linked to the functions of nitrate-reductase [44]. Se consumption altered the density-enhancing actions of catalase and peroxidase for the more efficient performance of antioxidants. The initiation of the antioxidant defense system by Se can be highlighted as a crucial system that contributes to more efficient safeguarding of crops against abiotic stressors [2,13,42]. Different forms of Se supplements have reportedly functioned as elicitors that facilitate higher levels of SM, as evidenced by an increase in the action of PAL enzymatic activity to synthesize phenylpropanoids, while being associated with an increase in the output of gene clusters that encode PAL and 4CL [2,10,12,78].
3.1 Regulation of Photosynthesis-Related Genes by Se
The most fundamental biological procedure in crops is photosynthesis. Se is capable of boosting antioxidant activity through enzymatic pathways, thereby helping plants defend themselves from stressful conditions. Enzymes of antioxidant capacity include, but are not limited to, peroxidase, catalase, glutathione peroxidase, superoxide dismutase, and ascorbate peroxidase [79–81]. Selenium-based compounds have been shown to stimulate biomass production and boost photosynthesis in lettuce and sorrel plants [82]. Of this kind, selenium-based compounds benefited the physiological aspects of barley and sorghum [61]. The normality of photosystem attributes was reportedly maintained by the application of selenium, protecting plants from the adverse effects of UV-B radiation and cadmium [83]. Selenium influenced the expression of photosynthesis-related genes. In potato leaves, selenite made a transient repression of genes encoding light-receptive compounds involved in photosynthesis [84]. From a perspective of proteomics, selenium treatments had a significant impact on rice crops, their primary metabolism, photosynthesis, and redox homeostasis. Selenium deficiency activated the anti-oxidative system, enhancing photosynthesis and primary metabolic activity. Applying high Se concentrations, on the other hand, harmed the photosynthetic apparatus, inhibited photosynthesis, and suppressed primary metabolic activity. Variations exist among the responsiveness of different genes to selenium [85]. Relevant research indicated that spraying amino acid, chelated-selenium on pears, grapes, and peaches increased their photosynthetic output. Precisely, the efficiency of PSII was enhanced by the application of supplementary Se which, in turn, promoted photochemical quenching [84].
Selenium-induced dysfunctions are likely to have adverse impacts on photosynthesis, suggesting preliminary causes of excessive ROS buildup and oxidative pressure [39]. However, regarding the anti-phytotoxic roles of Se, Meeta et al. [86] discovered that 0.1–0.5 μM of SeO32− reduced cellular levels of aminolevulinic acid (ALA) in maize foliage [86]. Relevantly, a decrease in chlorophyll content was significantly notable compared to the decrease in carotenoid compounds and ALA. Nonetheless, providing plants with more SeO32− resulted in a reduction of ALA buildup in the presence and absence of light. Based on the available data on growth inhibition, chlorophyll decreased significantly in cucumber plants that faced Se-deficiency [87]. As a result, a decline in photosynthetic pigment concentration levels is viewed as a more prevalent response to the phytotoxic effects of high-dose Se, whereas leaf area and FW seem to be affected less intensely. Zhang et al. [88] demonstrated in an experiment that SeO32− levels greater than 50 g.ha−1 decreased rice yields by affecting the photosynthetic integrity of plants while also disrupting their chlorophyll fluorescence [88]. With an abundance of Se-based supplements, the PSII was mostly suppressed because of abnormal changes in Fv/Fo and Fv/Fm ratios, resulting in a decrease in maximum quantum yield, a limited ability to uphold the normality of PSII functions, associated with a loss of thylakoid-membrane integrity [89]. Indicated ultrastructural adjustments, and chloroplast modifications, with a loss of appressed domains, due to the fact that toxic levels of SeO42− destabilize chloroplasts [89]. In effect, electron transport chains are inhibited by such toxic levels. Cell size, cellular activity, and maintenance are all involved in rendering photosynthesis optimal. Compared to the regulation of plant development, the normality of photosynthetic activity is more susceptible to Se toxicity. Excess amounts of Se-based compounds can disrupt the appropriate ratios of necessary minerals in crops, thereby instigating unwanted fluctuations in the availability of nutrient contents in plant cells. Se ions can interfere with the regularity of nutrient levels, destabilizing the physiological functions that they control. The interplay between Se and essential elements is proportional, resulting in synergistic and antagonistic impacts. In maize, the application of SeO32− (5–100 μM) led to high levels of calcium and phosphorus in the aerial parts of plants, whereas potassium was reduced [90]. Furthermore, Gui et al. [69] discovered that SeO32− (10–100 mg kg−1) suppressed the activity of roots in absorbing K, P, magnesium (Mg), copper (Cu), arsenic (As), and zinc (Zn) in Chinese brake fern (Pteris vittata L.). Se-based compounds reportedly altered the coefficients of permeability for certain ions, influencing their ability to cross membranes [91]. In contrast, some plant species cannot accumulate Se because Se-based amino acids fail to be replaced specifically with other viable Se equivalents. As a result, Se-susceptible crops incorporate Se into their protein structures, and, thus, render the proteins dysfunctional. In contrast, Se-tolerant species avoid incorporating Se into their proteins. Two amino acids are Se-based, i.e., selenocystathione and methylselenocysteine, which are normally not incorporated into protein structures in Se-susceptible species [92]. In Arabidopsis, the application of SeO32− led to cellular membrane adjustments and regulatory changes in stomatal behavior. SeO32− decreased the number of stomata in the leaves and instigated an intense condition of oxidative stress, as well as a medium degree of nitrosative stress [28]. Brassica juncea, on the other hand, responded to SeO32− by opening the stomata and accumulating more pectin. As a result, the plants developed severe nitrosative stress and a medium level of oxidative stress. The authors proposed that resistance to SeO32− partly emanates from the ability of plants to control oxidation processes. Kolbert et al. [93] demonstrated that the toxic effects of Se target crop proteomes, and, thus, result in the formation of not just oxy- and seleno-proteins, but nitro-proteins as well [93]. Crops that withhold Se-Cys from protein biosynthesis could indeed reduce Se-induced proteomic damages. Proteasome compounds are capable of removing old or deformed selenoproteins, nitroproteins, and oxyproteins.
