Open Access
REVIEW
A perspective on molecular and cellular biology-based developmental toxicology biomarkers
1 Department of Environmental Science, The University of Burdwan, Burdwan, India
2 Department of Environmental Science, Aghorekamini Prakashchandra Mahavidyalaya, Subhas Nagar, India
3 Department of Environmental Science, Sukanta Mahavidyalaya, University of North Bengal, Dhupguri, India
* Corresponding Author: PALAS SAMANTA. Email:
# Equal contribution
BIOCELL 2023, 47(12), 2579-2590. https://doi.org/10.32604/biocell.2023.031114
Received 15 May 2023; Accepted 12 September 2023; Issue published 27 December 2023
Abstract
The process of development is intricate and couple-dependent phenomenon. Accordingly, the study of molecular and cellular biology-based developmental toxicology biomarkers increasingly is becoming an important part of risk assessment and management of chemicals for detection of health outcomes and/or biological endpoint like cytotoxicity, cell death, etc. Since, the evolution of developmental toxicology field a number of tools/markers have been developed or addressed to deal with developmental outcomes, which can ultimately be used for the development of adverse outcome pathways (AOPs) of developmental toxicants. As a result, this paper provides an overview of the current state of developmental toxicology biomarkers and describes the strategies used in the selection and evaluation of such biomarkers in the context of developmental toxicity studies. Here, we discuss about the biological markers that are directly linked to developmental toxicity with respect to future revolutionary perspectives. Additionally, this chapter will address different associated outcomes of developmental exposure by intriguing advance techniques. The discussion focuses on the challenges associated with the development of biomarkers for developmental toxicity and highlights some of the recent advances in this area. Finally, the chapter concludes with a brief discussion of the future prospects for the use of molecular and cellular biology-based developmental toxicity biomarkers. Hope the present state of the art will provide a succinct summary of recent developments of biomarkers of developmental toxicology.Keywords
Starting in 1960s, when it became clear that thalidomide, a sedative/hypnotic commonly used at the time, had a particularly bad impact on human limb growth, there was a significant rise in consciousness regarding developmental toxic chemicals (Hougaard, 2021). Prior to that, different substances were being examined on adults, but infrequent testing was carried out on pregnant animals, and it was widely believed that the placental barrier protected the foetus from outside agents. Additionally, there has been significant progress made to identify probable developmental toxins in the environment and control human exposure to them since the beginning of the sixties, when prenatal sensitivity was first recognized (Estevan et al., 2017; Luconi et al., 2022). In addition to birth problems, growth retardation, mortality (including the loss of embryos and foetuses), and functional impairments in the newborn are increasingly recognized as detrimental developmental impacts (Draskau et al., 2020). In laboratory animals, over 1,200 distinct substances have been found to have detrimental developmental consequences; however, the implications of exposure to many of these substances in humans are not well known (Giavini and Menegola, 2012; Luconi et al., 2022).
Developmental toxicology, or the investigation of how poisons affect vital aspects of healthy development, has made strides scientifically since the 1960s. As toxicology has improved, so has the science of evaluating the risks that chemicals have on human health. Risk analysts typically depend on two types of data to make risk predictions: estimations of human toxication extent to a specific substance and assessments of the substance’s human toxicity according to developmental/embryonic outcomes of progeny from trial mothers-to-be subjected to that compound (Hougaard, 2021). Sometimes, data becomes accessible from other avenues, such as (1) relationships between structure and activity connecting the substance’s toxicity with its other family members, (2) the outcomes of the chemical’s in vitro experiments, and (3) human epidemiology findings regarding the compound’s impact (Committee on Developmental Toxicology, 2000; Luconi et al., 2022).
Assessors look for correct mechanism-based empirical information or evidence based on a substance’s clear comprehensive toxicity mechanisms, as established by developmental toxicologists, to construct their risk assessments (Estevan et al., 2017; Hougaard, 2021; Escher et al., 2022). These are scanty data. The evaluation of a substance’s potential effects on development is restricted by a number of assumptions. The finest evidence currently available for extrapolation of toxicant impacts on human beings comes from animal bioassays, primarily employing mammals (Malits et al., 2022). Just a tiny percentage of the substances used in society and the environment have undergone thorough evaluations for their possible harmful effects on animals due to the high cost and lengthy nature of these bioassays (European Commission, 2020a). The numerous efforts to build less complex and expensive experiments using tissue explants, cultured cells, or purified biological substances have thus far proven to be of little use for forecasting the effects of chemicals on human embryonic and fetal growth (Malits et al., 2022).
When suggesting the correct amounts of people’s exposure to a particular xenobiotic, risk analysts frequently employ four types of default hypotheses since they lack precise mechanism-based empirical evidence (Maertens et al., 2022; Escher et al., 2022). They begin by assuming that outcomes from tests on animals are applicable to people. People are considered the most vulnerable animals, barring contrary evidence, and tolerable human exposure is established at a level that is ten times lower than the highest exposure dose that has no adverse effects on animal’s growth (Xie et al., 2020; Luconi et al., 2022). Then, an additional 10-fold drop is implemented to adjust for the likelihood that the animal’s developmental responses, which were usually observed at sub-chronic exposition to the substance, would not accurately represent human reactions at extended (chronic) doses (Hougaard, 2021). Third, to account for the potential that sensitivity differs amongst human beings, with certain individuals fundamentally more susceptible to the substance, a tenfold decrease is included. Fourth, if the toxicology information for a substance is not satisfied, a tenfold decrease may occasionally be implemented (Ujházy et al., 2012; DeSesso, 2018; Hougaard, 2021). Due to the vulnerability of growing structures, a further child-specific ratio is occasionally used (often a 10-fold decrease). Although a lot of analysts are interested in employing mechanism-based empirical information rather than these present assumptions (approx. 10,000-fold exacerbated decrease in suitable exposure for people outside provided by the animal’s test), test results for the analysts’ use are frequently scarce due to scarce funds and have an unidentified relevance to people due to a shortage of knowledge of the fundamental mechanisms of developmental toxicology (Committee on Developmental Toxicology, 2000; Escher et al., 2022; Hougaard, 2021).
