Open Access
REVIEW
Molecular basis of COVID-19, ARDS and COVID-19-associated ARDS: Diagnosis pathogenesis and therapeutic strategies
1 Biopharmaceutical Research Lab, Anusandhan Kendra-1, SASTRA Deemed-to-be University, Thanjavur, 613401, India
2 School of Chemical and Biotechnology, SASTRA Deemed-to-be University, Thanjavur, 613401, India
* Corresponding Author: SENTHIL VISAGA AMBI. Email:
(This article belongs to the Special Issue: The aberrant signaling cascade transduction during disease development )
BIOCELL 2023, 47(11), 2335-2350. https://doi.org/10.32604/biocell.2023.029379
Received 15 February 2023; Accepted 25 August 2023; Issue published 27 November 2023
Abstract
The novel coronavirus pneumonia (COVID-19) is spreading worldwide and threatening people greatly. The routes by which SARS-CoV-2 causes lung injury have grown to be a major concern in the scientific community since patients with new Coronavirus, severe acute respiratory syndrome coronavirus (SARS-CoV-2) have a high likelihood of developing acute respiratory distress syndrome (ARDS) in severe cases. The mortality rate of COVID-19 has increased over the period due to rapid spread, and it becomes crucial to understand the disease epidemiology, pathogenic mechanisms, and suitable treatment strategies. ARDS is a respiratory disorder and is one of the clinical manifestations observed in patients with severe COVID-19. In this scenario, it is important to address this problem to develop suitable treatment strategies. This review attempts to present the prevalence of ARDS in COVID-19 patients and their predictive causes and risk factors, highlighting the contrasting features of COVID-19-induced ARDS with typical ARDS. This review also presents insights into the association between SARS-CoV-2 and lung damage while exploring the potential COVID-19 mechanisms in ARDS from the perspective of the angiotensin-converting enzyme 2 protein, cytokine storm, immune responses, and other signaling pathways. The review also discusses the diagnosis strategies, pathogenesis, risk factors, and treatment options of COVID-19-related ARDS.Graphical Abstract
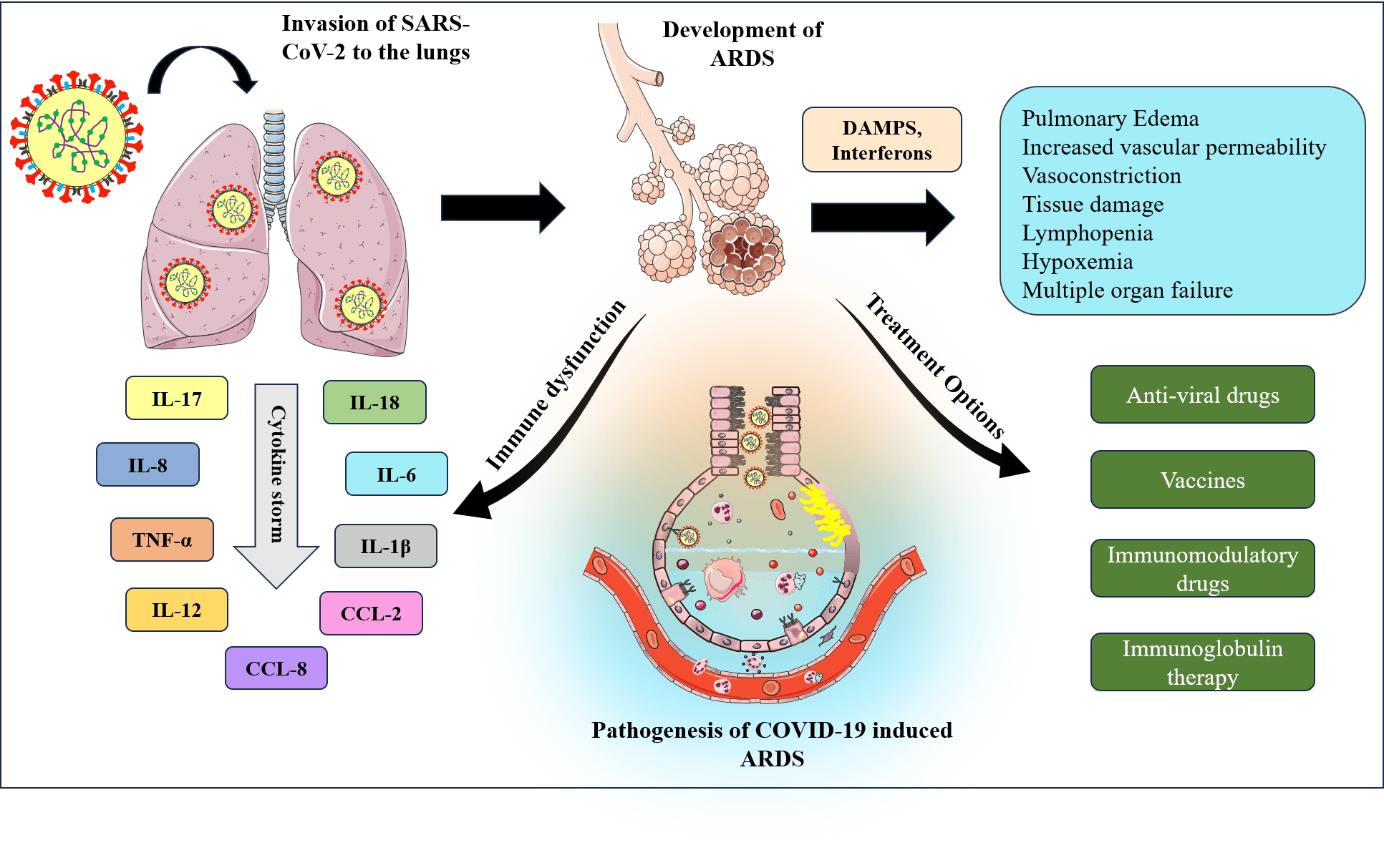
Keywords
Coronavirus disease of 2019 (COVID-19) is a highly contagious respiratory disease caused by Severe Acute Respiratory Syndrome Coronavirus-2 (SARS-CoV-2) which was first reported in Wuhan, China, in late December 2019. COVID-19 has swiftly spread across the globe and has become a global pandemic in a short period. Patients affected by COVID-19 develop symptoms during the incubation period of 1–14 days (Hu et al., 2021). The most common symptoms of COVID-19 include fever, dry cough, and other manifestations like fatigue and headache (Pullen et al., 2020). Several investigations have shown that older persons aged 60 years and above are especially prone to infection. The condition becomes critical when patients are found to have major comorbidities such as hypertension (30.7%), diabetes (14.3%), cardiovascular disease (11.9%), and respiratory diseases (Hu et al., 2021; Siordia, 2020). The highly infectious and fatal nature of COVID-19 led to the development of different vaccines. In addition, a few conventional anti-viral drugs and protease inhibitors were found to be effective against SARS-CoV-2
Most of the patients admitted to the hospital with COVID-19 infection had developed pneumonia, followed by Acute respiratory distress syndrome (ARDS) (Sanyaolu et al., 2020). ARDS is a life-threatening condition caused by the accumulation of fluid in the lungs air sacs. Patients often have trouble in breathing and require ventilator support for their survival. The most noticeable characteristics of ARDS are diffuse alveolar injury and pulmonary edema (Matthay et al., 2018). Despite significant progress in understanding the pathogenic mechanisms of ARDS, only a few pharmaceutical treatments are effective. The most common treatment for ARDS is mechanical ventilation. ARDS, in most of the severe COVID-19 patients, has been identified as disease primarily affecting the lungs. The occurrence of ARDS in COVID-19 patients increases mortality (Wu et al., 2020). The primary objective of this review is to identify the prevalence of ARDS in patients with COVID-19 and briefly understand their cause and risk factors, followed by the impact of current COVID-19 vaccines with respect to ARDS. The review also summarizes the epidemiology, pathogenesis, and current treatment strategies of ARDS and COVID-19. We have also tried to present the effect of different SARS-CoV-2 variants in developing ARDS.
SARS-CoV-2, the cause of COVID-19 infection, is known to replicate mostly in the lower respiratory tract. Due to human-to-human transmission, the virus has quickly spread to various parts of the world, with a high rate of transmission. When the infected person coughs or sneezes, fluid particles from their mouth or nose can spread to other people in the vicinity. To avoid contracting viruses, people are urged to practice preventive measures such as masks, social distancing, and adequate sanitization. Reverse transcription-polymerase chain reaction (RT-PCR) assays can be used to detect COVID-19 infections in patients (World Health Organization, 2023). However, the relentless research in the development of different vaccines have paved the way for reduced transmission and death. In this review, we discuss the epidemiology and structural characteristics of SARS-CoV-2 virus followed by their pathogenesis and treatment strategies currently followed.
The incidence of a pandemic caused by a coronavirus is not totally new to the world, since coronaviruses such as SARS-CoV and the Middle East Respiratory Syndrome Coronavirus (MERS-CoV) had emerged in the past. Scientists hypothesized that like SARS-CoV and MERS-CoV, SARS-CoV-2 may have evolved from bats because of its similarities to bat coronaviruses (Ganesh et al., 2021; Kuba et al., 2021). The first incidence of SARS-CoV epidemic was reported in China in the year 2002. A total of 8098 people were affected, with a death rate of 9.56%. The second outbreak, caused by MERS-CoV, began in Saudi Arabia in 2012 and affected 2279 persons worldwide, with Saudi Arabia reporting a high mortality rate (38.5%) (Ganesh et al., 2021). Next-generation sequencing of bronchoalveolar lavage fluid (BALF) samples collected from a patient infected by SARS-CoV-2 revealed a new kind of beta coronavirus (Zhou et al., 2020). Within a short period, the virus has rapidly spread across different cities of China through human-to-human transmission. The first case of SARS-CoV-2 outside China was reported in Thailand on 13 January 2020 followed by Japan and the USA, while India reported its first case on 30 January 2020 (Dhar Chowdhury and Oommen, 2020).
In January 2020, the World Health Organization (WHO) initially termed this new infection as 2019-Novel Coronavirus (2019-nCoV). Following that, the International Committee on Taxonomy of Viruses named the novel coronavirus as SARS-CoV-2, and WHO termed the disease as COVID-19 on February 11, 2020. SARS-CoV-2 spread fast around the globe in less than a month, prompting the WHO to declare it a global pandemic (Hu et al., 2021). Patients under the age of 18 years are more likely to be asymptomatic, increasing the risk of infection and transmission (Siordia, 2020). As of today (September 18 2023), Coronavirus has affected 770 million individuals, resulting in 6.9 million deaths. The United States of America has the most COVID-19 incidences with 103 million, followed by China with 99 million (World Health Organization, 2023).