3.2 Regulation of Phytohormones Biosynthesis Genes by Se
Se has key roles as a macronutrient in a wide range of organisms, although at high concentrations it can impede gene expression. While plants respond variedly to Se, supplementary amounts of Se are generally required to compensate for Se-deficient conditions. Other treatments may contribute to the cleaning up of Se-polluted soils. Plant hormones, e.g., salicylic acid, ethylene, and jasmonic acid, are tasked with triggering Se resistance and, according to biochemical and molecular research, aiding non-accumulator crops in their tolerance to Se toxicity. Non-accumulator crops are plants that cannot accumulate Se; otherwise, they will suffer from physiological dysfunctions. As phytohormones are generated, tolerance to Se toxicity is aided by processes that enhance ROS scavenging, as well as phytohormones that coordinate an appropriate amount of Se-uptake. When Se-rich soils become saturated with moisture, selenate becomes more likely to permeate shallow aquifers [94]. High levels of Se absorption from the soil profile can sometimes be perilous for animals and humans if they regularly include Se-affected plants in their diet [95]. Due to the chemical similarity between sulfur (S) and Se, sulfate transporters in plant cells may take up Se along with S and, even worse, integrate Se into the structure of methionine (Met) and cysteine (Cys) analogs, as well as seleno-Met (SeMet) and seleno-Cys (SeCys) [96]. Substitutions of important S amino acids, i.e., Met and Cys in proteins, with Se-analogs, can become lethal to plant cells because they restrict S bridge forming and/or distort protein formulation [97]. A significant portion of studies on Se-resistance elaborated on how S interacts with Se whenthe former is metabolized in plants. Based on recent findings, Se induces responses in the defensive system, often reacting hypersensitively to abiotic and biotic stressors [98]. An interplay between several induced responses forms an integral part of Se-resistance in both accumulator and non-accumulator crops [99]. A complete evaluation of Se-receptive gene clusters in A. thaliana, a non-accumulator species, revealed the participation of defensive phytohormones in imparting Se-resistance to plants. In fact, transcriptome analyses have been aimed at recognizing selenate-receptive gene clusters. Treating plants with appropriate amounts of selenate increased the rates at which jasmonic acid (JA)- and ethylene-responsive genes were expressed [100]. Furthermore, extensive amounts of analyses on gene expression revealed that most of these responsive genes were induced in crops treated with selenate [98]. Specifically, Se-treated plants showed a greater level of induction for several genes, i.e., pathogenesis-related 4 (At3g04720, PR4), ethylene response factor 1 (At3g23240, ERF1), vegetative storage protein 1 (At5g24780, VSP1), plant defense in 1.2 (At5g44420, PDF1.2), JA-responsive gene (At3g16470, JR), and proteinase inhibitor 2 (At2g02100, PIN2) [98]. Furthermore, important enzymes were expressed in larger amounts by genes responsible for the synthesis of 1-aminocyclopropane-1-carboxylate synthase 6 (At4g11280, ACS6), S-adenosyl-Met synthase (At1g02500, SAM), and ethylene [101], in addition to allene-oxide synthase (At5g42650, AOS) and lipoxygenase 2 (At3g45140, LOX2) genes. These were reportedly involved in JA biosynthesis [98]. Relevant findings indicated that JA and ethylene production rates usually differ in response to Se-treatments. Exogenous selenite increased the accumulation of JA and ethylene molecules less significantly in a selenite-susceptible accession ‘Ws-2’, compared to a selenite-tolerant accession ‘Col-0’ [102]. Thus, it can be inferred that Se-tolerance can be induced with the help of JA and ethylene in non-accumulator plants. The role of JA and ethylene in Se-tolerance has been explored in non-accumulators while considering their biosynthetic pathway or signaling. Mutations were identified in the Se-tolerant accession ‘Col-0’, in its acs6 locus which lacked ethylene-producing signs due to a mutation in the ACS6 gene. Also, there were mutations in ein2 which caused a lack in ethylene-signaling [103] and jar1 which lacked the ability to synthesize standard JA after the mutation [104]. The above mutants seemed to be more susceptible to selenate and selenite, compared to the susceptibility of the wild Col-0 genotype [105]. In contrast, a test was conducted on ‘Ws-2’, as a susceptible accession, to determine whether cellular tolerance to selenite was restricted by smaller amounts of JA buildup and ethylene release. The results demonstrated that the provision of MeJA or 1-amino-cyclopropane-1-carboxylic acid (ACC, an ethylene precursor) increased cellular tolerance to selenite in the ‘Ws-2’ accession [98]. Such findings implied that ethylene and JA are able to contribute to Se-tolerance in plants. Some other defensive patterns reportedly involved phytohormones and salicylic acid (SA) which accumulated in selenite-treated A. thaliana [106]. Among the phenylpropanoids, the biosynthesis of SA is triggered by various biotic and abiotic stressors [107]. In A. thaliana, isochorismate-synthase 1 (ICS1) encoded an enzyme that limited the rate of SA biosynthesis [108]. Higher amounts of SA accumulated in plant cells and the ICS1 gene was expressed more in response to exogenous selenite. This was observed in the Se-resistant accession ‘Col-0’ and also in the Se-susceptible accession ‘Ws-2’ [109]. In some cases, mutations in SID2 and NPR1 genes have reportedly hampered the biosynthesis and signaling of SA, respectively [110]. Nonetheless, no significant variation was observed in selenite tolerance, compared to the non-mutant genotype, ‘Col-0’, which is an Se-tolerant accession. Even so, using SA on these plants made them more susceptible to selenite [109]. In comparison with the role of JA and ethylene, Se-tolerance is rather suppressed by accumulated amounts of SA in plants. The exact mechanism of SA’s negative impact on Se-tolerance still seems unknown, but an interplay among JA, ethylene, and SA signaling can be a plausible cause. In fact, relevant research on phytohormones revealed their effective role in relieving biotic and abiotic stress in crops, possibly through a mutually antagonistic or coordinated manner. Regarding A. thaliana, many recent genetic experiments have shown that SA can affect JA-signaling antagonistically. For example, pad4 and eds4 mutants lacked accumulated amounts of SA, and, thus, responded favorably to elicitors of gene expressions dependent on JA [111]. Mutations in several JA-signaling proteins, suppressors of SA insensitivity2 (ssi2), kinase4 (mpk4), and coronatine insensitive1 (coi1), on the other hand, demonstrated that JA-signaling antagonizes the appropriate regulation of SA-related defense lines in A. thaliana [112]. Accordingly, SA is thought to suppress the action of ACC oxidase for the complete process in ethylene synthesis [113]. The suppression of SA signaling pathways via ethylene has been proven by transcriptome analyses and mutants that are not sensitive to ethylene [98]. Experimentally, enhanced Se-sensitivity can be associated with the existence of SA and could be mediated by the suppression of ethylene and JA-signaling pathways.
4 Selenium and Its Role in Secondary Metabolism
Many plants are known to synthesize and accumulate SMs throughout their life cycles. SMs are an indispensable group of compounds that counter pathogenic attacks. They are usually present in unique tissues and cells (e.g., epidermal cells and trichomes), and, thus, are known as phytoanticipins. Secondary metabolites are generated individually in response to the pathogenic presence and/or phytoalexins, the synthesis of which is induced by invading pathogens [114]. These substances defend against pathogenic microbes, viruses, herbivores (primarily insects), and other crops [115]. Understanding applicable pathways of biosynthetic mechanisms and the type of stimulation that determines their synthesis could be implemented in plant tissue culture and cellular metabolomics, primarily because Secondary metabolites have significant medical benefits [116]. Terpenes, plant volatiles, carotenoids, and phenolic substances include coumarins, tannins, flavonoids, and lignin. Together with sulfur- and nitrogen-containing substances (including cyanogenic glucosides, alkaloids, and non-protein amino acids or glucosinolate), they are major categories of Secondary metabolites in plants [117].