As a result, extrapolating, meaning, expenses, and efficiency issues provide difficulties for risk evaluators. Scientists are being sought to formulate methods for testing that utilize our rapidly developing understanding of normal growth in order to deliver accurate data with better forecasts for human developmental results (Ujházy et al., 2012; DeSesso, 2018; Hougaard, 2021). This is because a substantial amount of ambient substances, either naturally or synthesized, have not undergone sufficient evaluation for prospective developmental toxicity (DeSesso, 2018; Hougaard, 2021). The assessment of the human danger posed by environmental toxicants is a continuous challenge. Therefore, extensive investigation of in vivo developmental toxicity continues to necessitate an extensive number of animal models. Accordingly, the current paper provides an outline of developmental toxicity ranging from developmental defects to toxicity testing. This also highlights upon how the outcomes obtained from these toxicity methods might function as in vivo biomarkers of predictability to development and explains the way the information acquired might be utilized to anticipate chemical’s ability to generate in vivo embryonic damage.
History of Developmental Toxicology
Teratology or more specifically the developmental toxicology, or the scientific study of aberrant development, possesses a lengthy history that is largely mirrored in developmental biology (Ujházy et al., 2012). In the 19th century and into the first half of the 20th century, advancement was made in understanding the underlying reasons for aberrant development by scientific means. By that time, it had become apparent that congenital defects in humans might be a result of inherited, dietary (such as cretinism), pathogenic (such as congenital rubella syndrome), and chemical variables (Daston et al., 2014). It was additionally understood that these signs of disturbed development might be induced experimentally in a variety of animal species. The chronology of developmental toxicology is postulated as follows:
ca. 1750 BCE: During sixth Amorite king of the Old Babylonian Empire, Hammurabi, congenital malformations were recorded as rare and historical events. They believed that appearance of a specific type of malformation was indication of a specific future events (Warkany, 1971, 1977).
ca. 700 BCE: In the ruins of Mesopotamia, similar types of Hammurabi record have been found.
ca. 1500–500 BCE: Many of the gods in Hinduism are represented as having multiple limbs. This believe continuing at present. Similar believe also present in other religion.
The scientific study of poisons dates to Ancient Egypt, China, and Greece. Numerous toxins, that include hemlock, are discussed in the 1500 BCE Ebers Papyrus.
Shen Hung (2605 BCE, China) tested hundreds of herbs and wrote the first Pharmacopoeia.
Until the 18th century, that deformed organisms develop from miniature versions of themselves (Preformism theory).
ca. 1849: Willem Vrolik published a magnificent, early atlas of malformations and the specimens are kept in Vrolik museum.
Orfila, a 19thcentury Spanish physician and scientist, is a founder of modern toxicology that autopsied poison victims to evaluate target organ specific effects (liver, kidney, GI track, brain, etc.).
French naturalist Camille Dareste (1822–1899) excelled in investigative embryonic development. His publication, “Research on the Artificial Production of Monstrosities, or Essays on Experimental Teratogenesis (Dareste, 1876)”, was the first conclusive proof showing anomalies in the neural tube have been brought about by a malfunction of neural tube’s closing instead of sealed neural tube burst. Significantly, Wilson’s ideas, that emerged almost one century afterwards (Wilson, 1959, 1973), were motivated by and prophesied by Dareste’s five exploratory teratology theories.
In 1894: A groundbreaking (though brief-lived) scholarly publication focused on birth abnormalities (Book: “Teratologia: A Quarterly Journal of Antenatal Pathology”) was published by the prominent Scottish doctor J. W. Ballantyne.
In 19th century, the idea of teratogenesis emerged as a consequence of the advancement of illustrative embryology, in which anomalies were interpreted as deformities or mistakes made throughout a stage of growth/development.
By the 20th century, epigenesis, or the interplay among genetic programme and surroundings, was proved as well-established theory.
During the middle half of the twentieth century, scientists had shown that environmental influences could result in deformities and possibly multigenerational impacts.
The detailed chronology of origin of developmental events are displayed in Fig. 1.
Figure 1: Timeline of different developmental events (Source: DeSesso, 2018).
Challenges of Developmental Toxicology
REACH (Registration, Evaluation, Authorization, and Restriction of Chemical Substances in Europe), a European legislation, regulates between 70,000 and 100,000 chemical compounds, to which it has recommended to add compounds like pharmaceuticals (ECHA, 2020). International guidelines, namely Organization for Economic Co-operation and Development (OECD) and International Council for Harmonisation of Technical Requirements for Registration of Pharmaceuticals for Human Use (ICH) generally checking the developmental outcomes of chemicals and pharmaceutical compounds, respectively (Estevan et al., 2017; Hougaard, 2021). More specifically, the REACH is the European legislation while the OECD and ICH are harmonized the guidelines for regulatory testing, they do not regulate anything indeed the OECD guideline are used for in the data dossier for REACH approval (ECHA, 2020). Complete evaluation of developmental hazards of all substances is not possible due to the substantial expenditures and extensive usage of laboratory organisms (Munirathinam et al., 2023). Experimental toxicity offers the benefit of allowing for oversight of intoxication, surroundings, and heredity. Since only extremely large tonnage levels (>1,000 tonnes/year) cause substantial harm, REACH has boosted toxicity evaluation of industrial compounds, but information regarding developmental outcomes is still rare (ECHA, 2017). Furthermore, nevertheless extremely thorough testing procedures do not always ensure the identification of every developmental toxicity. In addition, certain results are too infrequent to identify an influence, and there is insufficient information available on how the organs of the offspring operate to a developmental toxicant. A unique and comprehensive protocol exists for developmental neurological damage; however, it is infrequently. Similar to drugs, only a small number of functional results in the progeny are evaluated (European Medicines Agency, 2017). In addition, testing standards mostly apply to substances that are being produced, not to the many substances created by human activities like welding process, burning, or using electronic cigarettes. According to Schmitz-Felten et al. (2016), only few committed scientists from academic institutions and government agencies are mostly responsible for evaluating these aspects. Currently, the EU assesses every substance separately, but it is now aware that hazards from numerous chemical encounters should be considered during evaluation of developmental damage, for instance, in employees who are exposed to compounds in both their personal and professional lives (European Commission, 2020b), not to mention medications.