SARS-CoV-2 is a beta coronavirus that has a crown-like appearance due to spikes when viewed under the electron microscope. It belongs to the Orthocoronavirinae subfamily of the Coronaviridae family, which includes four genus classifications: alpha, beta, delta, and gamma, with only alpha and beta being infectious to humans (Samudrala et al., 2020). SARS-CoV-2 is a positive-sensed single-strand RNA virus with four components: nucleocapsid protein (N), envelope protein (E), membrane protein (M), and spike proteins (S1, S2). Fig. 1 illustrates the typical structure of SARS-CoV-2. Nucleocapsid protein aids in the transcription of viruses and their assembly into host cells, whereas envelope protein, the smallest transmembrane protein, promotes in viral proliferation. Membrane protein helps in the entry of the virus into host cells, inhibits host cell genes and facilitates virus replication (Xia et al., 2021). Among all these proteins, spike protein is the most significant because it binds the virus to host cell receptor. Spike protein has two subunits, S1 and S2; S1 contains an N-terminal domain (NTD) and receptor binding domain (RBD), and the S2 subunit contains a fusion peptide, heptapeptide repeat sequence 1,2 (HR1, HR2), TM domain, and C-terminal domain (Huang et al., 2020). SARS-CoV-2 is highly prone to genetic mutations where nearly five different variants of the virus have been identified as ‘variants of concern’. WHO has named these variants with Greek letter alphabets like alpha, beta, gamma, delta, epsilon, Mu, and omicron (World Health Organization, 2023). Currently, Omicron variant has been identified to be highly virulent and it mostly affects the younger population and is less susceptible to vaccines (Firouzabadi et al., 2023).
Figure 1: Structure of SARS-CoV-2.
Pathogenesis and pathophysiology
The spike protein of SARS-CoV-2 plays a significant role in the entry of the virus through the human angiotensin converting enzyme-2 (hACE2) receptor into the host cell, and the pathogenesis is illustrated in Fig. 2. Initially, the RBD of the S1 subunit of spike protein binds to the hACE2 receptor (Huang et al., 2020). Researchers have found that the host cell proteases cleave the specific furin site between the S1 and S2 subunits, which ultimately facilitates the fusion of the virus with the host cell. Subsequently, the virus is internalized via endocytosis (Ou et al., 2020). After endosome formation, the virus releases viral RNA into the cytoplasm through cathepsin L-mediated process, where viral RNA is replicated and translated into new viral proteins (Hu et al., 2021). SARS-CoV-2 genomic RNA is reported to encode 28 proteins, including structural, non-structural, and accessory proteins (Pullen et al., 2020). Finally, newly formed viral particles are produced in the host cell and released into the circulation by exocytosis (Yuki et al., 2020). COVID-19 is usually transmitted via respiratory droplets from the coughing and sneezing of infected patients. The high transmission efficiency of COVID-19 is because of the 10%–20% higher binding capacity of SARS-CoV-2 spike protein to the hACE2 receptor (Ganesh et al., 2021). The binding of SARS-CoV-2 to the hACE2 receptor inhibits the binding of angiotensin (Ang-II). This competitive binding modulates renin-angiotensin-aldosterone-system (RAAS) signaling pathway (Beyerstedt et al., 2020; Kuba et al., 2021). The RAAS pathway regulates vasoconstriction and vasodilation. Renin converts angiotensinogen into Ang-I and angiotensin converting enzyme (ACE) converts Ang-I to Ang-II. Ang-II has deleterious effects like vasoconstriction, eventually it gets converted into Ang (1-7) by ACE2. If SARS-CoV-2 binds to the hACE2 receptor, it will downregulate the expression of ACE2 and eventually will lead to more amount of Ang-II presence in cells. Conclusively, it leads to oxidative stress and inflammation (Beyerstedt et al., 2020).
Figure 2: Pathogenesis of SARS-CoV-2.
When the person contracts the SARS-CoV-2, the inhaled virus binds to the epithelial cells of the nasal cavity and replicates. Then, the virus migrates to the conducting airways of the respiratory tract, which is defined as the mild stage of the infection. Some patients progress to the severe stage where the virus affects the type II alveolar epithelial cells (Mason, 2020). Most of the patients experience fever, dry cough and headache during infection and some patients have symptoms like loss of smell and taste (hyposmia, hypogeusia) (Singh et al., 2021). SARS-CoV-2 mainly affects the lungs which cause interstitial pneumonia, diffuse alveolar damage (DAD), lymphocytic inflammation, and pulmonary edema. Apart from lungs, it also affects other organs and causes multiple organ dysfunction in 2%–20% of patients who require mechanical ventilation (Tabary et al., 2020).
The United States Food and Drug Administration (FDA) and WHO have approved a wide range of drugs and vaccines. Corticosteroids have proven good therapeutic efficacy against ARDS; therefore, they are used to treat COVID-19. Corticosteroids decrease inflammation by binding to glucocorticoid and mineralocorticoid receptors. Dexamethasone has shown a promising anti-inflammatory effect, reduces the need for mechanical ventilation, and decreases mortality. Further, dexamethasone is now advised as a conventional treatment at a dosage of 6 mg per day for 10 days (Taher et al., 2023). Other corticosteroids such as hydrocortisone, methylprednisolone, and prednisone have also been used to treat COVID-19 infections, but only when a conventional medication is not available (Johns et al., 2021; Raju et al., 2021). Prior to the COVID-19 pandemic, vaccines for coronaviruses were not proven to be effective since the MERS vaccine had only completed phase 1 clinical trials (Modjarrad et al., 2019). The deadly nature of COVID-19 infections necessitates the development of vaccines, and a list of widely used vaccines is provided in Table 1 (Venkadapathi et al., 2021).
Four different kinds of vaccines have been developed until now: mRNA vaccine, adenoviral vector vaccine, inactivated whole virus vaccine, and subunit vaccine. In the case of the mRNA vaccine, mRNA of viral antigen, primarily protein present on its surface, is injected to elicit an immune response. Following this principle, two mRNA vaccines, like the Pfizer-BioNTech and Moderna vaccines, were approved. The benefit of employing the mRNA vaccine is that the sequence of mRNA can be altered, which can act effectively against different variants (Forchette et al., 2021). A few drawbacks of mRNA vaccines include RNA degradation, stability, and transport difficulties, but they can be resolved by using lipid nanoparticles (LNP) (Heinz and Stiasny, 2021). However, LNP encapsulation for mRNA vaccines will be stable under refrigerated temperatures only for 5–30 days (Forchette et al., 2021).
Adenovirus vaccines like Sputnik V and Johnson and Johnson use DNA that encodes viral spike protein and uses an adenovirus vector for delivery of antigen instead of LNP. China has developed two inactivated vaccines, including the Sinopharm BIBP vaccine and Sinovac-Coronavac, where the virus is inactivated using heat, radiation, and chemical processes, producing an immune response in the body without causing infection (Firouzabadi et al., 2023; Venkadapathi et al., 2021). Currently, in India, two vaccines are predominantly used: Covaxin and Covisheild (ChAdOx1 nCoV-19) (Kaushik et al., 2021). Covaxin was developed by the Indian company Bharat Biotech and approved by India for emergency use on 03 January, 2021. It is an inactivated whole virus vaccine that is chemically inactivated by β-propiolactone (Heinz and Stiasny, 2021). Covisheild is an adenoviral vector vaccine developed by the University of Oxford and AstraZeneca, UK. Phase 2 clinical study has reported that the Covisheild vaccine induced a strong immune response with less reactogenicity (Folegatti et al., 2020). As of today (18 September, 2023), 70% of the total world population has taken at least one dose of the COVID vaccine (Our World in Data, 2023)
Remdesivir is one of the most prescribed anti-viral drugs which has shown effectiveness against SARS, MERS, as well SARS-CoV-2. It reduces the SARS-CoV-2 infection by inhibiting the action of the viral replication enzyme. Plitidepsin is also an anti-viral drug that has shown better results compared to remdesivir in inhibiting COVID-19 by targeting translation factors (Forchette et al., 2021). Favipiravir has shown effectiveness against the Influenza virus and is also effective against the SARS-CoV-2 virus by inhibiting RNA polymerase enzyme. Umifenovir reduces the effect of SARS-CoV-2 by inhibiting the fusion of the virus with the host cell (Wang et al., 2021).
The combinational drug lopinavir/ritonavir, which is a protease inhibitor, has shown good results as it stalls the replication of the virus (Venkadapathi et al., 2021). Monoclonal antibodies have also been used for the treatment of COVID-19. Bevacizumab has been shown to alleviate pulmonary edema by inhibiting vascular endothelial growth factor (VEGF) (Hu et al., 2021). Tocilizumab and Sarilumab suppress the expression of interleukin 6 (IL-6), an inflammatory cytokine that is abundantly expressed in patients with COVID-19. Lenzilumab inhibits the expression of other inflammatory cytokines, namely granulocyte macrophage colony-stimulating factor, and eventually reduces the severity of the disease (Venkadapathi et al., 2021). Passive immunotherapy is also considered an effective therapy against COVID-19, and was followed in India. In this therapy, plasma collected from COVID-19-recovered patients is administered to affected individuals. However, although this passive immunotherapy reduced viral particles in patients, it had adverse side effects such as fever and anaphylactic reactions (Wang et al., 2021).
Acute Respiratory Distress Syndrome
ARDS is an inflammatory disorder with the characteristic features of diffuse alveolar damage (DAD), followed by non-cardiogenic pulmonary edema, and increased alveolar permeability (Kaku et al., 2020). Currently, the Berlin definition of ARDS is being followed, which categorizes ARDS as mild, moderate, or severe. The mortality rate of severe ARDS is still as high as 45% of ARDS-affected patients (Ranieri et al., 2012; Wilson and Calfee, 2020). The lungs are primarily engaged in the process of gas exchange, and patients with ARDS have a large dead space fraction, which is associated with greater mortality (Rezoagli et al., 2017). Patients with significant comorbidities are at higher risk than normal people, and numerous cases have also been reported in children. Even after recovering from ARDS, few patients experience muscle weakness, depression, or post-traumatic stress disorder (Dushianthan et al., 2011). Despite the high mortality, only a few therapies are effective due to the wide variety of ARDS phenotypes (Wilson and Calfee, 2020) and the response to treatment varies depending on the phenotypes.
Evolution of acute respiratory distress syndrome and epidemiology
In 1821, ARDS was described as idiopathic pulmonary edema, and after a century, they termed the condition as ARDS in 1967. In 1987, few scientists defined ARDS in the expanded version as ‘the Murray Lung Injury score’ and categorized ARDS into three different categories based on scores (Murray et al., 1988). A milder form of ARDS is called Acute lung injury (ALI) (Rezoagli et al., 2017). The American-European consensus conference was held in 1992 and they classified ALI and ARDS based on the PaO2/FiO2 (PF) ratio and the definition of ARDS outlined in Table 2 (Bernard et al., 1992). Over the years, the American European Consensus Conference (AECC) definition was widely accepted, but it has a few limitations. In 2012, the Berlin definition for ARDS was formulated to address the limitations of AECC, and mortality has been predicted for mild, moderate, and severe as 27%, 32%, and 45%, respectively. The term Acute lung injury has been removed from Berlin’s definition as it confuses clinicians when diagnosing patients, and hydrostatic edema was included as one of the criteria (Ranieri et al., 2012). Even the Berlin definition has certain drawbacks, in that it cannot be applied in a real-time world scenario where there is a limited number of resources for diagnosis. In addition, etiology factors have not been included.
In 2016, a study with 1046 patients was undertaken in Kigali, and a few alterations to the Berlin criteria were made, including the use of ultrasound, which has shown to be more accurate than chest radiography. In this study, the Spo2/FiO2 ratio 315 was employed to measure oxygenation instead of the PF ratio. Of 1046 patients, 4% fall under Kigali-modified ARDS criteria, and no patient has been classified as ARDS with the Berlin definition. Kigali-modified criteria needs further validation for utilizing it as a primary diagnostic tool (Riviello et al., 2016). ARDS has also been reported in children aged 2 to 17 years, with an incidence of 2.2–5.7 per 100,000 person-years and a death rate of 17%–33% compared to that in adults (Matthay et al., 2018). Recognition of ARDS is the primary factor that determines the survival of patients. Clinicians failed to recognize over 40% of all instances (Bellani et al., 2016). ARDS occurs in probably 10%–15% of patients admitted to the ICU, and among them, 23% of patients need mechanical ventilation. According to a few studies, men are more prone to develop ARDS, but women have a greater fatality rate (Meyer et al., 2021).