The Se/S quotients of shoots are mostly similar among angiosperms when tested under exactly equal climatic factors, and crops that accumulate an excess of S also accumulate more Se [118]. Secondary Se metabolite complementation and biosynthesis in plants appear to resemble aspects of S-based metabolites. Se could be found in a variety of Se-based SMs that result from SeMet and SeCys. Selenoglutathione seems to be a Se-metabolite with prominent roles. It is produced by the actions of glutamylcysteine synthetase and glutathione synthetase from SeCys, glutamate, and glycine [119], Se-allyl L-cysteine sulfoxide, compounds that result from SeCys directly or are generated by selenoglutathione indirectly in alliums [120]. Selenoglucosinolates can be found in tandem with Se-aglycons, generated by SeMet in brassicas species [121]. Surprisingly, despite the presence of Se-precursor compounds among the existing glucosinolate varieties synthesized by Brassicas, aromatic glucosinolates are derived from tyrosine and phenylalanine, while indolic glucosinolates are derived from aliphatic glucosinolate compounds,tryptophan, alanine, leucine, valine, and isoleucine [122]. With the presence of Se-metabolites, selenosugars are likely to be derived from cell walls in Se-treated crops [123].
5 Does Se Regulate Secondary Metabolites?
The effect of Se as a beneficial micronutrient on plants is dose-dependent. There are some literatures reporting protection role of Se for plants against abiotic stresses such as cold, drought and heavy metals [39]. A reduced amount of heavy metal absorption, detoxification, and chelation, along with a decline in ROS by antioxidant activity, are primary pathways in which Se mitigates the adverse effects of stress on plants [50]. Handa et al. [124] discovered that the application of Se assisted in crop health by restoring plant development and defense systems via improvements in SMs (Secondary Metabolites)against chromium toxicity in Brassica juncea. Additionally, using Se treatments on Melissa officinalis improved the ascorbic acid content, plant biomass, SMs content (e.g., caryophyllene oxide and caryophyllene), and proteins [51]. Nonetheless, whether Se has constructive effects depends on its dose, due to its ability to compete with sulfur, sometimes resulting in a decreased amount of sulfate absorption by plants, and partially due to the release of H2O2 [50]. Se was reportedly capable of ROS-scavenging via superoxide dismutase [125]. In spite of being a very simple molecule, H2O2 has a key role in cellular signaling, by oxidative modulation of the activity of redox sensitive proteins. It also affects the production of defensive secondary metabolites through the regulation of expression and activities of defense-related genes [126]. Changing H2O2 homeostasis, however, promotes hormone-related pathways for signaling and receptive patterns that distinguish changes in cells, allowing regular functions to be maintained in plant physiology. Phytohormones are important endogenous variables in cellular responses to stress [127]. There is limited knowledge of how Se can change the biosynthesis of phytohormones and secondary metabolites [127]. When small doses are applied, Se usually boosts the efficiency of antioxidants in non-accumulator crops. In this regard, using a moderate dose of Se (5 μM) on Melissa officinalis led to greater amounts of citral, z-citral, and geranyl acetate, whereas a low dose (0.2 μM) accelerated the synthesis of caryophyllene oxide in the essential oil. Also, the maximum amount of ascorbic acid occurred in response to this low dose [51]. Nitrogen-based compounds and their synthesis were affected by Se supplements that interacted with sulfur metabolism. Changes in nitrogen assimilation have significant implications for the biosynthesis of nitrogen-based compounds, including phenylpropanoids and alkaloids [118]. Se also increased the alkaloid content in Astragalus verus cells, thereby protecting cellular functions against stressors, although the increase in alkaloid content beyond a specific limit can indeed become problematic for cellular functions. Increasing the amounts of alkaloids in Astragalus verus cells, through a high-dose Se treatment, reduced plant longevity and biomass [128].
6 Conclusions and Prospective Outlooks
The impact of Se on crops changes from beneficial to toxic over a narrow concentration range, as reflected in plant growth and metabolism. There is much interest in the unique role of Se in counteracting the negative effects of various abiotic stresses in plants, rather than in its essentiality. Over the last three decades, substantial amounts of information have been obtained from examining the behavior of Se in crops, such as the patterns of uptake, assimilation, volatilization, toxicity, and tolerance. Even so, the processes through which Se counteracts stress remain unknown. To ensure that crops perform the most from Se application, appropriate amounts of Se form must be outlined for each stage of crop growth, species, plant size, method of application, the form of Se, and, most pertinently, the accumulation of Se. Tolerance to abiotic stresses can be improved by Se, although this requires precise evaluations to reveal the extent and manner of its effectiveness. While food products and fodders can be potentially biofortified with the application of Se, there is limited information on a few plants in this regard. Se toxicity is commonly linked to the synthesis of Se-based proteins, the production of ROS, and the oxidative stress that ensues, all of which lead to disruptions in plant physiology. Small doses of Se can boost the activity of antioxidants, thereby offering an intriguing area of research. Furthermore, finding the most appropriate dosages, researching Se-susceptible and Se-accumulator crops, and analyzing the dynamics of Seas an activator or inhibitor of biochemical pathways require extensive research. High Se levels in and around hyperaccumulators harm Se-sensitive partners while simultaneously providing a niche for Se tolerant expert partners. The negative impacts on generalist herbivores and Se-sensitive plants benefit the plants by reducing herbivory and decreasing the amount of nearby vegetation. High Se concentrations in and around hyperaccumulators may have functioned as a selective pressure for the evolution of Se-tolerant herbivores, pollinators, detrivores, fungi, and surrounding plants. Similarly, the evolution of Se-tolerant herbivores and pathogens may have led to the evolution of Se hyperaccumulation to ever-increasing levels. The existence of a Se-tolerant that may aggregate Se to significant concentrations at different trophic levels most likely leads to Se transport in the local food chain and, eventually, Se cycling in the local environment. The entry point into this movement is the Se hyperaccumulator plants, which may play a major role. At the moment, nothing is known regarding the proportional role of terrestrial plants in global Se cycling.
Transcriptome analyses have shed light on how Arabidopsis plants respond to Se-induced stress and Se-tolerance. Jasmonic acid and ethylene usually serve as signaling agents that regulate plant tolerance to selenite. Se and phytohormone interactions are perceived to regulate genes that control the biosynthesis of S-based compounds, as well as the absorption of S and its assimilation. In turn, these factors affect plant development and growth under standard and stressful conditions. Several fields of omics, such as transcriptomics, genomics, metabolomics, and proteomics can offer a wide range of tools that can assist in determining the amounts of Se that are beneficial or harmful to each plant species. Furthermore, carrying out future research on ways to facilitate Se-mediated metabolic processes could add to our present understanding of Se-dynamics and help identify the correct Se-mediated stress response.
Funding Statement: The authors received no specific funding for this study.
Conflicts of Interest: The authors declare that they have no conflicts of interest to report regarding the present study.