Types of Developmental Defects and Occurrence Pattern
Developmental abnormalities are frequently described as those which start in the developing embryo and fetus, or in the prenatal stage. Birth defects can take on a wide range of shapes and relationships, from straightforward structural defects to frequently horrifying malformations which might damage a whole bodily part. Table 1 lists a few of the most prevalent kinds of abnormalities. Table 2 summarizes the occurrence of different developmental outcomes. The substance or circumstance that is harmful to the mother-to-be during pregnancy is then considered a developmental toxin (Baines et al., 2021). However, growth occurs continuously throughout the life cycle and includes things like the adult’s ongoing regrowth of skin, intestinal lining, and hematopoietic system as well as the child’s and adolescent’s continued improvement and differentiation of their skeletal, nervous, and reproductive systems (Giavini and Menegola, 2012). Therefore, it appears unfair to limit the definition of developmental contaminants to substances that can harm an embryo or foetus when a mother is exposed to them during pregnancy. The line between the two, however, is not clearly defined, thus it is to be anticipated that poison exposures at other times could have an impact on growth in children, adolescents, and adults as well as have an impact on gametes and reproductive organs that may not manifest until a long time after in the gestation period.
Major developmental abnormalities, commonly known as major congenital disorders, affect 120,000 of the roughly 4 million live births that take place annually (a baby with birth defect/4½ minute or 1 in every 33 babies) in the United States (www.cdc.gov/birthdefects). These oddities include those that pose a serious impairment, need extensive treatment/surgery, or are potentially fatal. Worldwide, 240000 newborns die inside 28 days each year from congenital disorders (Table 3). Congenital disorders cause a further 170,000 deaths of kids below the age of 5 per year (WHO, 2023). Around the world, 6% of newborns are thought to have a congenital disease, which is related with hundreds of thousands of deaths (WHO, 2023).
Causes of Developmental Defects
Several factors can result in birth abnormalities (Feldkamp et al., 2017; Davidsen et al., 2021). Table 4 summarizes the list of factors causing birth defects. It is estimated that between 65 and 70 percent of all birth abnormalities have an unidentified aetiology. About 20% of congenital anomalies are hereditary in origin, and 3% to 5% of all congenital anomalies are caused by chromosomal, or cytogenetic, disorders (Finnell, 1999). There are issues like trisomy (the inheriting a full additional chromosome) or 4p-syndrome (changes in minor portion of genomic complement) among them (Feldkamp et al., 2017; Davidsen et al., 2021; Tanteles and Suri, 2007). Maternal viral infections including rubella, CMV, or HIV can cause two to three percent of developmental abnormalities (Cuckle, 2014). Unbalances in the mother’s metabolism, like those induced by diabetes, account for a minor portion of birth abnormalities (Cuckle, 2014). Malformations caused by teratogens account for 2% to 3% of deformities (Finnell, 1999; Baines et al., 2021; Tanteles and Suri, 2007). Those are seen as the outcome of iatrogenic or xenobiotic triggers during gestation.
Congenital disorders tend to have a greater incidence within families (i.e., demographic factors) and nations with limited resources, where financial hardship (i.e., socioeconomic condition) might be a hidden driver of these conditions (WHO, 2023). According to estimates, countries with low or middle incomes account for 94% of cases of severe congenital abnormalities. This greater likelihood, which is an implicit predictor, may be related to pregnant women’s likely insufficient access to enough nutrient-rich meals, their heightened contact with substances or variables like illness and alcohol, or their worsened accessibility to healthcare and screening (WHO, 2023).
Another risk associated with poor intrauterine foetal growth is maternal age (Ujházy et al., 2012). Chromosome anomalies such as Down syndrome, are more likely in older mothers (Marikawa et al., 2020; WHO, 2023). Others are caused by external influences, called environmental factors including infections such as pregnancy-related infections (such as syphilis, rubella, and Zika), radiation exposure, particular contaminants, pregnant nutrient deficiencies (such as an iron or folic deficiency), illnesses (such as maternal diabetes), or medications (such as alcohol and phenytoin) (Ujházy et al., 2012; Feldkamp et al., 2017; Tanteles and Suri, 2007; WHO, 2023). Although intricate relationships between genes and the environment are theorized, the reasons of the majority of congenital abnormalities, such as clubfoot, cleft lip, and palate, are unexplained (Ujházy et al., 2012).
Principle of Developmental Toxicology
Even though teratogenic stressors only contribute to a relatively small portion of total birth defects, 3 million people in the United States currently suffer from developmental disorders brought on by teratogenic infection in utero. Keep in mind that if we comprehend the six fundamental tenets of teratology, almost all teratogen-induced birth abnormalities are prevented (Finnell, 1999). The six principles are described below (Hood, 2012; Rogers, 2015):
Principle 1: Susceptibility to Teratogenesis Depends on the Genotype of the Conceptus and a Manner in which this Interacts with Adverse Environmental Factors;
Principle 2: Susceptibility to Teratogenesis Varies with the Developmental Stage at the Time of Exposure to an Adverse Influence;
Principle 3: Teratogenic Agents Act in Specific Ways (Mechanisms) on Developing Cells and Tissues to Initiate Sequences of Abnormal Developmental Events (Pathogenesis);
Principle 4: The Access of Adverse Influences to Developing Tissues Depends on the Nature of the Influence (Agent);
Principle 5: The Four Manifestations of Deviant Development are Death, Malformation, Growth Retardation and Functional;
Principle 6: Manifestations of Deviant Development Increase in Frequency and Degree as Dosage Increases, from No-Effect to the Totally Lethal Level.