The impairment of alveolar-capillary permeability and alveolar fluid clearance processes are the main causes of ARDS. In healthy lungs, the alveolar capillary membrane, commonly known as the “blood-air barrier”, is made up of endothelial cells, alveolar epithelial cells (Types I and II), alveolar macrophages, and extracellular matrix. Alveolar epithelial type I cells (ATI) are thin, flat cells that primarily regulate gas exchange (CO2, O2) between the alveolar unit and blood capillaries, as well as producing pro-inflammatory cytokines in response to infection. Alveolar epithelial type II cells (AT-II) are small, cuboidal and involved in the secretion of surfactant, which acts as a local defense system and reduces surface tension (Matthay et al., 2018). In response to injury or damage, these AT-II cells repair the tissue by trans-differentiating into AT-I cells. Impairment of endothelial permeability is the main factor in the pathogenesis of ARDS. Besides local defense mechanisms, alveolar macrophages are found within the epithelium. Fibroblast is found between the epithelium and the endothelium, and it aids in lung tissue wound repair (Nova et al., 2019).
In the first step of lung injury, the endothelial cells are activated, which alters endothelial permeability. Endothelial cells are activated by various factors like pathogens (bacteria, viruses), cytokines, and hypoxia (Meyer et al., 2021). The disintegration of vascular endothelial cadherin junctions can result in gaps and increased endothelial permeability (Huppert et al., 2019), which leads to the recruitment of neutrophils and platelets. Activated neutrophils release pro-inflammatory cytokines, chemokines, proteases, and reactive oxygen species, and they also interact with platelets and form aggregates. Although alveolar endothelial injury is not enough to generate ARDS, some degree of epithelial injury is essential for disease progression (Matthay and Zemans, 2011). In alveolar epithelial injury, activated neutrophils migrate to the epithelium via a paracellular route. The migrating neutrophils induce apoptosis of alveolar epithelial cells by releasing inflammatory mediators like matrix metalloproteinases, elastase, and reactive oxygen species (Matthay and Zemans, 2011). Another factor that contributes to the development of ARDS is impaired alveolar fluid clearance. The alveolar epithelial membrane in healthy lungs contains ion channels like the epithelial sodium channel (ENaC), which drains the fluid in the lungs and subsequently by the lymphatic system. This mechanism is disturbed due to multiple factors like hypoxia, the release of pro-inflammatory cytokines (tumor necrosis factor (TNF)-α, IL-8), and biomechanical injury (Huppert et al., 2019). In Fig. 3, the healthy alveolar-capillary membrane and ARDS-affected membrane are compared.
Figure 3: Comparison of healthy and ARDS affected alveolar capillary membrane.
ARDS has been differentiated into three different phases: exudative phase, proliferative phase, and fibrotic phase. After the onset of the disease, the first seven days are known as the exudative phase. Diffuse alveolar damage is a key aspect of the exudative phase, where alveolar epithelial and endothelial cells undergo apoptosis, and mostly AT-I cells degenerate. Histopathological examination of lung tissue revealed the formation of a hyaline membrane containing leaked plasma proteins. Downregulation of ENaC and alveolar fluid clearance results in non-cardiogenic pulmonary edema (Derwall et al., 2018; Tomashefski, 2020). The proliferative phase lasts about 7–14 days and is called the recovery phase, wherein AT-II cells start to proliferate into AT-I cells (Tomashefski, 2020). The fibrotic phase begins after 14 days, and extensive fibrosis and squamous metaplasia are seen (Sharp et al., 2015).
ARDS is caused by a number of factors, including sepsis, trauma, smoking, alcohol consumption, and severe acute pancreatitis. Sepsis is found to be the most common risk factor for the development of ARDS, and mortality was also high for sepsis-induced ARDS. Age and sex were identified as crucial factors, whereby males tend to develop ARDS more than females (TenHoor et al., 2001). Trauma is one of the etiological factors for ARDS, although the incidence declined after 2012 (Tignanelli et al., 2019). In 2018, a study identified the most common risk factors as sepsis, pneumonia, and shock whereas pancreatitis, drowning, and pulmonary contusion as the less common risk factors (Eworuke et al., 2018).
Blood transfusion has previously been identified as a primary cause of ARDS, accounting for about 25%–40% of cases (Eworuke et al., 2018; Kaku et al., 2020); however, its prevalence has decreased since 2009. One study reported aspiration-induced ARDS in 30% of cases and mortality was also very high (Kaku et al., 2020). Recently, a logistic regression analysis-related study identified risk factors for predicting ARDS in severe acute pancreatitis (SAP) patients, including APACHE score, Ranson score, c-reactive protein level, and albumin level (Zhang et al., 2021). A study conducted in 2014 identified that the prevalence of ARDS in diabetic patients has reduced lately (Gu et al., 2014). As previously stated, children can develop ARDS, and parameters such as organ failure during hospitalization, the PRISM score, Pinsp on day 1, and the mean airway pressure gradient are used to predict mortality in children (Panico et al., 2015). Though many influential factors were determined for ARDS, 8.3% of cases had no common risk factor identified (de Prost et al., 2017).
Sub phenotypes of acute respiratory distress syndrome and diagnostic biomarkers Diffuse alveolar damage (DAD) is a principal feature in ARDS, but few studies have reported that only 45% of cases have shown DAD in autopsy findings (Reilly et al., 2019). Treating ARDS is difficult because of varying phenotypes. Latent class analysis was performed to find the sub-phenotypes among the population with ARDS. Statistical analysis identified two phenotypes with different treatment responses, clinical characteristics, and biomarkers. Phenotype 1 is characterized as ‘less inflamed’ (Hypoinflammatory) compared to phenotype 2 (hyperinflammatory). Patients with phenotype 2 have significant organ failures and a higher mortality rate of 51% (Calfee et al., 2014). Researchers recently used transcriptomics to categorize ARDS, identifying two distinct phenotypes: reactive and uninflamed. Compared to uninflamed phenotype, the reactive sub-phenotype exhibits significantly expressed neutrophil-associated genes (Matthay et al., 2018).
Advancement in genomics approaches leads to the discovery of specific biomarkers for different phenotypes in the population with ARDS. Diagnostic biomarkers play a major role in predicting the severity of disease along with treatment outcomes. VEGF, IL-8, RAGE, and TGF-β are prominent factors in lung injury (Sharp et al., 2015). Survivors and non-survivors of ARDS can be determined based on the expression of significant proteins (Bhargava et al., 2017).
Mechanical ventilation is a primary treatment for ARDS to provide a sufficient oxygen supply. However, the treatment itself can cause ventilator-associated lung injury. Considering pharmacological therapies for ARDS, their role has not been efficiently proven in reducing the outcome of ARDS (Dushianthan et al., 2011). Some of the pharmacological therapies are included in Table 3 and can be used as adjuvant therapy for ARDS. ARDS is an inflammatory disorder; hence, corticosteroids are also the treatment of choice. Secondary analysis has been conducted and the authors have not identified any beneficial effects while using low-dose methylprednisolone (Zhang et al., 2015).
Nanobiotechnology has numerous advantages, including improved site targeting, bioavailability, and stability. Strategies like targeting endothelial cells, epithelial cells, macrophages, and neutrophils, have been employed for the treatment of ARDS (Qiao et al., 2021). Lipopolymeric microspheres encapsulating the corticosteroid dexamethasone were recently developed. Dexamethasone-loaded microspheres have shown reduced production of pro-inflammatory cytokines and reduced inflammatory reactions (Kotta et al., 2021).
COVID-19-Induced Acute Respiratory Distress Syndrome
Nearly one in four patients develop ARDS during SARS-CoV-2 infections (Tsai et al., 2022). The prevalence and mortality of COVID-19-induced ARDS are high, and demands the detailed understanding of its pathophysiology, risk factors, and treatment strategies. In addition to COVID-19, several infections lead to the development of ARDS. Here, we tried to compare COVID-19-induced ARDS with other forms of ARDS, and presented the impact of COVID-19 vaccines on ARDS and the effect of different SARS-CoV-2 variants in the development of ARDS.
Prevalence and mortality of acute respiratory distress syndrome due to COVID-19
Diagnosis of ARDS in COVID-19 patients has been followed in two steps. (1) Patients need to be SARS-CoV-2 RT-PCR test positive and (2) patients need to satisfy the Berlin definition (Gibson et al., 2020). A global literature survey conducted in 2020 states that nearly 33% prevalence of ARDS, 26% require intensive care, and 16% undergo mechanical ventilation for their survival (Tzotzos et al., 2020). Several studies have demonstrated that patients over the age of 60 years and those with diabetes and COVID-19 have an increased chance of developing ARDS during SARS-CoV-2 infection. In addition, ARDS was more common in COVID-19-affected men than in women (Azagew, 2023; Gujski et al., 2022).
How does SARS-CoV-2 induce acute respiratory distress syndrome?
ARDS has been observed predominantly in COVID-19 patients. While several theories emphasize the role of coronavirus in causing ARDS, the mechanism by which SARS-CoV-2 causes ARDS is still an active research area. Zheng et al. (2022) proposed different possible mechanisms of coronavirus inducing ARDS. First, excessive pro-inflammatory cytokine release causes a cytokine storm, which is followed by acute lung injury. Second, authors have proposed that the interaction of SARS-CoV-2 with the Fas/FasL signaling pathway promotes the development of ARDS, in which Fas/FasL results in abnormal apoptosis of alveolar epithelial cells and produces pro-inflammatory mediators that damage lung tissue (Zheng et al., 2022). In another study, researchers observed that SARS-CoV-2 ORF3a, M, ORF7a, and N proteins are potent nuclear factor (NF)-κB activators which leads to activation of NF-κB pathway and release of pro-inflammatory cytokines (Su et al., 2021; Zheng et al., 2022). In addition to the role of N protein in causing ARDS, envelope protein of SARS-CoV-2 also promotes the development by upregulating the expression of pro-inflammatory cytokines (Xia et al., 2021). It has been hypothesized that downregulated ACE2 expression and increased Ang II have a role in the progression of ARDS because Ang II itself is a pro-inflammatory cytokine (Hojyo et al., 2020; Zhang et al., 2020). According to another hypothesis, the IL-6-STAT3 signaling is involved in the development of ARDS. Due to the activation of Ang II, soluble IL-6Rα is produced and simultaneously activates JAK/STAT3 and NF-κB through the IL-6 amplifier mechanism (Hojyo et al., 2020). Further studies have revealed that alveolar epithelial cell death caused by necrosis influences the progression of ARDS in patients with COVID-19 through the release of damage-associated molecular patterns (DAMPs). The necrosis of alveolar epithelial cells occurs in early stage of COVID-19 disease, which eventually progresses to alveolar tissue damage and ARDS (Tojo et al., 2023). Besides, several studies have speculated that hyperactivation of neutrophils triggers the formation of neutrophils extracellular traps (NET) that leads to tissue inflammation followed by ARDS. Activated neutrophils release various pro-inflammatory cytokines, including IL-6, IL-8, and TNF-α which damage the vascular endothelial cells (Cesta et al., 2023). Overall, the replicating virus affects the host immune system and recruits the immune cells. Activated immune cells secrete pro-inflammatory cytokines and chemokines and eventually induce the apoptosis of alveolar epithelial cells by secreting TNF-α and interferon (Batah and Fabro, 2021). Fig. 4 depicts the proposed pathogenic process of COVID-19-induced ARDS. In COVID-19-induced ARDS patients, alveolar epithelial cells are more affected than endothelial cells (Li and Ma, 2020).