References
1. Floor, G. H., Román-Ross, G. (2012). Selenium in volcanic environments: A review. Applied Geochemistry, 27(3), 517–531. [Google Scholar]
2. Kleiber, T., Krzesiński, W., Przygocka-Cyna, K., Spiżewski, T. (2018). Alleviation effect of selenium on manganese stress of plants. Ecological Chemistry and Engineering, 25(1), 143–152. [Google Scholar]
3. Bybordi, A., Saadat, S., Zargaripour, P. (2018). The effect of zeolite, selenium and silicon on qualitative and quantitative traits of onion grown under salinity conditions. Archives of Agronomy and Soil Science, 64(4), 520–530. [Google Scholar]
4. Varlamova, E. G., Cheremushkina, I. V. (2017). Contribution of mammalian selenocysteine-containing proteins to carcinogenesis. Journal of Trace Elements in Medicine and Biology, 39, 76–85. [Google Scholar] [PubMed]
5. Sharapov, M. G., Novoselov, V. I., Gudkov, S. V. (2019). Radioprotective role of peroxiredoxin 6. Antioxidants, 8(1), 15–37. [Google Scholar] [PubMed]
6. Sharapov, M. G., Novoselov, V. I. (2019). Catalytic and signaling role of peroxiredoxins in carcinogenesis. Biochemistry, 84, 79–100. [Google Scholar] [PubMed]
7. Zhang, Y., Roh, Y. J., Han, S. J., Park, I., Lee, H. M. et al. (2020). Role of selenoproteins in redox regulation of signaling and the antioxidant system: A review. Antioxidants, 9(5), 383. [Google Scholar] [PubMed]
8. Gudkov, S. V., Shafeev, G. A., Glinushkin, A. P., Shkirin, A. V., Barmina, E. V. et al. (2020). Production and use of selenium nanoparticles as fertilizers. ACS Omega, 5(28), 17767–17774. [Google Scholar] [PubMed]
9. D’Amato, R., Regni, L., Falcinelli, B., Mattioli, S., Benincasa, P. et al. (2020). Current knowledge on selenium biofortification to improve the nutraceutical profile of food: A comprehensive review. Journal of Agricultural and Food Chemistry, 68(14), 4075–4097. [Google Scholar] [PubMed]
10. Bodnar, M., Konieczka, P., Namiesnik, J. (2012). The properties, functions, and use of selenium compounds in living organisms. Journal of Environmental Science and Health, Part C, 30(3), 225–252. [Google Scholar]
11. Hasanuzzaman, M., Nahar, K., Alam, M., Fujita, M. (2014). Modulation of antioxidant machinery and the methylglyoxal detoxification system in selenium-supplemented Brassica napus seedlings confers tolerance to high temperature stress. Biological Trace Element Research, 161(3), 297–307. [Google Scholar] [PubMed]
12. Pereira, A. S., Dorneles, A. O. S., Bernardy, K., Sasso, V. M., Bernardy, D. P. et al. (2018). Selenium and silicon reduce cadmium uptake and mitigate cadmium toxicity in Pfaffia glomerata (Spreng.) Pedersen plants by activation antioxidant enzyme system. Environmental Science and Pollution Research, 25(19), 18548–18558. [Google Scholar] [PubMed]
13. Sattar, A., Cheema, M. A., Sher, A., Ijaz, M., Ul-Allah, S. et al. (2019). Physiological and biochemical attributes of bread wheat (Triticum aestivum L.) seedlings are influenced by foliar application of silicon and selenium under water deficit. Acta Physiologiae Plantarum, 41(8), 1–11. [Google Scholar]
14. Mata-Ramírez, D., Serna-Saldívar, S. O., Antunes-Ricardo, M. (2019). Enhancement of anti-inflammatory and antioxidant metabolites in soybean (Glycine max) calluses subjected to selenium or UV-light stresses. Scientia Horticulturae, 257, 108669. [Google Scholar]
15. Huang, C., Qin, N., Sun, L., Yu, M., Hu, W. et al. (2018). Selenium improves physiological parameters and alleviates oxidative stress in strawberry seedlings under low-temperature stress. International Journal of Molecular Sciences, 19(7), 1913. [Google Scholar] [PubMed]
16. Elkelish, A. A., Soliman, M. H., Alhaithloul, H. A., El-Esawi, M. A. (2019). Selenium protects wheat seedlings against salt stress-mediated oxidative damage by up-regulating antioxidants and osmolytes metabolism. Plant Physiology and Biochemistry, 137, 144–153. [Google Scholar] [PubMed]
17. Chauhan, R., Awasthi, S., Tripathi, P., Mishra, S., Dwivedi, S. et al. (2017). Selenite modulates the level of phenolics and nutrient element to alleviate the toxicity of arsenite in rice (Oryza sativa L.). Ecotoxicology and Environmental Safety, 138, 47–55. [Google Scholar] [PubMed]
18. Hemmati, M., Delkhosh, B., Rad, A. H. S., Mohammadi, G. N. (2019). Effect of the application of foliar selenium on canola cultivars as influenced by different irrigation regimes. Journal of Agricultural Sciences, 25(3), 309–318. [Google Scholar]
19. Hawrylak-Nowak, B., Dresler, S., Rubinowska, K., Matraszek-Gawron, R., Woch, W. et al. (2018). Selenium biofortification enhances the growth and alters the physiological response of lamb’s lettuce grown under high temperature stress. Plant Physiology and Biochemistry, 127, 446–456. [Google Scholar] [PubMed]
20. Rasool, A., Shah, W. H., Mushtaq, N. U., Saleem, S., Hakeem, K. R. et al. (2022). Amelioration of salinity induced damage in plants by selenium application: A review. South African Journal of Botany, 147, 98–105. [Google Scholar]
21. Aissa, N., Malagoli, M., Radhouane, L. (2018). An approach to alleviate the impact of drought stress with selenium amendment. Iranian Journal of Science and Technology, Transactions A: Science, 42, 283–288. [Google Scholar]
22. Tadina, N., Germ, M., Kreft, I., Breznik, B., Gaberščik, A. (2007). Effects of water deficit and selenium on common buckwheat (Fagopyrum esculentumMoench) plants. Photosynthetica, 45, 472–476. [Google Scholar]
23. Sieprawska, A., Kornas, A., Filek, M. (2015). Involvement of selenium in protective mechanisms of plants under environmental stress conditions--review. Acta Biologica Cracoviensia Series Botanica, 57(1), 9–20. [Google Scholar]
24. Golob, A., Kugovnik, A., Kreft, I., Gaberščik, A., Germ, M. (2019). The interactions between UV radiation, drought and selenium in different buckwheat species. Acta Biological Slovenica, 62, 57–66. [Google Scholar]
25. Castillo-Godina, R., Foroughbakhch-Pournavab, R., Benavides-Mendoza, A. (2016). Effect of selenium on elemental concentration and antioxidant enzymatic activity of tomato plants. Journal of Agricultural Science and Technology, 18(1), 233–244. [Google Scholar]
26. Yildiztugay, E., Ozfidan-Konakci, C., Kucukoduk, M., Tekis, S. A. (2017). The impact of selenium application on enzymatic and non-enzymatic antioxidant systems in Zea mays roots treated with combined osmotic and heat stress. Archives of Agronomy and Soil Science, 63(2), 261–275. [Google Scholar]