Developmental Toxicology Biomarkers
Developmental biomarkers are generally classified as (a) exposure, (b) effect, and finally (c) susceptibility (Giavini and Menegola, 2012). Generally, exposure biomarkers are treated as early markers, which may act as an interaction between different chemical agent and with different target molecules (Barr and Buckley, 2012). Homeostasis, proper growth, distinctness, development, or behavior are interfered by architectural or functional changes that are either permanent or reversible. External insult, encompassing harmful substances like beverages, food, chemical substances, and physical elements, is what causes developmental/embryonic toxicity (Gupta and Gupta, 2020). These biomarkers can include physical changes, such as malformations or growth abnormalities, as well as cognitive and behavioral changes (Giavini and Menegola, 2012). Exposure to developmental toxicants can lead to a variety of adverse health outcomes, including birth defects, neurological disorders, and developmental delays. Some examples of developmental toxicology biomarkers include changes in gene expression, alterations in hormone levels, and changes in cellular processes (Marikawa et al., 2020). These biomarkers can be used to identify potential risks to human health and to develop strategies for reducing exposure to toxic substances. The exposure of developmental toxicants refers to the harmful effects of environmental or chemical factors on the development of an organism, particularly during pregnancy. Such exposure may lead to developmental abnormalities, growth retardation, behavioral alterations, and other adverse consequences. Developmental toxicity can be caused by various factors such as exposure to radiation, toxic chemicals, drugs, infectious agents, and hormonal imbalances in the mother (Xie et al., 2020; Marikawa, 2022). The severity and type of developmental toxicity dependent upon factors, including the timing, duration, and intensity of exposure. Here is a breakdown of the ways that developmental toxicity can occur:
Maternal exposure: Maternal exposure occurs when pregnant women are exposed to toxic substances that can affect the development of the fetus. Substances like alcohol, cigarette smoke, and certain medications can cross the placenta and damage fetal organs, leading to various abnormalities (CDC, 2023).
Neonatal exposure: Neonatal exposure occurs when infants are exposed to toxins during the first few months of life. This can happen through breast milk, contaminated food or water, or through skin contact with contaminated surfaces or objects (Cuckle, 2014).
Childhood exposure: Children can also be exposed to developmental toxins during their early life when their organs and tissues are still developing. Exposure can happen through various means, including exposure to lead-based paint or lead-contaminated soil, pesticides, or other chemicals in the environment. Such exposure can lead to neuro-developmental disorders, cognitive deficits, and behavioral problems (WHO, 2023).
Overall, the exposure of developmental toxicant can have severe and long-lasting effects on an individual’s health and well-being. To prevent developmental toxicity, it is essential to avoid or minimize exposure to toxic substances during pregnancy and early childhood. This can be achieved through proper nutrition, healthy lifestyle habits, avoiding environmental toxins, and using appropriate protective measures while working in environments with potential developmental toxic substances.
Biomarker of effects generally manifest during pregnancy and can also include long-term consequences throughout the life of the individual (Barr and Buckley, 2012). Here are some of the potential effects of developmental toxicity (Barr and Buckley, 2012): 1. Structural abnormalities: Exposure to chemicals during development can cause physical malformations such as limb reduction, facial clefts, and heart defects (Sujan et al., 2023); 2. Growth retardation: Some chemicals can impair the growth of the fetus, leading to low birth weight and developmental delays (Zacharias et al., 2023); 3. Neurological damage: Exposure to certain toxins during development can impair growth of central nervous system (CNS). This could lead to problems with cognition, behavior, and mood regulation later in life (Popova et al., 2023); 4. Endocrine dysfunction: Chemicals that interfere with hormones can disrupt the normal development of the endocrine system, which can have long-term effects on reproductive health, metabolism, and other bodily functions (Interdonato et al., 2023); 5. Immune system impairment: Toxin exposure during development may also weaken the immune system, making a person more vulnerable to contagious infections along with other illnesses (Smith et al., 2023); 6. Carcinogenesis: A subset of developmental toxins can also increase the risk of cancer later in life (Knox et al., 2023). Overall, developmental toxicity can have a wide range of effects on the developing organism, many of which can persist throughout the lifespan of the individual (Giavini and Menegola, 2012).
Developmental toxicology is the study of how exposure to various toxic substances during pregnancy can impact the development of the fetus. Based on time, length, and exposure dose, certain substances can interfere with critical developmental processes and result in a range of birth defects and developmental disabilities. However, developmental toxicology biomarkers are used to identify potential risks to developing embryos, fetuses, or newborns due to exposure to chemicals, drugs, or other environmental factors. These biomarkers can include changes in gene expression, protein levels, epigenetic alterations, and structural abnormalities. By monitoring these biomarkers, scientists can identify potential developmental toxicants and determine safe exposure levels for pregnant women and infants. There are various factors that can influence the susceptibility of a developing fetus to developmental toxicants: 1. Stage of development: Different organs and systems develop at different stages during pregnancy, and are more vulnerable during certain critical windows of development (Kidd et al., 2023). For example, the central nervous system is most vulnerable to environmental toxicants during the first trimester; 2. Dose and duration of exposure: The severity of toxic effects increases with the dose and duration of exposure. Even low-level exposures to certain substances over an extended period may trigger an aggregate impact on foetal development; 3. Route of exposure: The method by which a toxicant enters the body can have an impact on its effect. For example, a substance that is ingested will be metabolized in the liver, while inhalants will be absorbed more quickly into the bloodstream; 4. Genetic susceptibility: Some individuals are genetically more vulnerable to environmental toxicants, due to variations in gene expression or enzyme systems. For example, genetic factors may influence the ability of the liver to metabolize certain substances (Matthee et al., 2023); 5. Interaction with other substances: Exposure to multiple toxicants can have a synergistic effect, where the combined effect is greater than the effects of each substance alone. For example, exposure to lead and alcohol during pregnancy can result in cognitive deficits that are greater than the deficits resulting from exposure to either substance alone. Overall, developmental toxicology aims to identify the factors that increase susceptibility to toxicants, and to develop strategies for reducing exposure to these substances during critical periods of fetal development.
Developmental Toxicity Testing Methods
Developmental toxicity testing methods are generally divided into two categories namely cellular assays, 58 mainly concerned with stem cell and whole embryoculture (WEC) test (Ciallella et al., 2022; Tait et al., 2021; Vračko et al., 2022).