Figure 4: Pathogenesis of COVID-19 induced ARDS.
Comparison of COVID-19-acute respiratory distress syndrome (ARDS) with other ARDS
As stated by various reports, COVID-19-related ARDS is different from typical ARDS. A few studies have reported the onset period of ARDS in COVID-19 patients as between 8 and 12 days; hence, the Berlin definition of onset is not applicable here (Li and Ma, 2020). In COVID-19-related ARDS patients, respiratory system compliance is relatively high (28%) but the PF ratio is unaffected (Grasselli et al., 2020; Grieco et al., 2020). Regarding lung weight, there were conflicting reports. In a study published in 2020 by Chauvelot and colleagues found increased lung weight in patients with COVID-19-induced ARDS, while no significant difference was reported by Grasselli and Tonetti (Chauvelot et al., 2020; Grasselli et al., 2020).
COVID-19 related ARDS is categorized as mild, mild-moderate, and moderate-severe (Li and Ma, 2020). In another study, researchers compared COVID-19 patients with and without ARDS and found that patients with COVID-19-induced ARDS had higher levels of C-reactive protein, IL-6, and lactate dehydrogenase (Dreher et al., 2020). There were many radiographic differences between patients with ARDS and non-ARDS individuals. As a result, the most common characteristic findings in COVID-19 CT images were glass opacities, vascular enlargement, and bilateral abnormalities, whereas pulmonary edema may also be observed in COVID-19-induced ARDS (Kwee and Kwee, 2020). Numerous viruses lead to the development of ARDS. For example, chest radiological findings of patients infected with SARS-CoV are not so different from those with typical ARDS, which has diffused bilateral infiltrates whereas MERS-CoV has nodular opacities and consolidation (Das et al., 2016; Koo et al., 2018; Sampathkumar et al., 2003).
Numerous studies have compared COVID-19-induced ARDS with Influenza-induced ARDS. The study by (Ding et al., 2020) evaluated the distinct clinical features identified between Influenza ARDS and COVID-19 ARDS. The median time for ARDS development after disease onset is relatively longer (9.5 days) in patients with COVID-19 than in patients with influenza (7 days), but severe ARDS was primarily seen in patients with influenza. Multiple organ dysfunction and septic shock were also identified as the primary causes of death in patients with COVID-19-induced ARDS, whereas refractory hypoxemia was identified as the cause of death in influenza induced ARDS patients (Ding et al., 2020). Furthermore, clinical outcomes of patients with COVID-19-induced ARDS and influenza-induced ARDS were compared. Though 28-day mortality was high in patients with influenza, those with COVID-19-induced ARDS required longer mechanical ventilation (Volkov et al., 2023). Tang et al. (2020) investigated the radiological features of COVID-19 ARDS with influenza-induced ARDS. They observed that 95% of COVID-19 patients exhibited ground glass opacities more than influenza patients, but consolidation was mostly observed in influenza-associated ARDS patients (Tang et al., 2020).
Tracheal aspirate RNA sequencing analysis to compare COVID-19-induced ARDS with other causes of ARDS revealed that COVID-19-induced ARDS is characterized by decreased expression of pro-inflammatory genes and compromised expression of interferon-stimulated genes, as compared to other causes (Sarma et al., 2021). COVID-19-induced ARDS and non-COVID ARDS had different treatment responses. Methylprednisolone treatment is effective in improving oxygenation and reducing C-reactive protein (CRP) levels in patients with COVID-19-induced ARDS compared to patients with non-COVID ARDS (Longobardo et al., 2021).
Risk factors for predicting acute respiratory distress syndrome in COVID-19 patients
Severe illness and mortality have been observed in patients with COVID-19-induced ARDS. Several studies have started focusing on identifying risk factors for the development of ARDS in COVID-19 patients. Xu et al. (2021) have utilized machine learning technology to uncover 19 major risk factors that could predict ARDS. In addition, they present clinical features that can be highly observed in patients with COVID-19-induced ARDS, such as coughing, dyspnea, radiological findings like ground-glass opacities, consolidation, and comorbidities like hypertension and diabetes (Xu et al., 2021). A meta-analysis performed to identify the key risk factors in which patients aged 41–64 years mostly progressed to ARDS after COVID-19 infection. Researchers also reported that prolonged mechanical ventilation with therapy, multilobe involvement in the chest, lymphopenia, fever, are the most significant risk factors for the development of ARDS (Tsai et al., 2022). In another study, authors performed multiple logistic regression analyses and identified older age, pulmonary infiltration, and higher CRP levels as risk factors. They devised a 0–3 scoring system to predict the onset of ARDS, including age, CRP level, and lung infiltration as significant indicators (Seo et al., 2021). Wu and co-researchers identified a few factors that determine death in COVID-19-induced ARDS patients, such as older age, neutrophilia, higher LDH levels, and D-dimer. They also observed that methylprednisolone treatment is beneficial in COVID-19 patients (Wu et al., 2020). Anti-viral therapy using lopinavir-ritonavir has no effect in reducing the mortality of patients with COVID-19-induced ARDS, whereas they have predicted the mortality by higher leukocyte count (Zhang et al., 2020).
Apart from the fact that various risk factors influence the severity of ARDS in COVID-19 patients, different phenotypes of ARDS make it challenging for clinicians to provide effective treatment options (Lascarrou, 2021). As previously discussed in the ARDS chapter about the distinct phenotypes (hyper/hypo inflammatory), study has been conducted to understand the prevalence of these phenotypes among patients with COVID-19-induced ARDS. The prevalence of the hyperinflammatory phenotype is very low, although interestingly, increased mortality in hypo inflammatory phenotype is unlikely in a typical ARDS population (Sinha et al., 2020). Distinct phenotypes have also been specifically identified in COVID-19-induced ARDS. Although the class 2 phenotype is less common, it has a higher death rate (40%) than the class 1 phenotype (23%). They differentiated these two phenotypes by increased biomarker D-dimers (Ranjeva et al., 2021). In another report, authors differentiated phenotypes based on lung elastance, lung weight, and lung recruitability. The type L phenotype is characterized by low lung weight, whereas type H has comparatively high lung elastance and lung weight that is similar to the typical severe ARDS category (Gattinoni et al., 2020; Marini et al., 2003).
Treatments for COVID-19-induced-acute respiratory distress syndrome
Currently, researchers are highly keen on developing efficient pharmacological treatments for COVID-19 due to its infectious nature. In addition to COVID-19 clinical manifestations, most of the patients develop ARDS pathologies. So far, no specific standard treatment has been designed for COVID-19-induced ARDS. The use of corticosteroids is considered a primary treatment option to treat ARDS. In May 2020, a small study was conducted to observe the efficacy of methylprednisolone, where the treatment improved oxygenation and reduced inflammatory markers (Kolilekas et al., 2020). Furthermore, patients were given high doses of corticosteroids at an early stage. The duration of hospitalization and mechanical ventilation could be reduced as a result of treatment, which led to a much lower death rate (Boglione et al., 2021). In contrast, a retrospective cohort study conducted by Hung et al. (2022) reported that a higher dose of methylprednisolone did not give any better outcome in COVID-19 induced ARDS patients (Hung et al., 2022). Recently, a prospective controlled clinical trial including 106 patients with COVID-19-induced ARDS compared the efficacy of different corticosteroids, namely dexamethasone, methylprednisolone, and hydrocortisone. Dexamethasone had better clinical outcomes compared to other two corticosteroids in terms of better clinical status on day-28 (Taher et al., 2023). Besides, inhaled nitric oxide showed good results in ARDS, in which enhanced oxygenation was observed (Lotz et al., 2021).
Ruxolitinib, a Janus kinase inhibitor, has anti-inflammatory properties. In sixteen coronavirus-affected patients treated with ruxolitinib, the survival rate improved with decreased IL-6 and CRP levels (Neubauer et al., 2021). Many different treatment options have been investigated for COVID-19-induced ARDS patients, and a low-dose radiation therapy has been proposed. An optimum low therapeutic dose of radiation induces anti-inflammatory effects by polarizing macrophages to an anti-inflammatory phenotype (Dhawan et al., 2020). Additionally, exogenous surfactants have been used to treat ARDS in COVID-19 patients because type 2 alveolar epithelial cells were severely impaired in these patients. In one report, exogenous surfactants was administered to a 48-year-old patient who showed significant clinical improvement following treatment (Heching et al., 2021). Another experimental therapy that showed good efficacy in hospitals was the combinational treatment of tocilizumab and ivermectin. After treatment, hyper-inflammatory markers were reduced, and the patient was discharged (Chuang et al., 2021). Lung transplantation may be a good choice in patients with severe COVID-19-induced ARDS because individuals have exhibited better outcomes. However, this result cannot be taken into account because only 11 patients participated in the study, making it difficult to extrapolate the findings to a larger population (Ko et al., 2022).
Impact of COVID-19 vaccines in acute respiratory distress syndrome
In this current scenario, most people have been vaccinated against COVID-19. Understanding the influence of COVID-19 vaccinations on ARDS is critical. Fortunately, primary vaccination against SARS-CoV-2 infection prevents the development of ARDS and death in patients hospitalized after COVID-19 infection (Robalo et al., 2023). Similarly, in-hospital mortality could also been reduced in mechanically ventilated COVID-19 induced ARDS patients after the full vaccination (Siempos et al., 2022). In contrast, in one report, a patient developing ARDS after receiving a second dose of the COVID-19 vaccination. Here, the RT-PCR result of the patient was negative, yet IgG levels were elevated, and ground-glass opacities and diffuse bilateral infiltrates were seen on chest radiography (Sahin Tutak et al., 2021). In another case, the patient developed interstitial lung disease after vaccination for COVID-19. The CT scans of the patient revealed bilateral diffuse ground-glass opacities, which is a characteristic of ARDS. The mechanisms that cause ARDS to develop after vaccination are relatively unknown, the alveolar endothelial cells may have undergone cytotoxic injury (DeDent and Farrand, 2022). Another theory is that post-vaccination, the viral protein present in the mRNA vaccine is translated and triggers an inflammatory cascade in the lungs as a result of the immune response (Yoshimura et al., 2020). These occurrences have also been seen in people who have received the influenza vaccine. A 53-year-old woman received the influenza vaccine and developed ARDS afterward (Firstenberg et al., 2011). These inconsistencies necessitate in-depth investigation into the association between vaccination and ARDS development.