27. Balakhnina, T., Nadezhkina, E. (2017). Effect of selenium on growth and antioxidant capacity of Triticum aestivum L. during development of lead-induced oxidative stress. Russian Journal of Plant Physiology, 64(2), 215–223. [Google Scholar]
28. Molnár, Á., Kolbert, Z., Kéri, K., Feigl, G., Ördög, A. S. et al. (2018). Selenite-induced nitro-oxidative stress processes in Arabidopsis thaliana and Brassica juncea. Ecotoxicology and Environmental Safety, 148, 664–674. [Google Scholar]
29. Mahmud, J. A., Bhuyan, M., Anee, T. I., Nahar, K., Fujita, M. et al. (2019). Reactive oxygen species metabolism and antioxidant defense in plants under metal/metalloid stress. In: Plant abiotic stress tolerance, pp. 221–257. Springer Nature. https://doi.org/10.1007/978-3-030-06118-0_10 [Google Scholar] [CrossRef]
30. Fayram, A. H. (2022). Relative importance of two correlated variables on aquatic macroinvertebrate communities in a Colorado Front Range river: Selenium and urbanization. Environmental Monitoring and Assessment, 194(10), 1–11. [Google Scholar]
31. Sharma, A., Gupta, S., Negi, N. P., Patel, D. P., Raina, M. et al. (2022). Selenium and nano-selenium-mediated drought stress tolerance in plants. In: Selenium and nano-selenium in environmental stress management and crop quality improvement, pp. 121–148. Cham: Springer. https://doi.org/10.1007/978-3-031-07063-1_7 [Google Scholar] [CrossRef]
32. Puccinelli, M., Malorgio, F., Pezzarossa, B. (2017). Selenium enrichment of horticultural crops. Molecules, 22(6), 933. [Google Scholar] [PubMed]
33. Lapaz, A. D. M., Santos, L. F. D. M., Yoshida, C. H. P., Heinrichs, R., Campos, M. et al. (2019). Physiological and toxic effects of selenium on seed germination of cowpea seedlings. Bragantia, 78, 498–508. [Google Scholar]
34. Banerjee, A., Roychoudhury, A. (2019). Role of selenium in plants against abiotic stresses: Phenological and molecular aspects. Molecular Plant Abiotic Stress: Biology and Biotechnology, 123–133. https://doi.org/10.1002/9781119463665.ch7 [Google Scholar] [CrossRef]
35. Hasanuzzaman, M., Bhuyan, M. B., Raza, A., Hawrylak-Nowak, B., Matraszek-Gawron, R. et al. (2020). Selenium in plants: Boon or bane? Environmental and Experimental Botany, 178, 104170. [Google Scholar]
36. Shekari, L., Aroiee, H., Mirshekari, A., Nemati, H. (2019). Protective role of selenium on cucumber (Cucumis sativus L.) exposed to cadmium and lead stress during reproductive stage role of selenium on heavy metals stress. Journal of Plant Nutrition, 42(5), 529–542. [Google Scholar]
37. Kaur, N., Sharma, S., Kaur, S., Nayyar, H. (2014). Selenium in agriculture: A nutrient or contaminant for crops? Archives of Agronomy and Soil Science, 60(12), 1593–1624. [Google Scholar]
38. Wang, N., Tan, H. Y., Li, S., Xu, Y., Guo, W. et al. (2017). Supplementation of micronutrient selenium in metabolic diseases: Its role as an antioxidant. Oxidative Medicine and Cellular Longevity, 2017, 7478523. [Google Scholar]
39. Gupta, M., Gupta, S. (2017). An overview of selenium uptake, metabolism, and toxicity in plants. Frontiers in Plant Science, 7, 2074. [Google Scholar] [PubMed]
40. Djanaguiraman, M., Devi, D. D., Shanker, A. K., Sheeba, J. A., Bangarusamy, U. (2005). Selenium-an antioxidative protectant in soybean during senescence. Plant and Soil, 272(1), 77–86. [Google Scholar]
41. Bekheta, M., Abbas, S., El-Kobisy, O., Mahgoub, M. (2008). Influence of selenium and paclobutrazole on growth, metabolic activities and anatomical characters of Gebera Jasmonii L. Australian Journal of Basic and Applied Sciences, 2(4), 1284–1297. [Google Scholar]
42. Cartes, P., Jara, A., Pinilla, L., Rosas, A., Mora, M. (2010). Selenium improves the antioxidant ability against aluminium-induced oxidative stress in ryegrass roots. Annals of Applied Biology, 156(2), 297–307. [Google Scholar]
43. Ansary, M., Rahman, W., Sakib, M., Islam, T. (2022). Application of selenium and nano-selenium in abiotic stress management, crop improvement, and agro-biotechnology. In: Selenium and nano-selenium in environmental stress management and crop quality improvement, pp. 271–310. Cham: Springer. https://doi.org/10.1007/978-3-031-07063-1_14 [Google Scholar] [CrossRef]
44. Haghighi, M., Sheibanirad, A., Pessarakli, M. (2016). Effects of selenium as a beneficial element on growth and photosynthetic attributes of greenhouse cucumber. Journal of Plant Nutrition, 39(10), 1493–1498. [Google Scholar]
45. Shekari, L., Kamelmanesh, M., Mozafarian, M., Sadeghi, F. (2016). Beneficial effects of selenium on some morphological and physiological trait of hot pepper (Capsicum anuum). Majallah-iUlum-i Bāghbānī, 29(4), 594–600. [Google Scholar]
46. Mroczek-Zdyrska, M., Strubińska, J., Hanaka, A. (2017). Selenium improves physiological parameters and alleviates oxidative stress in shoots of lead-exposed Vicia faba L. minor plants grown under phosphorus-deficient conditions. Journal of Plant Growth Regulation, 36(1), 186–199. [Google Scholar]
47. Ashraf, M. A., Akbar, A., Parveen, A., Rasheed, R., Hussain, I. et al. (2018). Phenological application of selenium differentially improves growth, oxidative defense and ion homeostasis in maize under salinity stress. Plant Physiology and Biochemistry, 123, 268–280. [Google Scholar] [PubMed]
48. Alyemeni, M. N., Ahanger, M. A., Wijaya, L., Alam, P., Bhardwaj, R. et al. (2018). Selenium mitigates cadmium-induced oxidative stress in tomato (Solanum lycopersicum L.) plants by modulating chlorophyll fluorescence, osmolyte accumulation, and antioxidant system. Protoplasma, 255(2), 459–469. [Google Scholar] [PubMed]
49. Handa, N., Kohli, S., Sharma, A., Thukral, A., Bhardwaj, R. et al. (2018). Selenium ameliorates chromium toxicity through modifications in pigment system, antioxidative capacity, osmotic system, and metal chelators in Brassica juncea seedlings. South African Journal of Botany, 119, 1–10. [Google Scholar]
50. Alves, L. R., Rossatto, D. R., Rossi, M. L., Martinelli, A. P., Gratão, P. L. (2020). Selenium improves photosynthesis and induces ultrastructural changes but does not alleviate cadmium-stress damages in tomato plants. Protoplasma, 257(2), 597–605. [Google Scholar] [PubMed]