The embryonic stem cell test (EST) and rat limb bud micromass (MM) represent two cellular tests for embryonic toxicity that has granted authorization by the European Centre for the Validation of Alternative Methods (ECVAM) (Louisse et al., 2012). A number of additional (embryonic stem) cell-based tests that concentrate on various in vitro outputs for developmental toxicity are additionally disclosed in the literature; however, ECVAM has not yet approved all these assays except EST. The cellular tests are addressed in greater depth as follows:
MM test: Primary cultured cells isolated from midbrains and/or limb buds are employed in MM test. Exclusively limb bud cells, which originate from rat embryo harvested at gestational day (GD) 14, are utilized in the standardized MM test technique. Limb buds are separated and placed on higher-density plates (MM cultures), which enables chondrocyte differentiation (Louisse et al., 2012). The impact on the development and differentiating limb bud cells is evaluated following 5 days exposure to the test substance. The forecasting algorithm for the MM test only uses the EC50 values acquired in the differentiating assay because neither the EC50 results acquired in multiplicity testing nor the EC50 readings of both growth and differentiation resulted to better projections. The MM test was not advised for screening reasons since methotrexate, a potent embryotoxic chemical, was labeled as non-embryotoxic (Spielmann et al., 2004).
EST test: In 1990s, embryonic stem cells were initially employed to assess a substance’s potential for embryonic toxicity (Louisse et al., 2012). Due to these activities, the EST was created and approved by the ECVAM using its forecasting model (Reddy et al., 2023). This method evaluates the ability of a test substance to prevent the proliferating embryonic stem (ES-D3) and embryonic fibroblasts (3T3 cells) as well as 3T3 cells, as well as potentiality of a test substance to prevent ES-D3 cells differentiating into squeeze cardiomyocytes. The period of toxication lasts for 10 days, during that the medium is replaced twice (preferably either 3 or 5 days). The obtained EC50 values from these experiments are used in a model for forecasting to determine if the drug is strongly embryotoxic. The EST is suitable for evaluating the potential embryotoxicity of substance for regulatory screening (Louisse et al., 2012).
Other cellular assays: Specific gene expression for development of neurons (Stummann et al., 2007, 2011; Zimmer et al., 2011a, 2011b), differentiated osteoblasts, or endothelial differentiation are just a few in vitro outputs for developmental toxicity that are being examined in embryonic stem cell-based setups. These readings could serve as helpful biomarkers for negative impacts on particular developmental routes, such as neurodevelopment, development of bones, or vasculogenesis and/or angiogenesis, respectively. Utilizing toxicogenomics for acquiring markers has achieved significance over the past ten years (Afshari et al., 2011). By comparing normal cell gene expression profiles, cells subjected to teratogenic substances, and cells subjected to non-teratogenic in embryonic stem cell cultures exposed to various substances, transcriptomic studies have been utilized to distinguish teratogens from non-teratogens (van Dartel et al., 2011). To evaluate whether substances possess identical mechanisms of operation and whether processes impacted by in vivo intoxication are additionally regulated by embryo culture in in vitro, as well as to show the predictive ability of in vitro tests, toxicogenomic approaches are also proven to be beneficial.
Whole embryo culture (WEC) assays
The one and only WEC assay that was officially approved by the ECVAM is the post-implantation rat WEC test (Piersma et al., 2004). Other in vivo cultivation test includes the chicken embryotoxicity screening test (CHEST) and the frog embryo teratogenesis assay–Xenopus (FETAX). The utilization of zebrafish eggs for developmental toxicology studies has also been covered in a number of publications. Morphological analysis is generally employed to assess the developmental toxicological efficacy of the drug tested in the majority of investigations utilizing WECs.
The post implantation rat WEC test: For many years, rats’ WECs have been utilized to evaluate the developmental toxicity of several substances (Louisse et al., 2012). In WEC, pregnant rat’s embryonic cells (GD10) are cultivated for 48 h in 100% rat serum that contains the test substance. Following this phase of culture, evaluations of the head length, yolk sac diameter, and crown-to-rump length are obtained (Louisse et al., 2012), along with a score of the heartbeat and yolk sac circulation. Additionally, progress, morphological, developmental, and functional factors are assessed. Malformation frequency and sensitivity are noted. The maximum effect concentration (ICmax), 50% effect concentrations (IC50), and no observed effect concentrations (ICNOEC) are calculated. The ICmax thresholds for abnormalities and IC50 scores acquired in 3T3 proliferating method of EST were incorporated in an additional forecasting framework, whereas ICNOEC total morphological score (ICNOEC TMS) and IC50 scores for triggering abnormalities (IC50mal) had been utilized for categorization in the initial forecasting framework established for the WEC test (Piersma et al., 2004; Louisse et al., 2012). The embryotoxicity classifications of the test chemicals (non-, weak, or strong) were shown to be more accurately predicted by the second forecasting framework. In order to evaluate the likelihood of embryotoxicity of substances for regulatory assessment, it was determined that the WEC test was adequate (Piersma et al., 2004; Louisse et al., 2012).
CHEST: Chicken eggs are used in the CHEST to assess a compound’s probable developmental harm. were the authors of the technique’s initial descriptions. In CHEST, embryonic eggs are subjected to a variety of test substance doses for varying amounts of time, following which the results are evaluated. The quantity of aberrant, stunted-growth, and dying foetuses is counted, and the test substance’s developmental toxicity power is calculated based on this data (Louisse et al., 2012). The CHEST procedure is broken up into two phases: (i) determining the toxic dosage spectrum in the early period of administration (24 h; CHEST I); and (ii) determining the teratogenic dosage spectrum, which also includes later impacts on embryo development (days 2, 3, and 4; CHEST II). Following the CHEST study and other testing procedures in numerous investigations, it was determined that CHEST is a repeatable method of testing that provides quantitative data for analysis (Adler et al., 2011). For regular screening reasons and mechanistic research, both academia and industry utilize CHEST (Adler et al., 2011).
FETAX: Over the course of the organogenesis period, Xenopus laevis mid-blastula-stage eggs were subjected to the FETAX, a screening test for developmental toxicity (Mouche et al., 2011; Louisse et al., 2012). A test compound’s teratogenic capability is assessed after a 96-h exposure by rating the larval mortality and deformity rates as well as the larva lengths (Mouche et al., 2011). The resultant LC50, which represents 50% embryo death, is then divided by the EC50, which represents 50% induction of deformed larvae, to yield a teratogenic index (TI). The substance is classed as being positive if the TI is greater than 1.2 and as negatives if the TI is less than 1.0. If development, morphogenesis, or durability are disrupted by a substance with a TI between 1.0 and 1.2, the substance is categorized as positive, but just when the quantity that disrupts morphogenesis is less than the level that causes significant death (Mouche et al., 2011).