Effect of different SARS-CoV-2 variants in the development of acute respiratory distress syndrome
As mentioned earlier, SARS-CoV-2 has undergone several mutations during these three years. Several SARS-CoV-2 variants, including alpha, beta, gamma, delta, and omicron, have caused catastrophic damage in various countries. The alpha variant (B.1.1.7), which can spread faster than the wild strain, was first discovered in the United Kingdom in December 2020. On the other hand, beta (B.1.351) and gamma (P.1 lineage) variants were found first in South Africa and Brazil, respectively, in December 2020 and January 2021. The delta variant (B.1.617.2), initially discovered in India on October 2020, later spread to other nations, and resulted in a significant number of infections. Omicron has recently been found as a circulating variant of concern (B.1.1.521) with various subgroups and has been reported to primarily affect younger individuals (Firouzabadi et al., 2023). Each of these variants led to the emergence of different COVID-19 waves across the world. Few studies have examined the impact of various variants on the development of ARDS during SARS-CoV-2 infection. The clinical characteristics of the alpha variant and the wild SARS-CoV-2 variant (Wuhan strain) were compared in a retrospective cohort study. Patients infected with the alpha variant had a higher severity of ARDS than those infected with the wild type. Similarly, enhanced 28-day mortality was observed for the alpha variant (Ritchie et al., 2022). Another retrospective study in Vietnam investigated the clinical characteristics and case fatality of patients affected by the delta variant. The overall fatality rate was 52.2%, and ARDS was mostly seen to occur in non-survivors; patients who developed ARDS when exposed to the delta variant had a significant mortality rate (70%) as well (Do et al., 2023). In addition, the time for onset of disease (ARDS) after admission was longer in the delta variant compared to the wild strain (Peng et al., 2022). Researchers have recently started to investigate the clinical features of the omicron variant. Patients with the delta variant are highly prone to developing ARDS compared to those infected with omicron (de Prost et al., 2017). Similar results have been obtained in a retrospective multi-center-matched cohort study conducted in Belgium while assessing the clinical severity between delta and omicron variants. When compared to patients with the delta variant, in-hospital mortality was quite low in those infected with omicron (van Goethem et al., 2022). Khamis et al. (2022) performed a retrospective case-series investigation to examine the clinical characteristics of several COVID-19 variants (wild, alpha, delta, and omicron). Results have demonstrated that patients with the alpha variant are more prone than others to experience ARDS (Khamis et al., 2022). On the other hand, one retrospective analysis to determine the disease severity among many variants, including wild strain, delta, and omicron, showed that the severity of the disease dramatically decreased from wild strain to delta and omicron. Authors have reported a higher prevalence of ARDS in patients infected wild SARS-CoV-2 variant than those infected with the other two (Han et al., 2023). Although we cannot definitively say which strain is more likely to cause ARDS than others, the majority of studies report that the prevalence of ARDS is comparatively high in patients infected with alpha variant or wild SARS-CoV-2 strain.
The COVID-19 pandemic is persisting despite strict prevention protocols and the development of numerous vaccines. Patients admitted to the hospital due to COVID-19 infection greatly develop ARDS. ARDS is a fatal disease due to its heterogeneity, presence of comorbidities, and the limited number of pharmacological therapies developed. The ARDS research has certain limitations, such as the lack of conventional diagnostic techniques, ineffective animal models, and difficulty recognizing distinct phenotypes among patients. This makes it challenging for clinicians to treat ARDS in patients with COVID-19. Analysis of the prevalence of ARDS in COVID-19 patients showed a clear link between ARDS and SARS-CoV-2 as it induces the development of ARDS through the production of massive amounts of inflammatory cytokines. Alveolar epithelial cells and ACE2 receptors are primarily involved in COVID-19-induced ARDS pathogenesis. However, the pathogenic mechanism needs to be addressed clearly in the future for the development of effective treatment of patients with COVID-19-induced ARDS. As of now, COVID-19 vaccinations are somewhat effective at lowering the severity of ARDS during SARS-CoV-2 infection. However, large-sample investigations are necessary to validate these findings. Nonetheless, some investigations have found that ARDS can occur following vaccination, the cause of which is still unknown. This necessitates additional research towards the creation of effective vaccinations for managing COVID-19-induced ARDS. In this review, we also attempted to present the effect of multiple SARS-CoV-2 variants on the development of ARDS. Though few studies have compared the severity of disease, further research is needed to understand the clinical characteristics and mortality of patients with ARDS caused by different SARS-CoV-2 variants. Considering the mutagenic nature of SARS-CoV-2 and the heterogeneity of ARDS, effective treatment methods are yet to be developed.
Acknowledgement: The authors express their gratitude to SASTRA Deemed-to-be University, Tamil Nadu, India for infrastructure and financial support. The authors also extend their appreciation for the contribution of Dr. Diraviyam Thirumalai and other members from the Biopharmaceutical Research Lab, SASTRA Deemed-to-be University.
Funding Statement: The authors received no specific funding for this study.
Author Contributions: The authors confirm their contributions to the paper as follows: Study Conception & Design: Senthil Visaga Ambi; Collection of Data, Review and Editing: Senthil Visaga Ambi, Priyadharshini Thanjavur Sriramamoorthi, Gayathri Gopal, Shibi Muralidhar, Sai Ramanan Eswaran, Danush Narayan Panneerselvam, Bhuvaneswaran Meiyanathan, Srichandrasekar Thuthikkadu Indhuprakash; Manuscript Preparation and Figure Drafting: Priyadharshini Thanjavur Sriramamoorthi, Shibi Muralidhar, Gayathri Gopal. All authors reviewed the results and approved the final version of the manuscript.
Availability of Data and Materials: Data sharing is not applicable to this article as no datasets were generated or analyzed during the current study.
Ethics Approval: Not applicable.
Conflicts of Interest: The authors declare that they have no conflicts of interest to report regarding the present study.
References
Azagew AW (2023). COVID-19 induced acute respiratory distress syndrome; A systematic review and meta-analysis, pp. 1–19. https://doi.org/10.21203/rs.3.rs-2184060/v1 [Google Scholar] [CrossRef]
Batah SS, Fabro AT (2021). Pulmonary pathology of ARDS in COVID-19: A pathological review for clinicians. Respiratory Medicine 17: 106239. https://doi.org/10.1016/j.rmed.2020.106239 [Google Scholar] [PubMed] [CrossRef]
Bellani G, Laffey JG, Pham T, Fan E, Brochard L et al. (2016). Epidemiology, patterns of care, and mortality for patients with acute respiratory distress syndrome in intensive care units in 50 countries. JAMA 315: 788–800. https://doi.org/10.1001/jama.2016.0291 [Google Scholar] [PubMed] [CrossRef]
Bernard GR, Artigas A, Brigham KL, Carlet J, Falke K et al. (1992). The American-European consensus conference on ARDS definitions, mechanisms, relevant outcomes, and clinical trial coordination. Americal Journal of Respiratory and Critical Care Medicine 149: 818–824. https://doi.org/10.1164/ajrccm.149.3.7509706. [Google Scholar] [CrossRef]
Beyerstedt S, Barbosa Casaro E, Bevilaqua Rangel É (2020). COVID-19: Angiotensin-converting enzyme 2 (ACE2) expression and tissue susceptibility to SARS-CoV-2 infection. European Journal of Clinical Microbiology and Infectious Diseases 40: 905–919. https://doi.org/10.1007/s10096-020-04138-6/Published [Google Scholar] [CrossRef]
Bhargava M, Viken K, Wang Q, Jagtap P, Bitterman P, Ingbar D, Wendt C (2017). Bronchoalveolar lavage fluid protein expression in acute respiratory distress syndrome provides insights into pathways activated in subjects with different outcomes. Scientific Reports 7: 319. https://doi.org/10.1038/s41598-017-07791-8 [Google Scholar] [PubMed] [CrossRef]
Boglione L, Olivieri C, Rostagno R, Poletti F, Moglia R, Bianchi B, Esposito M, Biffi S, Borrè S (2021). Role of the early short-course corticosteroids treatment in ARDS caused by COVID-19: A single-center, retrospective analysis. Advances in Medical Sciences 66: 262–268. https://doi.org/10.1016/j.advms.2021.04.002 [Google Scholar] [PubMed] [CrossRef]
Calfee CS, Delucchi K, Parsons PE, Thompson BT, Ware LB, Matthay MA (2014). Subphenotypes in acute respiratory distress syndrome: Latent class analysis of data from two randomised controlled trials. The Lancet Respiratory Medicine 2: 611–620. https://doi.org/10.1016/S2213-2600(14)70097-9 [Google Scholar] [PubMed] [CrossRef]
Cesta MC, Zippoli M, Marsiglia C, Gavioli EM, Cremonesi G, Khan A, Mantelli F, Allegretti M, Balk R (2023). Neutrophil activation and neutrophil extracellular traps (NETs) in COVID-19 ARDS and immunothrombosis. European Journal of Immunology 53: 1–10. https://doi.org/10.1002/eji.202250010 [Google Scholar] [PubMed] [CrossRef]
Chauvelot L, Bitker L, Dhelft F, Mezidi M, Orkisz M et al. (2020). Quantitative-analysis of computed tomography in COVID-19 and non COVID-19 ARDS patients: A case-control study. Journal of Critical Care 60: 169–176. https://doi.org/10.1016/j.jcrc.2020.08.006 [Google Scholar] [PubMed] [CrossRef]
Chuang TY, Tsai MH, Wu LM, Ho SJ, Yeh PS, Liu YL, Fred Yang HJ (2021). Successful treatment of tocilizumab and ivermectin for a patievnt with ARDS due to COVID-19. Journal of Microbiology, Immunology and Infection 54: 147–148. https://doi.org/10.1016/j.jmii.2020.09.007 [Google Scholar] [PubMed] [CrossRef]