51. Tavakoli, S., Enteshari, S., Yousefifard, M. (2020). Investigation of the effect of selenium on growth, antioxidant capacity and secondary metabolites in Melissa officinalis. Iranian Journal of Plant Physiology, 10(2), 3125–3134. [Google Scholar]
52. Dai, Z., Imtiaz, M., Rizwan, M., Yuan, Y., Huang, H. et al. (2019). Dynamics of selenium uptake, speciation, and antioxidant response in rice at different panicle initiation stages. Science of the Total Environment, 691, 827–834. [Google Scholar] [PubMed]
53. Rady, M. M., Belal, H. E., Gadallah, F. M., Semida, W. M. (2020). Selenium application in two methods promotes drought tolerance in Solanum lycopersicum plant by inducing the antioxidant defense system. Scientia Horticulturae, 266, 109290. [Google Scholar]
54. Manaf, H. H. (2016). Beneficial effects of exogenous selenium, glycine betaine and seaweed extract on salt stressed cowpea plant. Annals of Agricultural Sciences, 61(1), 41–48. [Google Scholar]
55. Jiang, C., Zu, C., Lu, D., Zheng, Q., Shen, J. et al. (2017). Effect of exogenous selenium supply on photosynthesis, Na+ accumulation and antioxidative capacity of maize (Zea mays L.) under salinity stress. Scientific Reports, 7(1), 1–14. [Google Scholar]
56. Habibi, G. (2017). Physiological, photochemical and ionic responses of sunflower seedlings to exogenous selenium supply under salt stress. Acta Physiologiae Plantarum, 39(10), 1–9. [Google Scholar]
57. Astaneh, R. K., Bolandnazar, S., Nahandi, F. Z., Oustan, S. (2019). Effects of selenium on enzymatic changes and productivity of garlic under salinity stress. South African Journal of Botany, 121, 447–455. [Google Scholar]
58. Hasanuzzaman, M., Fujita, M. (2011). Selenium pretreatment upregulates the antioxidant defense and methylglyoxal detoxification system and confers enhanced tolerance to drought stress in rapeseed seedlings. Biological Trace Element Research, 143(3), 1758–1776. [Google Scholar] [PubMed]
59. Habibi, G. (2013). Effect of drought stress and selenium spraying on photosynthesis and antioxidant activity of spring barley/Ucinek susnega stresa in skropljenja s selenom na fotosintezo in antioksidativno aktivnost jarega jecmena. Acta Agriculturae Slovenica, 101(1), 31–39. [Google Scholar]
60. Proietti, P., Nasini, L., Del Buono, D., D’Amato, R., Tedeschini, E. et al. (2013). Selenium protects olive (Olea europaea L.) from drought stress. Scientia Horticulturae, 164, 165–171. [Google Scholar]
61. Djanaguiraman, M., Prasad, P. V., Seppanen, M. (2010). Selenium protects sorghum leaves from oxidative damage under high temperature stress by enhancing antioxidant defense system. Plant Physiology and Biochemistry, 48(12), 999–1007. [Google Scholar] [PubMed]
62. Chandra, S., Roychoudhury, A. (2020). Role of selenium and manganese in mitigating oxidative damages. Protective Chemical Agents in the Amelioration of Plant Abiotic Stress: Biochemical and Molecular Perspectives, 597–621. https://doi.org/10.1002/9781119552154.ch30 [Google Scholar] [CrossRef]
63. Iqbal, M., Hussain, I., Liaqat, H., Ashraf, M. A., Rasheed, R. et al. (2015). Exogenously applied selenium reduces oxidative stress and induces heat tolerance in spring wheat. Plant Physiology and Biochemistry, 94, 95–103. [Google Scholar] [PubMed]
64. Balal, R. M., Shahid, M. A., Javaid, M. M., Iqbal, Z., Anjum, M. A. et al. (2016). The role of selenium in amelioration of heat-induced oxidative damage in cucumber under high temperature stress. Acta Physiologiae Plantarum, 38(6), 1–14. [Google Scholar]
65. Hawrylak-Nowak, B., Dresler, S., Rubinowska, K., Matraszek-Gawron, R., Woch, W. et al. (2018). Selenium biofortification enhances the growth and alters the physiological response of lamb’s lettuce grown under high temperature stress. Plant Physiology and Biochemistry, 127, 446–456. [Google Scholar] [PubMed]
66. Hawrylak-Nowak, B., Dresler, S., Wójcik, M. (2014). Selenium affects physiological parameters and phytochelatins accumulation in cucumber (Cucumis sativus L.) plants grown under cadmium exposure. Scientia Horticulturae, 172, 10–18. [Google Scholar]
67. Rizwan, M., Ali, S., Rehman, M. Z. U., Rinklebe, J., Tsang, D. C. et al. (2021). Effects of selenium on the uptake of toxic trace elements by crop plants: A review. Critical Reviews in Environmental Science and Technology, 51(21), 2531–2566. [Google Scholar]
68. Filek, M., Keskinen, R., Hartikainen, H., Szarejko, I., Janiak, A. et al. (2008). The protective role of selenium in rape seedlings subjected to cadmium stress. Journal of Plant Physiology, 165(8), 833–844. [Google Scholar] [PubMed]
69. Gui, J. -Y., Rao, S., Huang, X., Liu, X., Cheng, S. et al. (2022). Interaction between selenium and essential micronutrient elements in plants: A systematic review. Science of the Total Environment, 853, 158673. [Google Scholar] [PubMed]
70. Srivastava, M., Ma, L. Q., Rathinasabapathi, B., Srivastava, P. (2009). Effects of selenium on arsenic uptake in arsenic hyperaccumulator Pteris vittata L. Bioresource Technology, 100(3), 1115–1121. [Google Scholar] [PubMed]
71. Hasanuzzaman, M., Hossain, M. A., Fujita, M. (2012). Exogenous selenium pretreatment protects rapeseed seedlings from cadmium-induced oxidative stress by upregulating antioxidant defense and methylglyoxal detoxification systems. Biological Trace Element Research, 149(2), 248–261. [Google Scholar] [PubMed]
72. Mozafariyan, M., Shekari, L., Hawrylak-Nowak, B., Kamelmanesh, M. M. (2014). Protective role of selenium on pepper exposed to cadmium stress during reproductive stage. Biological Trace Element Research, 160(1), 97–107. [Google Scholar] [PubMed]
73. Malerba, M., Cerana, R. (2018). Effect of selenium on the responses induced by heat stress in plant cell cultures. Plants, 7(3), 64. [Google Scholar] [PubMed]
74. Hawrylak-Nowak, B. (2022). Biological activity of selenium in plants: Physiological and biochemical mechanisms of phytotoxicity and tolerance. In: Selenium and nano-selenium in environmental stress management and crop quality improvement, pp. 341–363. Springer. https://doi.org/10.1007/978-3-031-07063-1_17 [Google Scholar] [CrossRef]
75. Iqbal, M., Shafiq, F., Anwar, S., Akram, N. A., Ashraf, M. A. et al. (2022). Selenium and nano-selenium-mediated heat stress tolerance in plants. In: Selenium and nano-selenium in environmental stress management and crop quality improvement, pp. 149–171. Springer. https://doi.org/10.1007/978-3-031-07063-1_8 [Google Scholar] [CrossRef]