Zebra fish embryos: Danio rerio has grown in acceptance as an experimental aliquot for investigating embryonic toxicity in recent decades (Cassar et al., 2019; Beekhuijzen et al., 2015). Fertilized embryos of 24, 48, 72, and 144 h following toxicant intoxicatiion, Selderslaghs et al. (2009) measured TI data (LC50/EC50, malformation), and they were capable of distinguishing between four substances that were hazardous for growth and two that were not.
Instead of TI values, as is stated in the FETAX, the differentiation of positive from negatives was predicated on the reality that neither TI values were able to be computed for the two negative substances because neither LC50 nor EC50, abnormalities, had been determined (Selderslaghs et al., 2009). A bigger set of chemicals (27 chemicals) were classified employing this zebrafish test in a more recent investigation employing a forecasting framework with a TI threshold value of 2. A threshold of 72% and a precision of 100% were identified for these 27 chemicals when contrasting the categorization acquired in this zebrafish experiment with data from animals (Selderslaghs et al., 2012). In order to evaluate the zebrafish embryo’s growth based on certain time outcomes, Hermsen et al. (2011) created a quantitative assessment approach. The impact of test substances on zebrafish development is evaluated in their zebrafish embryotoxicity test utilizing a ratings system for development and teratogenic consequences (Hermsen et al., 2011). Based on investigations on locomotory movements (Selderslaghs et al., 2010) and nervous system transcription (Fan et al., 2010), zebrafish embryos were additionally employed to evaluate the neurodevelopmental toxicity possibility of substances. DeMicco et al. (2010) investigated impact of a number of pyrethroid insecticides in zebrafish embryos and came to the conclusion that the zebrafish could represent an excellent substitute model for investigating neurodevelopmental poisoning pathways as well as could possibly be utilized to determine substances that ought to be examined further in mammals.
Conclusion and Future Perspectives
Although developmental toxicity is a very complicated issue, the investigation addressed an eclectic aspect on challenges, factors affecting developmental defects, biomarkers of embryonic toxicity and different developmental toxicity testing methodologies. Despite the significant transformation has taken place, the primary hurdle is the extensive variety of drugs and substances, which makes it impossible to create suitable records for developmental toxicities for everyone. Given that there is scant or insufficient study in living things, researchers require immediate action to forecast potential hazards and quantify the risks associated with those compounds. For example, AOP systems and in silico techniques, this calls for an improved awareness of the pathogenic mechanisms behind disturbance of fertilization and foetal development. As a starting point for choosing combination of testing methodologies for specific testing batteries, the in vitro test system library should eventually include the entire reproduction cycle (including placental events).
It necessitates in vivo significance of the occasionally straightforward in vitro outcomes as well as how to translate in vitro dosages into in vivo dynamics. Nonetheless we must additionally address animal experiments as the benchmark for evaluating human hazards, investigate the impact of strain variations on hazardous reaction (Skovmand et al., 2020), and examine the ways outcomes made in animals and in vitro are associated with human beings’ confirmation (Bonde et al., 2017).
Generally developmental toxicity is connected with wider range of tissues/organs, such as the renal system, cardiovascular, and immunity. To bring about biomarkers that are connected with epigenetic alterations, a link between them and causality needs to be demonstrated. The gestation phase for both men and women, along with the interactions between infections throughout multiple phases in life and infections that occur concurrently, should also be taken into account when discussing times of vulnerability. The emphasis ought to change from data sufficient substances to the ones lacking a repository to novel kinds of substances, and mixtures of compounds and typical variables in daily life, such as non-chemical variables in the job-related environment, psychological strain, dietary habits, ageing, etc.
Developmental toxicology will undoubtedly be studied for the foreseeable future, it is assured. Developmental toxicology promotes research into both the problems and potential remedies to the problems mentioned and subsequently encourages initial studies, methodology documents, and critical reviews for a wide audience in the various sectors necessary to maintain the favorable conditions for the expansion of this growing discipline of study.
Toxicology investigations must take into account putative embryotoxicity and developmental toxicity since an egg and its growing organ framework are more susceptible to harmful substances than an adult’s organ. Numerous important aspects associated with initial embryonic organogenesis could be accurately reenacted in organoids as well. As a result, testing for developmental toxicology and/or embryotoxicity can be done using human organoids. Further investigation is required to overcome the shortcomings of the available human organoids, even if organoid innovations are intriguing vehicles for exploring embryonic toxicological evaluation. The twenty-first century will see sophisticated contemporary toxicity evaluations thanks to cutting-edge technologies and human organoids.
Acknowledgement: Authors are highly acknowledging the Principal of Sukanta Mahavidyalaya, Dhupguri and Aghorekamini Prakash Chandra Mahavidyalaya, Bengai and Head of Department of Environmental Science, the University of Burdwan for continuous support and encouragement during the course of writing this manuscript.
Funding Statement: The authors received no specific funding for this study.
Author Contributions: Study conception and design: S. Pal, P. Samanta; data collection: S. Dey, P. Samanta; draft manuscript preparation: S. Dey, S. Pal, A. R. Ghosh, P. Samanta. All authors reviewed the draft manuscript and approved the final version of the manuscript.
Availability of Data and Materials: Data sharing not applicable to this article as no datasets were generated or analyzed during the current study.
Ethics Approval: Not applicable.
Conflicts of Interest: The authors declare that they have no conflicts of interest to report regarding the present study.