Das KM, Lee EY, Langer RD, Larsson SG (2016). Middle east respiratory syndrome coronavirus: What does a radiologist need to know? American Journal of Roentgenology 206: 1193–1201. https://doi.org/10.2214/AJR.15.15363 [Google Scholar] [PubMed] [CrossRef]
de Prost N, Pham T, Carteaux G, Dessap AM, Brun-Buisson C et al. (2017). Etiologies, diagnostic work-up and outcomes of acute respiratory distress syndrome with no common risk factor: A prospective multicenter study. Annals of Intensive Care 7: 2526. https://doi.org/10.1186/s13613-017-0281-6 [Google Scholar] [PubMed] [CrossRef]
DeDent AM, Farrand E (2022). Vaccine-induced interstitial lung disease: A rare reaction to COVID-19 vaccination. Thorax 77: 9–10. https://doi.org/10.1136/thoraxjnl-2021-217985 [Google Scholar] [PubMed] [CrossRef]
Derwall M, Martin L, Rossaint R (2018). The acute respiratory distress syndrome: Pathophysiology, current clinical practice, and emerging therapies. Expert Review of Respiratory Medicine 20: 1021–1029. https://doi.org/10.1080/17476348.2018.1548280 [Google Scholar] [PubMed] [CrossRef]
Dhar Chowdhury S, Oommen AM (2020). Epidemiology of COVID-19. Journal of Digestive Endoscopy 11: 7. https://doi.org/10.1055/s-0040-1712187 [Google Scholar] [CrossRef]
Dhawan G, Kapoor R, Dhawan R, Singh R, Monga B, Giordano J, Calabrese EJ (2020). Low dose radiation therapy as a potential life saving treatment for COVID-19-induced acute respiratory distress syndrome (ARDS). Radiotherapy and Oncology 147: 212–216. https://doi.org/10.1016/j.radonc.2020.05.002 [Google Scholar] [PubMed] [CrossRef]
Ding L, Chen Y, Su N, Xu X, Yin J, Qiu J, Wang J, Zheng D (2020). Comparison of acute respiratory distress syndrome in patients with COVID-19 and influenza A (H7N9) virus infection. International Journal of Infectious Diseases 122: 593–598. https://doi.org/10.1016/j.ijid.2022.06.053 [Google Scholar] [PubMed] [CrossRef]
Do TV, Manabe T, Vu GV, Nong VM, Fujikura Y et al. (2023). Clinical characteristics and mortality risk among critically ill patients with COVID-19 owing to the B.1.617.2 (Delta) variant in Vietnam: A retrospective observational study. PLoS One 18: e0279713. https://doi.org/10.1371/journal.pone.0279713 [Google Scholar] [PubMed] [CrossRef]
Dreher M, Kersten A, Bickenbach J, Balfanz P, Hartmann B et al. (2020). The characteristics of 50 hospitalized COVID-19 patients with and without ARDS. Deutsches Arzteblatt International 117: 271–278. https://doi.org/10.3238/arztebl.2020.0271 [Google Scholar] [CrossRef]
Dushianthan A, Grocott MPW, Postle AD, Cusack R (2011). Acute respiratory distress syndrome and acute lung injury. Postgraduate Medical Journal 87: 612–622. https://doi.org/10.1136/pgmj.2011.118398 [Google Scholar] [PubMed] [CrossRef]
Eworuke E, Major JM, Gilbert McClain LI (2018). National incidence rates for acute respiratory distress syndrome (ARDS) and ARDS cause-specific factors in the United States (2006–2014). Journal of Critical Care 47: 192–197. https://doi.org/10.1016/j.jcrc.2018.07.002 [Google Scholar] [PubMed] [CrossRef]
Fanelli V, Vlachou A, Ghannadian S, Simonetti U, Slutsky AS, Zhang H (2013). Acute respiratory distress syndrome: New definition, current and future therapeutic options. Journal of Thoracic Disease 5: 326–334. https://doi.org/10.3978/j.issn.2072-1439.2013.04.05 [Google Scholar] [PubMed] [CrossRef]
Firouzabadi N, Ghasemiyeh P, Moradishooli F, Mohammadi S (2023). Update on the effectiveness of COVID-19 vaccines on different variants of SARS-CoV-2. International Immunopharmacology 117. https://doi.org/10.1016/j.intimp.2023.January [Google Scholar] [CrossRef]
Firstenberg MS, Abel E, Blais D, Crestanello J, Mangino JE (2011). Postvaccination influenza 2009 H1N1 respiratory failure requiring extracorporeal membrane oxygenation. Case Reports in Infectious Diseases 2011: 1–2. https://doi.org/10.1155/2011/167963 [Google Scholar] [PubMed] [CrossRef]
Folegatti PM, Ewer KJ, Aley PK, Angus B, Becker S et al. (2020). Safety and immunogenicity of the ChAdOx1 nCoV-19 vaccine against SARS-CoV-2: A preliminary report of a phase 1/2, single-blind, randomised controlled trial. The Lancet 396: 467–478. https://doi.org/10.1016/S0140-6736(20)31604-4 [Google Scholar] [PubMed] [CrossRef]
Forchette L, Sebastian W, Liu T (2021). A comprehensive review of COVID-19 virology, vaccines, variants, and therapeutics. Current Medical Science 41: 1037–1051. https://doi.org/10.1007/s11596-021-2395-1 [Google Scholar] [PubMed] [CrossRef]
Ganesh B, Rajakumar T, Malathi M, Manikandan N, Nagaraj J, Santhakumar A, Elangovan A, Malik YS (2021). Epidemiology and pathobiology of SARS-CoV-2 (COVID-19) in comparison with SARS, MERS: An updated overview of current knowledge and future perspectives. Clinical Epidemiology and Global Health 10. https://doi.org/10.1016/j.cegh.2020.100694 [Google Scholar] [PubMed] [CrossRef]
Gattinoni L, Chiumello D, Caironi P, Busana M, Romitti F, Brazzi L, Camporota L (2020). COVID-19 pneumonia: Different respiratory treatments for different phenotypes? Intensive Care Medicine 46: 1099–1102. https://doi.org/10.1007/s00134-020-06033-2 [Google Scholar] [PubMed] [CrossRef]
Gibson PG, Qin L, Puah SH (2020). COVID-19 acute respiratory distress syndrome (ARDSClinical features and differences from typical pre-COVID-19 ARDS. Medical Journal of Australia 213: 54–56. https://doi.org/10.5694/mja2.50674 [Google Scholar] [PubMed] [CrossRef]
Grasselli G, Tonetti T, Protti A, Langer T, Girardis M et al. (2020). Pathophysiology of COVID-19-associated acute respiratory distress syndrome: A multicentre prospective observational study. The Lancet Respiratory Medicine 8: 1201–1208. https://doi.org/10.1016/S2213-2600(20)30370-2 [Google Scholar] [PubMed] [CrossRef]
Grieco DL, Bongiovanni F, Chen L, Menga LS, Cutuli SL et al. (2020). Respiratory physiology of COVID-19-induced respiratory failure compared to ARDS of other etiologies. Critical Care 24: 122. https://doi.org/10.1186/s13054-020-03253-2 [Google Scholar] [PubMed] [CrossRef]
Gu WJ, Wan YD, Tie HT, Kan QC, Sun TW (2014). Risk of acute lung injury/acute respiratory distress syndrome in critically ill adult patients with pre-existing diabetes: A meta-analysis. PLoS One 9: e90426. https://doi.org/10.1371/journal.pone.0090426 [Google Scholar] [PubMed] [CrossRef]
Gujski M, Jankowski M, Rabczenko D, Goryński P, Juszczyk G (2022). The prevalence of acute respiratory distress syndrome (ARDS) and outcomes in hospitalized patients with COVID-19—A study based on data from the polish national hospital register. Viruses 14: 76. https://doi.org/10.3390/v14010076 [Google Scholar] [PubMed] [CrossRef]
Han X, Chen J, Chen L, Jia X, Fan Y et al.(2023). Comparative analysis of clinical and CT findings in patients with SARS-CoV-2 original strain, Delta and Omicron variants. Biomedicines 11: 901. https://doi.org/10.3390/biomedicines11030901 [Google Scholar] [PubMed] [CrossRef]
Hasan SS, Capstick T, Ahmed R, Kow CS, Mazhar F, Merchant Ha, Zaidi STR (2020). Mortality in COVID-19 patients with acute respiratory distress syndrome and corticosteroids use: A systematic review and meta-analysis. Expert Review of Respiratory Medicine 14: 1149–1163. https://doi.org/10.1080/17476348.2020.1804365 [Google Scholar] [PubMed] [CrossRef]
Heching M, Lev S, Shitenberg D, Dicker D, Kramer MR (2021). Surfactant for the treatment of ARDS in a patient with COVID-19. Chest 160: e9–e12. https://doi.org/10.1016/j.chest.2021.01.028 [Google Scholar] [PubMed] [CrossRef]
Heinz FX, Stiasny K (2021). Profiles of current COVID-19 vaccines. Wiener Klinische Wochenschrift 133: 271–283. https://doi.org/10.1007/s00508-021-01835-w [Google Scholar] [PubMed] [CrossRef]
Hojyo S, Uchida M, Tanaka K, Hasebe R, Tanaka Y, Murakami M, Hirano T (2020). How COVID-19 induces cytokine storm with high mortality. Inflammation and Regeneration 40: 1054. https://doi.org/10.1186/s41232-020-00146-3 [Google Scholar] [PubMed] [CrossRef]
Horie S, McNicholas B, Rezoagli E, Pham T, Curley G et al. (2020). Emerging pharmacological therapies for ARDS: COVID-19 and beyond. Intensive Care Medicine 46: 2265–2283. https://doi.org/10.1007/s00134-020-06141-z [Google Scholar] [PubMed] [CrossRef]
Hu B, Guo H, Zhou P, Shi ZL (2021). Characteristics of SARS-CoV-2 and COVID-19. Nature Reviews Microbiology 19: 141–154. https://doi.org/10.1038/s41579-020-00459-7 [Google Scholar] [PubMed] [CrossRef]
Huang Y, Yang C, Xu XF, Xu W, Liu SW (2020). Structural and functional properties of SARS-CoV-2 spike protein: Potential antivirus drug development for COVID-19. Acta Pharmacologica Sinica 41: 1141–1149. https://doi.org/10.1038/s41401-020-0485-4 [Google Scholar] [PubMed] [CrossRef]
Hung NN, Sin CC, Kim CS, Shyang KS, Ching YM, Teck TS (2022). Methylprednisolone in severe COVID-19 with acute respiratory distress syndrome—Less is more? Medical Journal of Malaysia 77: 650–654. [Google Scholar]
Huppert LA, Matthay MA, Ware LB (2019). Pathogenesis of acute respiratory distress syndrome. Seminars in Respiratory and Critical Care Medicine 40: 31–39. https://doi.org/10.1055/s-0039-1683996 [Google Scholar] [PubMed] [CrossRef]
Johns M, George S, Taburyanskaya M, Poon YK (2021). A review of the evidence for corticosteroids in COVID-19. Journal of Pharmacy Practice 35: 626–637. https://doi.org/10.1177/0897190021998502 [Google Scholar] [PubMed] [CrossRef]
Kaku S, Nguyen CD, Htet NN, Tutera D, Barr J, Paintal HS, Kuschner WG (2020). Acute respiratory distress syndrome: Etiology, pathogenesis, and summary on management. Journal of Intensive Care Medicine 35: 723–737. https://doi.org/10.1177/0885066619855021 [Google Scholar] [PubMed] [CrossRef]
Kaushik SK, Bobdey S, Faujdar DS, Anand V, Kumar Yadav A, (2021). Coronavirus disease 2019 vaccines: Perspectives and update. Medical Journal Armed Forces India 77: S245–S249. https://doi.org/10.1016/j.mjafi.2021.04.003 [Google Scholar] [PubMed] [CrossRef]