76. Liu, H., Shi, Z., Li, J., Zhao, P., Qin, S. et al. (2018). The impact of phosphorus supply on selenium uptake during hydroponics experiment of winter wheat (Triticum aestivum) in China. Frontiers in Plant Science, 9, 373. [Google Scholar] [PubMed]
77. Wu, Z., Yin, X., Bañuelos, G. S., Lin, Z. Q., Liu, Y. et al. (2016). Indications of selenium protection against cadmium and lead toxicity in oilseed rape (Brassica napus L.). Frontiers in Plant Science, 7, 1875. [Google Scholar] [PubMed]
78. Sharma, V. K., McDonald, T. J., Sohn, M., Anquandah, G. A., Pettine, M. et al. (2017). Assessment of toxicity of selenium and cadmium selenium quantum dots: A review. Chemosphere, 188, 403–413. [Google Scholar] [PubMed]
79. Chen, H., Zhang, M., Qu, Z., Xie, B. (2008). Antioxidant activities of different fractions of polysaccharide conjugates from green tea (Camellia sinensis). Food Chemistry, 106(2), 559–563. [Google Scholar]
80. Feng, T., Chen, S., Gao, D., Liu, G., Bai, H. et al. (2016). Selenium improves photosynthesis and protects photosystem II in pear (Pyrus bretschneiderigrape (Vitis viniferaand peach (Prunus persica). Photosynthetica, 53(4), 609–612. [Google Scholar]
81. Vítová, M., Bišová, K., Hlavová, M., Zachleder, V., Rucki, M. et al. (2011). Glutathione peroxidase activity in the selenium-treated alga Scenedesmus quadricauda. Aquatic Toxicology, 102(1–2), 87–94. [Google Scholar]
82. Xue, T., Hartikainen, H., Piironen, V. (2001). Antioxidative and growth-promoting effect of selenium on senescing lettuce. Plant and soil, 237(1), 55–61. [Google Scholar]
83. Li, H., Liu, X., Wassie, M., Chen, L. (2020). Selenium supplementation alleviates cadmium-induced damages in tall fescue through modulating antioxidant system, photosynthesis efficiency, and gene expression. Environmental Science and Pollution Research, 27(9), 9490–9502. [Google Scholar] [PubMed]
84. Feng, T., Chen, S., Gao, D., Liu, G., Bai, H. et al. (2015). Selenium improves photosynthesis and protects photosystem II in pear (Pyrus bretschneiderigrape (Vitis viniferaand peach (Prunus persica). Photosynthetica, 53(4), 609–612. [Google Scholar]
85. Huang, A., Huang, K., Peng, J., Huang, S., Bi, X. et al. (2019). Effects of foliar spraying of selenium fertilizer on selenium-enriched content, heavy metal content and yield of sweet corn grain. Journal of Southern Agriculture, 50(1), 40–44. [Google Scholar]
86. Meeta, J., Mahija, P., Rekha, G. (2017). Influence of selenium supplementation on δ-aminolevulinic acid formation in greening maize leaf segments. Research Journal of Phytochemistry, 11(3), 111–117. [Google Scholar]
87. Hawrylak-Nowak, B., Matraszek, R., Pogorzelec, M. (2015). The dual effects of two inorganic selenium forms on the growth, selected physiological parameters and macronutrients accumulation in cucumber plants. Acta Physiologiae Plantarum, 37(2), 1–13. [Google Scholar]
88. Zhang, M., Tang, S., Huang, X., Zhang, F., Pang, Y. et al. (2014). Selenium uptake, dynamic changes in selenium content and its influence on photosynthesis and chlorophyll fluorescence in rice (Oryza sativa L.). Environmental and Experimental Botany, 107, 39–45. [Google Scholar]
89. Yaghoobizadeh, F., Rajabi Memary, H., Roayaei Ardakani, M. (2021). Bioaccumulation and the effect of selenate concentration on growth and photosynthetic pigment content of spirulina platensis. Journal of Phycological Research, 5(1), 624–641. [Google Scholar]
90. Hawrylak-Nowak, B. (2008). Effect of selenium on selected macronutrients in maize plants. Journal of Elementology, 13(4), 513–519. [Google Scholar]
91. Nawaz, F., Naeem, M., Ashraf, M. Y., Tahir, M. N., Zulfiqar, B. et al. (2016). Selenium supplementation affects physiological and biochemical processes to improve fodder yield and quality of maize (Zea mays L.) under water deficit conditions. Frontiers in Plant Science, 7, 1438. [Google Scholar] [PubMed]
92. Brown, T. A., Shrift, A. (1982). Selenium: Toxicity and tolerance in higher plants. Biological Reviews, 57(1), 59–84. [Google Scholar]
93. Kolbert, Z., Molnár, Á., Feigl, G., Van Hoewyk, D. (2019). Plant selenium toxicity: Proteome in the crosshairs. Journal of Plant Physiology, 232, 291–300. [Google Scholar] [PubMed]
94. McNeal, J. M., Balistrieri, L. S. (1989). Geochemistry and occurrence of selenium: An overview. Selenium in Agriculture and the Environment, 23, 1–13. https://doi.org/10.2136/sssaspecpub23 [Google Scholar] [CrossRef]
95. Kumar, A., Prasad, K. S. (2021). Role of nano-selenium in health and environment. Journal of Biotechnology, 325, 152–163. [Google Scholar] [PubMed]
96. Reynolds, R. J. B., Jones, R. R., Heiner, J., Crane, K. M., Pilon‐Smits, E. A. H. (2020). Effects of selenium hyperaccumulators on soil selenium distribution and vegetation properties. American Journal of Botany, 107(7), 970–982. [Google Scholar] [PubMed]
97. Schomburg, L., Arnér, E. S. (2017). Selenium metabolism in herbivores and higher trophic levels including mammals. In: Selenium in plants, pp. 123–139. Springer. https://doi.org/10.1007/978-3-319-56249-0_8 [Google Scholar] [CrossRef]
98. Tamaoki, M. (2008). The role of phytohormone signaling in ozone-induced cell death in plants. Plant Signaling & Behavior, 3(3), 166–174. [Google Scholar]
99. Li, S., Liu, C. (2022). Use of selenium accumulators and hyperaccumulators in Se-phytoremediation technologies: Recent progress and future perspectives. Selenium and Nano-Selenium in Environmental Stress Management and Crop Quality Improvement, 365–381. https://doi.org/10.1007/978-3-031-07063-1_18 [Google Scholar] [CrossRef]
100. Schiavon, M., Santoro, V. (2022). Manipulation of selenium metabolism in plants for tolerance and accumulation. In: Selenium and nano-selenium in environmental stress management and crop quality improvement, pp. 325–340. Springer. https://doi.org/10.1007/978-3-031-07063-1_16 [Google Scholar] [CrossRef]
101. Wu, Z., Zhang, X., Zhang, N., Gao, X., Feng, X. et al. (2022). Identification of ACC synthetase genes in saccharum and their expression profiles during plant growth and in response to low-nitrogen stress. Tropical Plant Biology, 15, 1–14. [Google Scholar]