References
Adler S, Basketter D, Creton S, Pelkonen O, Van Benthem J et al. (2011). Alternative (non-animal) methods for cosmetics testing: Current status and future prospects—2010. Archives of Toxicology 85: 367–485. https://doi.org/10.1007/s00204-011-0693-2 [Google Scholar] [PubMed] [CrossRef]
Afshari CA, Hamadeh HK, Bushel PR (2011). The evolution of bioinformatics in toxicology: Advancing toxicogenomics. Toxicological Sciences 120: S225–S237. https://doi.org/10.1093/toxsci/kfq373 [Google Scholar] [PubMed] [CrossRef]
Baines RP, Wolton K, Thompson CRL (2021). Dictyosteliumdiscoideum: An alternative nonanimal model for developmental toxicity testing. Toxicological Sciences 183: 302–318. https://doi.org/10.1093/toxsci/kfab097 [Google Scholar] [PubMed] [CrossRef]
Barr DB, Buckley B (2012). In vivo biomarkers and biomonitoring in reproductive and developmental toxicity. In: Gupta RC (ed.Reproductive and Developmental Toxicology, pp. 253–265. Amsterdam: Elsevier Inc. [Google Scholar]
Beekhuijzen M, de Koning C, Flores-Guillén ME, de Vries-Buitenweg S, Tobor-Kaplon M, van de Waart B, Emmen H (2015). From cutting edge to guideline: A first step in harmonization of the zebrafish embryotoxicity test (ZET) by describing the most optimal test conditions and morphology scoring system. Reproductive Toxicology 56: 64–76. https://doi.org/10.1016/j.reprotox.2015.06.050 [Google Scholar] [PubMed] [CrossRef]
Bonde JP, Flachs EM, Rimborg S, Glazer CH, Giwercman A et al. (2017). The epidemiologic evidence linking prenatal and postnatal exposure to endocrine disrupting chemicals with male reproductive disorders: A systematic review and meta-analysis. Human Reproduction Update 23: 104–125. https://doi.org/10.1093/humupd/dmw036 [Google Scholar] [PubMed] [CrossRef]
Cassar S, Beekhuijzen M, Beyer B, Chapin R, Dorau M, Hoberman A, Tornesi B (2019). A multi-institutional study benchmarking the zebrafish developmental assay for prediction of embryotoxic plasma concentrations from rat embryo-fetal development studies. Reproductive Toxicology 86: 33–44. https://doi.org/10.1016/j.reprotox.2019.02.004 [Google Scholar] [PubMed] [CrossRef]
CDC (2023). Data statistics on birth defects. https://www.cdc.gov/ncbddd/birthdefects/data.html (accessed on 05/05/2023) [Google Scholar]
Ciallella HL, Russo DP, Sharma S, Li Y, Sloter E, Sweet L, Huang H, Zhu H (2022). Predicting prenatal developmental toxicity based on the combination of chemical structures and biological data. Environmental Science & Technology 56: 5984–5998. https://doi.org/10.1021/acs.est.2c01040 [Google Scholar] [PubMed] [CrossRef]
Committee on Developmental Toxicology (2000). Scientific Frontiers in Developmental Toxicology and Risk Assessment. Washington DC: National Academy Press. https://www.ncbi.nlm.nih.gov/books/NBK225678/ (accessed on 05/05/2023) [Google Scholar]
Cuckle H (2014). Prenatal screening using maternal markers. Journal of Clinical Medicine 3: 504–520. https://doi.org/10.3390/jcm3020504 [Google Scholar] [PubMed] [CrossRef]
Dareste C (1876). Recherches sur la Production Artificielle des Monstruosités, ou Essais de TératogénieExpérimentale. Paris: C Reinwald. https://www.biodiversitylibrary.org/item/99425#page/7/mode/1up (accessed on 05/05/2023) [Google Scholar]
Daston GP, Beyer BK, Carney EW, Chapin RE, Friedman JM, Piersma AH, Scialli AR (2014). Exposure-based validation list for developmental toxicity screening assays. Birth Defects Research Part B Developmental and Reproductive Toxicology 101: 423–428. https://doi.org/10.1002/bdrb.21132 [Google Scholar] [PubMed] [CrossRef]
Davidsen N, Rosenmai AK, Lauschke K, Svingen T, Vinggaard AM (2021). Developmental effects of PFOS, PFOA and GenX in a 3D human induced pluripotent stem cell differentiation model. Chemosphere 279: 130624. https://doi.org/10.1016/j.chemosphere.2021.130624 [Google Scholar] [PubMed] [CrossRef]
DeMicco A, Cooper KR, Richardson JR, White LA (2010). Developmental neurotoxicity of pyrethroid insecticides in zebrafish embryos. Toxicological Sciences 113: 177–186. https://doi.org/10.1093/toxsci/kfp258 [Google Scholar] [PubMed] [CrossRef]
DeSesso JM (2018). The arrogance of teratology: A brief chronology of attitudes throughout history. Birth Defects Research 111: 123–141. https://doi.org/10.1002/bdr2.1422 [Google Scholar] [PubMed] [CrossRef]
Draskau MK, Spiller CM, Boberg J, Bowles J, Svingen T (2020). Developmental biology meets toxicology: Contributing reproductive mechanisms to build adverse outcome pathways. Molecular Human Reproduction 26: 111–116. https://doi.org/10.1093/molehr/gaaa001 [Google Scholar] [PubMed] [CrossRef]
ECHA (2017). Guidance on information requirements and chemical safety assessment, Version 6.0. In: Chapter R.7a: Endpoint Specific Guidance. Helsinki: European Chemicals Agency. https://echa.europa.eu/guidance-documents/guidance-on-information-requirements-and-chemical-safety-assessment (accessed on 05/05/2023) [Google Scholar]
ECHA (2020). The use of alternatives to testing on animals for the REACH regulation. In: Fourth Report under Article 117(3) of the REACH Regulation. ECHA, Finland: Helsinki. https://echa.europa.eu/documents/10162/0/alternatives_test_animals_2020_en.pdf (accessed on 05/05/2023) [Google Scholar]
Escher SE, Partosch F, Konzok S, Jennings P, Luijten M, Kienhuis A, de Leeuw V, Reuss R, Lindemann K, Bennekou SH (2022). Development of a roadmap for action on new approach methodologies in risk assessment. EFSA Supporting Publications 19: 7341E. https://efsa.onlinelibrary.wiley.com/doi/pdf/10.2903/sp.efsa.2022.EN-7341 (accessed on 05/05/2023) [Google Scholar]