Khamis F, Al Awaidy S, Ba’Omar M, Osman W, Chhetri S et al.(2022). The impact of demographic, clinical characteristics and the various COVID-19 variant types on all-cause mortality: A case-series retrospective study. Diseases 10: 100. https://doi.org/10.3390/diseases10040100 [Google Scholar] [PubMed] [CrossRef]
Ko RE, Oh DK, Choi SM, Park S, Park JE, Lee JG, Kim YT, Jeon K (2022). Lung transplantation for severe COVID-19-related ARDS. Therapeutic Advances in Respiratory Disease 16. https://doi.org/10.1177/17534666221081035 [Google Scholar] [PubMed] [CrossRef]
Kolilekas L, Loverdos K, Giannakaki S, Vlassi L, Levounets A, Zervas E, Gaga M (2020). Can steroids reverse the severe COVID-19 induced cytokine storm? Journal of Medical Virology 92: 2866–2869. https://doi.org/10.1002/jmv.26165 [Google Scholar] [PubMed] [CrossRef]
Koo HJ, Lim S, Choe J, Choi SH, Sung H, Do KH (2018). Radiographic and CT features of viral pneumonia. Radiographics 38: 719–739. https://doi.org/10.1148/rg.2018170048 [Google Scholar] [PubMed] [CrossRef]
Kotta S, Aldawsari HM, Badr-Eldin SM, Binmahfouz LS, Bakhaidar RB, Sreeharsha N, Nair AB, Ramnarayanan C (2021). Lung targeted lipopolymeric microspheres of dexamethasone for the treatment of ARDS. Pharmaceutics 13: 1347. https://doi.org/10.3390/pharmaceutics13091347 [Google Scholar] [PubMed] [CrossRef]
Kuba K, Yamaguchi T, Penninger JM (2021). Angiotensin-converting enzyme 2 (ACE2) in the pathogenesis of ARDS in COVID-19. Frontiers in Immunology 12: 1–7. https://doi.org/10.3389/fimmu.2021.732690 [Google Scholar] [PubMed] [CrossRef]
Kwee TC, Kwee RM (2020). Chest CT in COVID-19: What the radiologist needs to know. Radiographics 40: 1848–1865. https://doi.org/10.1148/rg.2020200159 [Google Scholar] [PubMed] [CrossRef]
Lascarrou JB (2021). COVID-19-related ARDS: One disease, two trajectories, and several unanswered questions. The Lancet Respiratory Medicine 9: 1345–1347. https://doi.org/10.1016/S2213-2600(21)00381-7 [Google Scholar] [PubMed] [CrossRef]
Li X, Ma X (2020). Acute respiratory failure in COVID-19: Is it “typical” ARDS? Critical Care 24: 198. https://doi.org/10.1186/s13054-020-02911-9 [Google Scholar] [PubMed] [CrossRef]
Longobardo A, Snow TAC, Montanari C, Shulman R, Singer M, Arulkumaran N (2021). COVID-19 and non-COVID ARDS patients demonstrate a distinct response to low dose steroids—A retrospective observational study. Journal of Critical Care 62: 46–48. https://doi.org/10.1016/j.jcrc.2020.11.012 [Google Scholar] [PubMed] [CrossRef]
Lotz C, Muellenbach RM, Meybohm P, Mutlak H, Lepper PM et al. (2021). Effects of inhaled nitric oxide in COVID-19-induced ARDS—Is it worthwhile? Acta Anaesthesiologica Scandinavica 65: 629–632. https://doi.org/10.1111/aas.13757 [Google Scholar] [PubMed] [CrossRef]
Marini JJ, Hotchkiss JR, Broccard AF (2003). Bench-to-bedside review: Microvascular and airspace linkage in ventilator-induced lung injury. Critical Care 7: 435–444. https://doi.org/10.1186/cc2392 [Google Scholar] [PubMed] [CrossRef]
Mason RJ (2020). Pathogenesis of COVID-19 from a cell biology perspective. European Respiratory Journal 55: 2000607. https://doi.org/10.1183/13993003.00607-2020 [Google Scholar] [PubMed] [CrossRef]
Matthay MA, Zemans RL (2011). The acute respiratory distress syndrome: Pathogenesis and treatment. Annual Review of Pathology: Mechanisms of Disease 6: 147–163. https://doi.org/10.1146/annurev-pathol-011110-130158 [Google Scholar] [PubMed] [CrossRef]
Matthay MA, Zemans RL, Zimmerman GA, Arabi YM, Beitler JR, Mercat A, Herridge M, Randolph AG, Calfee CS (2018). Acute respiratory distress syndrome. Nature Reviews Disease Primers 5: 319. https://doi.org/10.1038/s41572-019-0069-0 [Google Scholar] [PubMed] [CrossRef]
Meyer NJ, Gattinoni L, Calfee CS (2021). Acute respiratory distress syndrome. The Lancet 398: 622–637. https://doi.org/10.1016/S0140-6736(21)00439-6 [Google Scholar] [PubMed] [CrossRef]
Modjarrad K, Roberts CC, Mills KT, Castellano AR, Paolino K et al. (2019). Safety and immunogenicity of an anti-Middle East respiratory syndrome coronavirus DNA vaccine: A phase 1, open-label, single-arm, dose-escalation trial. The Lancet Infectious Diseases 19: 1013–1022. https://doi.org/10.1016/S1473-3099(19)30266-X [Google Scholar] [PubMed] [CrossRef]
Murray JF, Matthay MA, Luce JM, Flick MR (1988). Pulmonary perspectives an expanded definition of the adult respiratory distress syndrome 1. American Review of Respiratory Diseases 139: 720–723. https://doi.org/10.1164/ajrccm/138.3.720 [Google Scholar] [CrossRef]
Neubauer A, Johow J, Mack E, Burchert A, Meyn D et al. (2021). The janus-kinase inhibitor ruxolitinib in SARS-CoV-2 induced acute respiratory distress syndrome (ARDS). Leukemia 35: 2917–2923. https://doi.org/10.1038/s41375-021-01374-3 [Google Scholar] [PubMed] [CrossRef]
Nova Z, Skovierova H, Calkovska A (2019). Alveolar-capillary membrane-related pulmonary cells as a target in endotoxin-induced acute lung injury. International Journal of Molecular Sciences 20: 831. https://doi.org/10.3390/ijms20040831 [Google Scholar] [PubMed] [CrossRef]
Ou X, Liu Y, Lei X, Li P, Mi D et al. (2020). Characterization of spike glycoprotein of SARS-CoV-2 on virus entry and its immune cross-reactivity with SARS-CoV. Nature Communications 11: 1967. https://doi.org/10.1038/s41467-020-15562-9 [Google Scholar] [PubMed] [CrossRef]
Our World in Data (2023). Coronavirus (COVID-19) Vaccinations. https://ourworldindata.org/covid-vaccinations (accessed 09/03/2022). [Google Scholar]
Panico FF, Troster EJ, Oliveira CS, Faria A, Lucena M, João PRD, Saad ED, Foronda FAK, Delgado AF, de Carvalho WB (2015). Risk factors for mortality and outcomes in pediatric acute lung injury/acute respiratory distress syndrome. Pediatric Critical Care Medicine 16: e194–e200. https://doi.org/10.1097/PCC.0000000000000490 [Google Scholar] [PubMed] [CrossRef]
Peng J, Li Q, Dong J, Yuan G, Wang D (2022). Case report: The experience of managing a moderate ARDS caused by SARS-CoV-2 Omicron BA.2 ariant in Chongqing, China: Can we do better? Frontiers in Medicine 9: 921135. https://doi.org/10.3389/fmed.2022.921135 [Google Scholar] [PubMed] [CrossRef]
Pullen MF, Skipper CP, Hullsiek KH, Bangdiwala AS, Pastick KA et al. (2020). Symptoms of COVID-19 outpatients in the United States. Open Forum Infectious Diseases 7: 1708. https://doi.org/10.1093/ofid/ofaa271 [Google Scholar] [PubMed] [CrossRef]
Qiao Q, Liu X, Yang T, Cui K, Kong L, Yang C, Zhang Z (2021). Nanomedicine for acute respiratory distress syndrome: The latest application, targeting strategy, and rational design. Acta Pharmaceutica Sinica B 11: 3060–3091. https://doi.org/10.1016/j.apsb.2021.04.023 [Google Scholar] [PubMed] [CrossRef]
Raju R, Prajith V, Biatris PS, Sam Johnson Udaya Chander J (2021). Therapeutic role of corticosteroids in COVID-19: A systematic review of registered clinical trials. Future Journal of Pharmaceutical Sciences 7: 727. https://doi.org/10.1186/s43094-021-00217-3 [Google Scholar] [PubMed] [CrossRef]
Ranieri VM, Rubenfeld GD, Thompson BT, Ferguson ND, Caldwell E, Fan E, Camporota L, Slutsky AS (2012). Acute respiratory distress syndrome: The Berlin definition. JAMA 307: 2526–2533. https://doi.org/10.1001/jama.2012.5669 [Google Scholar] [PubMed] [CrossRef]
Ranjeva S, Pinciroli R, Hodell E, Mueller A, Hardin CC, Thompson BT, Berra L (2021). Identifying clinical and biochemical phenotypes in acute respiratory distress syndrome secondary to coronavirus disease-2019. eClinicalMedicine 34: 100829. https://doi.org/10.1016/j.eclinm.2021.100829 [Google Scholar] [PubMed] [CrossRef]
Reilly JP, Calfee CS, Christie JD (2019). Acute respiratory distress syndrome phenotypes. Seminars in Respiratory and Critical Care Medicine 40: 19–30. https://doi.org/10.1055/s-0039-1684049 [Google Scholar] [PubMed] [CrossRef]
Rezoagli E, Fumagalli R, Bellani G (2017). Definition and epidemiology of acute respiratory distress syndrome. Annals of Translational Medicine 5: 282. https://doi.org/10.21037/atm.2017.06.62 [Google Scholar] [PubMed] [CrossRef]
Ritchie AI, Kadwani O, Saleh D, Baharlo B, Broomhead LR et al. (2022). Clinical and survival differences during separate COVID-19 surges: Investigating the impact of the Sars-CoV-2 alpha variant in critical care patients. PLoS One 17: 1–10. https://doi.org/10.1371/journal.pone.0269244 [Google Scholar] [PubMed] [CrossRef]
Riviello ED, Kiviri W, Twagirumugabe T, Mueller A, Banner-Goodspeed VM, Officer L, Novack V, Mutumwinka M, Talmor DS, Fowler RA (2016). Hospital incidence and outcomes of the acute respiratory distress syndrome using the Kigali modification of the Berlin definition. American Journal of Respiratory and Critical Care Medicine 193: 52–59. https://doi.org/10.1164/rccm.201503-0584OC [Google Scholar] [PubMed] [CrossRef]
Robalo Q, de Mot L, Vandromme M, van Goethem N, Gabrio A, Chung PYJ, Meurisse M, Catteau L, Thijs C, Blot K (2023). Association between COVID-19 primary vaccination and severe disease caused by SARS-CoV-2 delta variant among hospitalized patients: A Belgian retrospective cohort study. Vaccines 11: 1–21. https://doi.org/10.3390/vaccines11010014 [Google Scholar] [PubMed] [CrossRef]
Sahin Tutak A, Söylemez F, Konuk HB, Çakmak E, Karakaya B, Doğan A, Sayiner HS, Aksöz S, Alev M (2021). A patient presenting with ARDS after COVID-19 vaccination: A COVID-19 case report. Journal of Infection and Public Health 14: 1395–1397. https://doi.org/10.1016/j.jiph.2021.05.017 [Google Scholar] [PubMed] [CrossRef]
Sampathkumar P, Temesgen Z, Smith TF, Thompson RL (2003). SARS: Epidemiology, clinical presentation, management, and infection control measures. Mayo Clinic Proceedings 78: 882–890. https://doi.org/10.4065/78.7.882 [Google Scholar] [PubMed] [CrossRef]
Samudrala PK, Kumar P, Choudhary K, Thakur N, Wadekar GS, Dayaramani R, Agrawal M, Alexander A (2020). Virology, pathogenesis, diagnosis and in-line treatment of COVID-19. European Journal of Pharmacology 883: 173375. https://doi.org/10.1016/j.ejphar.2020.173375 [Google Scholar] [PubMed] [CrossRef]