102. Hasan, M., Hossain, M. A., Burritt, D. J., Ashrafuzzaman, M. (2022). Cereals and phytohormones under ozone stress. In: Sustainable remedies for abiotic stress in cereals, pp. 443–467. Springer. https://doi.org/10.1007/978-981-19-5121-3_18 [Google Scholar] [CrossRef]
103. Mamenko, T., Kots, S. (2022). Stress-induced ethylene production by soybean symbiotic systems depending on genetic characterization of Bradyrhizobium strains. Modern Phytomorphology, 16(2), 28–31. [Google Scholar]
104. Yi, R., Yan, J., Xie, D. (2020). Light promotes jasmonate biosynthesis to regulate photomorphogenesis in Arabidopsis. Science China Life Sciences, 63(7), 943–952. [Google Scholar] [PubMed]
105. Szőllősi, R., Molnár, Á., Oláh, D., Kondak, S., Kolbert, Z. (2022). Selenium toxicity and tolerance in plants: Recent progress and future perspectives. In: Selenium and nano-selenium in environmental stress management and crop quality improvement, pp. 311–324. https://doi.org/10.1007/978-3-031-07063-1_15 [Google Scholar] [CrossRef]
106. Hasan, M. M., Rahman, M. A., Skalicky, M., Alabdallah, N. M., Waseem, M. et al. (2021). Ozone induced stomatal regulations, MAPK and phytohormone signaling in plants. International Journal of Molecular Sciences, 22(12), 6304. [Google Scholar] [PubMed]
107. Shiragaki, K., Iizuka, T., Ichitani, K., Kuboyama, T., Morikawa, T. et al. (2019). HWA1- and HWA2-mediated hybrid weakness in rice involves cell death, reactive oxygen species accumulation, and disease resistance-related gene upregulation. Plants, 8(11), 450. [Google Scholar] [PubMed]
108. Huang, Q., Xu, R., Zhang, Y., Yan, Z., Chen, H. et al. (2022). Salicylic acid ameliorates cadmium toxicity by increasing nutrients uptake and upregulating antioxidant enzyme activity and uptake/transport-related genes in Oryza sativa L. indica. Journal of Plant Growth Regulation, 42, 1–13. [Google Scholar]
109. Tamaoki, M. (2008). The role of phytohormone signaling in ozone-induced cell death in plants. Plant Signaling & Behavior, 3(3), 166–174. [Google Scholar]
110. Ding, Y., Dommel, M. R., Wang, C., Li, Q., Zhao, Q. et al. (2020). Differential quantitative requirements for NPR1 between basal immunity and systemic acquired resistance in Arabidopsis thaliana. Frontiers in Plant Science, 11, 570422. [Google Scholar] [PubMed]
111. Lorenzo, O., Solano, R. (2005). Molecular players regulating the jasmonate signalling network. Current Opinion in Plant Biology, 8(5), 532–540. https://doi.org/10.1016/j.pbi.2005.07.003 [Google Scholar] [PubMed] [CrossRef]
112. Liu, Y., Li, Y. (2022). MPK4 negatively regulates the l-arabinose synthesis of cell wall in Arabidopsis. Biochemical and Biophysical Research Communications, 613, 7–11. [Google Scholar] [PubMed]
113. Babarabie, M., Zarei, H., Badeli, S. (2018). Morphological, physiological and biochemical response of Chrysanthemum to thiamine and salicylic acid. Journal of Plant Physiology and Breeding, 8(2), 109–120. [Google Scholar]
114. Schütz, V., Frindte, K., Cui, J., Zhang, P., Hacquard, S. et al. (2021). Differential impact of plant secondary metabolites on the soil microbiota. Frontiers in Microbiology, 12, 1267. [Google Scholar]
115. Jain, S., Jain, J., Singh, J. (2020). The rhizosphere microbiome: Microbial communities and plant health. In: Plant microbiome paradigm, pp. 175–190. Springer. https://doi.org/10.1007/978-3-030-50395-6_10 [Google Scholar] [CrossRef]
116. Isah, T. (2019). Stress and defense responses in plant secondary metabolites production. Biological Research, 52(1), E762. [Google Scholar]
117. Bhatla, S. C., Lal, M. A. (2018). Plant physiology, development and metabolism. Springer. https://doi.org/10.1007/978-981-13-2023-1 [Google Scholar] [CrossRef]
118. White, P. J. (2018). Selenium metabolism in plants. Biochimica et Biophysica Acta (BBA)-General Subjects, 1862(11), 2333–2342. [Google Scholar] [PubMed]
119. Pivato, M., Fabrega-Prats, M., Masi, A. (2014). Low-molecular-weight thiols in plants: Functional and analytical implications. Archives of Biochemistry and Biophysics, 560(10), 83–99. [Google Scholar] [PubMed]
120. González-Morales, S., Pérez-Labrada, F., García-Enciso, E. L., Leija-Martínez, P., Medrano-Macías, J. et al. (2017). Selenium and sulfur to produce Allium functional crops. Molecules, 22(4), 558. [Google Scholar]
121. Matich, A. J., McKenzie, M. J., Lill, R. E., McGhie, T. K., Chen, R. K. Y. et al. (2015). Distribution of selenoglucosinolates and their metabolites in Brassica treated with sodium selenate. Journal of Agricultural and Food Chemistry, 63(7), 1896–1905. [Google Scholar] [PubMed]
122. Wiesner-Reinhold, M., Schreiner, M., Baldermann, S., Schwarz, D., Hanschen, F. S. et al. (2017). Mechanisms of selenium enrichment and measurement in brassicaceous vegetables, and their application to human health. Frontiers in Plant Science, 8, 1365. [Google Scholar] [PubMed]
123. Ouerdane, L., Aureli, F., Flis, P., Bierla, K., Preud’Homme, H. et al. (2013). Comprehensive speciation of low-molecular weight selenium metabolites in mustard seeds using HPLC–electrospray linear trap/orbitrap tandem mass spectrometry. Metallomics, 5(9), 1294–1304. [Google Scholar] [PubMed]
124. Handa, N., Kohli, S. K., Sharma, A., Thukral, A. K., Bhardwaj, R. et al. (2018). Selenium ameliorates chromium toxicity through modifications in pigment system, antioxidative capacity, osmotic system, and metal chelators in Brassica juncea seedlings. South African Journal of Botany, 119, 1–10. [Google Scholar]
125. da Silveira, E. L., Semmar, N., Cartes, J. E., Tuset, V. M., Lombarte, A. et al. (2020). Methods for trophic ecology assessment in fishes: A critical review of stomach analyses. Reviews in Fisheries Science & Aquaculture, 28(1), 71–106. [Google Scholar]
126. Kahromi, S., Khara, J. (2021). Chitosan stimulates secondary metabolite production and nutrient uptake in medicinal plant Dracocephalum kotschyi. Journal of the Science of Food and Agriculture, 101(9), 3898–3907. [Google Scholar] [PubMed]
127. Verma, V., Ravindran, P., Kumar, P. P. (2016). Plant hormone-mediated regulation of stress responses. BMC Plant Biology, 16(1), 1–10. [Google Scholar]
128. Maassoumi, A. A., Ashouri, P. (2022). The hotspots and conservation gaps of the mega genus Astragalus (Fabaceae) in the Old-World. Biodiversity and Conservation, 31, 1–21. [Google Scholar]
Cite This Article
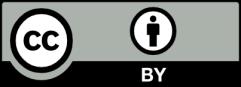