Sanyaolu A, Okorie C, Marinkovic A, Patidar R, Younis K, Desai P, Hosein Z, Padda I, Mangat J, Altaf M (2020). Comorbidity and its impact on patients with COVID-19. SN Comprehensive Clinical Medicine 2: 1069–1076. https://doi.org/10.1007/s42399-020-00363-4 [Google Scholar] [PubMed] [CrossRef]
Sarma A, Christenson SA, Byrne A, Mick E, Pisco AO et al. (2021). Tracheal aspirate RNA sequencing identifies distinct immunological features of COVID-19 ARDS. Nature Communications 12: 1033. https://doi.org/10.1038/s41467-021-25040-5 [Google Scholar] [PubMed] [CrossRef]
Seo JW, Kim SE, Choi EY, Hong KS, Oh TH et al. (2021). Risk factors and a scoring system to predict ARDS in patients with COVID-19 pneumonia in Korea: A multicenter cohort study. Disease Markers 2021: 1–7. https://doi.org/10.1155/2021/8821697 [Google Scholar] [PubMed] [CrossRef]
Sharp C, Millar AB, Medford ARL (2015). Advances in understanding of the pathogenesis of acute respiratory distress syndrome. Respiration 89: 420–434. https://doi.org/10.1159/000381102 [Google Scholar] [PubMed] [CrossRef]
Siempos II, Grapsa E, Adamos g, Andrianopoulos I, Tsolaki V et al. (2022). Association between vaccination status and mortality among intubated patients with COVID-19-related acute respiratory distress syndrome. JAMA Network Open 5: E2235219. https://doi.org/10.1001/jamanetworkopen.2022.35219 [Google Scholar] [PubMed] [CrossRef]
Singh R, Kang A, Luo X, Jeyanathan M, Gillgrass A, Afkhami S, Xing Z (2021). COVID-19: Current knowledge in clinical features, immunological responses, and vaccine development. FASEB Journal 35: e21409. https://doi.org/10.1096/fj.202002662R [Google Scholar] [PubMed] [CrossRef]
Sinha P, Calfee CS, Cherian S, Brealey D, Cutler S et al. (2020). Prevalence of phenotypes of acute respiratory distress syndrome in critically ill patients with COVID-19: A prospective observational study. The Lancet Respiratory Medicine 8: 1209–1218. https://doi.org/10.1016/S2213-2600(20)30366-0 [Google Scholar] [PubMed] [CrossRef]
Siordia JA (2020). Epidemiology and clinical features of COVID-19: A review of current literature. Journal of Clinical Virology 127. https://doi.org/10.1016/j.jcv.2020.104357 [Google Scholar] [PubMed] [CrossRef]
Su CM, Wang L, Yoo D (2021). Activation of NF-κB and induction of proinflammatory cytokine expressions mediated by ORF7a protein of SARS-CoV-2. Scientific Reports 11: 1–12. https://doi.org/10.1038/s41598-021-92941-2 [Google Scholar] [PubMed] [CrossRef]
Tabary M, Khanmohammadi S, Araghi F, Dadkhahfar S, Tavangar SM (2020). Pathologic features of COVID-19: A concise review. Pathology Research and Practice 216: 153097. https://doi.org/10.1016/j.prp.2020.153097 [Google Scholar] [PubMed] [CrossRef]
Taher A, Lashkari M, Keramat F, Hashemi SH, Sedighi L, Poorolajal J, Mehrpooya M (2023). Comparison of the efficacy of equivalent doses of dexamethasone, methylprednisolone, and hydrocortisone for treatment of COVID-19-related acute respiratory distress syndrome: A prospective three-arm randomized clinical trial. Wiener Medizinische Wochenschrift 173: 140–151. https://doi.org/10.1007/s10354-022-00993-4 [Google Scholar] [PubMed] [CrossRef]
Tang X, Du RH, Wang R, Cao TZ, Guan LL et al. (2020). Comparison of hospitalized patients with ARDS caused by COVID-19 and H1N1. Chest 158: 195–205. https://doi.org/10.1016/j.chest.2020.03.032 [Google Scholar] [PubMed] [CrossRef]
TenHoor T, Mannino DM, Moss M (2001). Risk factors for ARDS in the United States: Analysis of the 1993 national mortality followback study. Chest 119: 1179–1184. https://doi.org/10.1378/chest.119.4.1179 [Google Scholar] [PubMed] [CrossRef]
Tignanelli CJ, Hemmila MR, Rogers MAM, Raghavendran K (2019). Nationwide cohort study of independent risk factors for acute respiratory distress syndrome after trauma. Trauma Surgery and Acute Care Open 4: e000249. https://doi.org/10.1136/tsaco-2018-000249 [Google Scholar] [PubMed] [CrossRef]
Tojo K, Yamamoto N, Tamada N, Mihara T, Abe M, Nishii M, Takeuchi I, Goto T (2023). Early alveolar epithelial cell necrosis is a potential driver of COVID-19-induced acute respiratory distress syndrome. iScience 26: 105748. https://doi.org/10.1016/j.isci.2022.105748 [Google Scholar] [PubMed] [CrossRef]
Tomashefski JF (2020). Pulmonary pathology of acute respiratory distress syndrome Annual Review of Pathology: Mechanisms of Disease 6: 147–163. [Google Scholar]
Tsai YT, Ku HC, Maithreepala SD, Tsai YJ, Chen LF, Ko NY, Konara Mudiyanselage SP (2022). Higher risk of acute respiratory distress syndrome and risk factors among patients with COVID-19: A systematic review, meta-analysis and meta-regression. International Journal of Environmental Research and Public Health 19: 1–15. https://doi.org/10.3390/ijerph192215125 [Google Scholar] [PubMed] [CrossRef]
Tzotzos SJ, Fischer B, Fischer H, Zeitlinger M (2020). Incidence of ARDS and outcomes in hospitalized patients with COVID-19: A global literature survey. Critical Care 24: 516. https://doi.org/10.1186/s13054-020-03240-7 [Google Scholar] [PubMed] [CrossRef]
Van Goethem N, Chung PYJ, Meurisse M, Vandromme M, De Mo L et al. (2022). Clinical severity of SARS-CoV-2 omicron variant compared with delta among hospitalized COVID-19 patients in Belgium during Autumn and Winter season 2021–2022. Viruses 14: 1297. https://doi.org/10.3390/v14061297 [Google Scholar] [PubMed] [CrossRef]
Venkadapathi J, Govindarajan VK, Sekaran S, Venkatapathy S (2021). A minireview of the promising drugs and vaccines in pipeline for the treatment of COVID-19 and current update on clinical trials. Frontiers in Molecular Biosciences 8: 637378. https://doi.org/10.3389/fmolb.2021.637378 [Google Scholar] [PubMed] [CrossRef]
Villar J, Ferrando C, Martínez D, Ambrós A, Muñoz T et al. (2020). Dexamethasone treatment for the acute respiratory distress syndrome: A multicentre, randomised controlled trial. The Lancet Respiratory Medicine 8: 267–276. https://doi.org/10.1016/S2213-2600(19)30417-5 [Google Scholar] [PubMed] [CrossRef]
Volkov L, Delpuech M, Conrad M, Courte G, Cravoisy A, Nace L, Baumann C, Gibot S (2023). Clinical outcomes and characteristics of critically ill patients with influenza- and COVID—Induced ARDS: A retrospective, matched cohort study. Frontiers in Medicine 9: 1027984. https://doi.org/10.3389/fmed.2022.1027984 [Google Scholar] [CrossRef]
Wang C, Wang Z, Wang G, Lau JYN, Zhang K, Li W (2021). COVID-19 in early 2021: Current status and looking forward. Signal Transduction and Targeted Therapy 6: 114. https://doi.org/10.1038/s41392-021-00527-1 [Google Scholar] [PubMed] [CrossRef]
Wilson JG, Calfee CS (2020). ARDS subphenotypes: Understanding a heterogeneous syndrome. Critical Care 24: 102. https://doi.org/10.1186/s13054-020-2778-x [Google Scholar] [PubMed] [CrossRef]
World Health Organization (2023). Coronavirus disease (COVID-19) pandemic. https://www.who.int/emergencies/diseases/novel-coronavirus-2019 (accessed 10/10 2021). [Google Scholar]
Wu C, Chen X, Cai Y, Xia J, Zhou X et al. (2020). Risk factors associated with acute respiratory distress syndrome and death in patients with coronavirus disease 2019 pneumonia in Wuhan, China. JAMA Internal Medicine 180: 934–943. https://doi.org/10.1001/jamainternmed.2020.0994 [Google Scholar] [PubMed] [CrossRef]
Xia B, Shen X, He Y, Pan X, Liu FL et al. (2021). SARS-CoV-2 envelope protein causes acute respiratory distress syndrome (ARDS)-like pathological damages and constitutes an antiviral target. Cell Research 31: 847–860. https://doi.org/10.1038/s41422-021-00519-4 [Google Scholar] [PubMed] [CrossRef]
Xu W, Sun NN, Gao HN, Chen ZY, Yang Y, Ju B, Tang LL (2021). Risk factors analysis of COVID-19 patients with ARDS and prediction based on machine learning. Scientific Reports 11: 1708. https://doi.org/10.1038/s41598-021-82492-x [Google Scholar] [PubMed] [CrossRef]
Yoshimura Y, Sasaki H, Miyata N, Miyazaki K, Okudela K et al. (2020). An autopsy case of COVID-19-like acute respiratory distress syndrome after mRNA-1273 SARS-CoV-2 vaccination. International Journal of Infectious Diseases 121: 98–101. https://doi.org/10.1016/j.ijid.2022.04.057 [Google Scholar] [PubMed] [CrossRef]
Yuki K, Fujiogi M, Koutsogiannaki S (2020). COVID-19 pathophysiology: A review. Clinical Immunology 215: 108427. https://doi.org/10.1016/j.clim.2020.108427 [Google Scholar] [PubMed] [CrossRef]
Zhang Z, Chen L, Ni H (2015). The effectiveness of corticosteroids on mortality in patients with acute respiratory distress syndrome or acute lung injury: A secondary analysis. Scientific Reports 5: 549. https://doi.org/10.1038/srep17654 [Google Scholar] [PubMed] [CrossRef]
Zhang JJY, Lee KS, Ang LW, Leo YS, Young BE (2020). Risk Factors for severe disease and efficacy of treatment in patients infected with COVID-19: A systematic review, meta-analysis, and meta-regression analysis. Clinical Infectious Diseases 71: 2199–2206. https://doi.org/10.1093/cid/ciaa576 [Google Scholar] [PubMed] [CrossRef]
Zhang W, Zhang M, Kuang Z, Huang Z, Gao L, Zhu J (2021). The risk factors for acute respiratory distress syndrome in patients with severe acute pancreatitis: A retrospective analysis. Medicine 100: e23982. https://doi.org/10.1097/MD.0000000000023982 [Google Scholar] [PubMed] [CrossRef]
Zhang X, Zhang X, Li S, Niu S (2020). ACE2 and COVID-19 and the resulting ARDS. Postgraduate Medical Journal 96: 403–407. https://doi.org/10.1136/postgradmedj-2020-137935 [Google Scholar] [PubMed] [CrossRef]
Zheng J, Miao J, Guo R, Guo J, Fan Z, Kong X, Gao R, Yang L (2022). Mechanism of COVID-19 causing ARDS: Exploring the possibility of preventing and treating SARS-CoV-2. Frontiers in Cellular and Infection Microbiology 12. https://doi.org/10.3389/fcimb.2022.931061 [Google Scholar] [PubMed] [CrossRef]
Zhou P, Lou YX, Wang XG, Hu B, Zhang L, Zhang W, Si HR, Zhu Y, Li B, Huang CL et al. (2020). A pneumonia outbreak associated with a new coronavirus of probable bat origin. Nature 579: 270–273. https://doi.org/10.1038/s41586-020-2012-7 [Google Scholar] [PubMed] [CrossRef]
Cite This Article
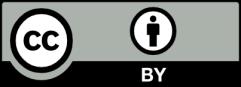