Estevan C, Pamies D, Vilanova E, Sogorb MA (2017). OECD guidelines for in vivo testing of reproductive toxicity. In: Reproductive and Developmental Toxicology, pp. 163–178. Cambridge: Academic Press. https://doi.org/10.1016/B978-0-12-804239-7.00009-3 [Google Scholar] [CrossRef]
European Commission (2020a). Chemicals Strategy for Sustainability Towards a Toxic Free Environment. COM 667 final. Brussels: European Commission, 25. https://environment.ec.europa.eu/strategy/chemicals-strategy_en (accessed on 05/05/2023) [Google Scholar]
European Commission (2020b). Chemicals Strategy for Sustainability towards a Toxic-Free Environment. COM/2020/667 final. Brussels: European Commission, 2. https://eur-lex.europa.eu/legal-content/EN/TXT/?uri=COM%3A2020%3A667%3AFIN (accessed on 05/05/2023) [Google Scholar]
European Medicines Agency (2017). ICH S5 (R3) guideline on reproductive toxicology: Detection of toxicity to reproduction for human pharmaceuticals. Amsterdam. https://www.ema.europa.eu/en/ich-s5-r3-guideline-detection-reproductive-developmental-toxicity-human-pharmaceuticals-scientific (accessed on 05/05/2023) [Google Scholar]
Fan CY, Cowden J, Simmons SO, Padilla S, Ramabhadran R (2010). Gene expression changes in developing zebrafish as potential markers for rapid developmental neurotoxicity screening. Neurotoxicology and Teratology 32: 91–98. https://doi.org/10.1016/j.ntt.2009.04.065 [Google Scholar] [PubMed] [CrossRef]
Feldkamp ML, Carey JC, Byrne JLB, Krikov S, Botto LD (2017). Etiology and clinical presentation of birth defects: Population-based study. BMJ 357: j2249. https://doi.org/10.1136/bmj.j2249 [Google Scholar] [PubMed] [CrossRef]
Finnell RH (1999). Teratology: General considerations and principles. Journal of Allergy and Clinical Immunology 103: S337–S342. https://doi.org/10.1016/S0091-6749(99)70259-9 [Google Scholar] [PubMed] [CrossRef]
Giavini E, Menegola E (2012). Biomarkers of teratogenesis: Suggestions from animal studies. Reproductive Toxicology 34: 180–185. https://doi.org/10.1016/j.reprotox.2012.05.003 [Google Scholar] [PubMed] [CrossRef]
Gupta PK, Gupta PK (2020). Developmental toxicology. In: Gupta PK, ringer S (edsProblem Solving Questions in Toxicology: A Study Guide for the Board and other Examinations, pp. 137–143. Sringer. https://doi.org/10.1007/978-3-030-50409-0_10 [Google Scholar] [CrossRef]
Hermsen SA, van den Brandhof EJ, van der Ven LT, Piersma AH (2011). Relative embryotoxicity of two classes of chemicals in a modified zebrafish embryotoxicity test and comparison with their in vivo potencies. Toxicology in Vitro 25: 745–753. https://doi.org/10.1016/j.tiv.2011.01.005 [Google Scholar] [PubMed] [CrossRef]
Hood RD. (2012). Developmental and Reproductive Toxicology: A Practical Approach. 3rd edition. Boca Raton: CRC Press. https://doi.org/10.3109/9781841848211 [Google Scholar] [CrossRef]
Hougaard KS (2021). Next generation reproductive and developmental toxicology: Crosstalk into the future. Frontiers in Toxicology 3: 652571. https://doi.org/10.3389/ftox.2021.652571 [Google Scholar] [PubMed] [CrossRef]
Interdonato L, Siracusa R, Fusco R, Cuzzocrea S, Di Paola R (2023). Endocrine disruptor compounds in environment: Focus on women’s reproductive health and endometriosis. International Journal of Molecular Sciences 24: 5682. https://doi.org/10.3390/ijms24065682 [Google Scholar] [PubMed] [CrossRef]
Kidd SA, Gong J, Massazza A, Bezgrebelna M, Zhang Y, Hajat S (2023). Climate change and its implications for developing brains-in utero to youth: A scoping review. The Journal of Climate Change and Health 13: 100258. https://doi.org/10.1016/j.joclim.2023.100258 [Google Scholar] [CrossRef]
Knox KE, Dodson RE, Rudel RA, Polsky C, Schwarzman MR (2023). Identifying toxic consumer products: A novel data set reveals air emissions of potent carcinogens, reproductive toxicants, and developmental toxicants. Environmental Science & Technology 57: 7454–7465. https://doi.org/10.1021/acs.est.2c07247 [Google Scholar] [PubMed] [CrossRef]
Louisse J, Verwei M, Woutersen RA, Blaauboer BJ, Rietjens IM (2012). Toward in vitro biomarkers for developmental toxicity and their extrapolation to the in vivo situation. Expert Opinion on Drug Metabolism & Toxicology 8: 11–27. https://doi.org/10.1517/17425255.2012.639762 [Google Scholar] [PubMed] [CrossRef]
Luconi M, Sogorb MA, Markert UR, Benfenati E, May T, Wolbank S, Roncaglioni A, Schmidt A, Straccia M, Tait S (2022). Human-based new approach methodologies in developmental toxicity testing: A step ahead from the state of the art with a feto-placental organ-on-chip platform. International Journal of Environmental Research and Public Health 19: 15828. https://doi.org/10.3390/ijerph192315828 [Google Scholar] [PubMed] [CrossRef]
Maertens A, Golden E, Luechtefeld TH, Hoffmann S, Tsaioun K, Hartung T (2022). Probabilistic risk assessment-the keystone for the future of toxicology. Altex 39: 3–39. https://doi.org/10.14573/altex.2201081 [Google Scholar] [PubMed] [CrossRef]
Malits J, Naidu M, Trasande L (2022). Exposure to endocrine disrupting chemicals in Canada: Population-based estimates of disease burden and economic costs. Toxics 10: 146. https://doi.org/10.3390/toxics10030146 [Google Scholar] [PubMed] [CrossRef]
Marikawa Y (2022). Toward better assessments of developmental toxicity using stem cell-based in vitro embryogenesis models. Birth Defects Research 114: 972–982. https://doi.org/10.1002/bdr2.1984 [Google Scholar] [PubMed] [CrossRef]
Marikawa Y, Chen H, Menor M, Deng Y, Alarcon VB (2020). Exposure-based assessment of chemical teratogenicity using morphogenetic aggregates of human embryonic stem cells. Reproductive Toxicology 91: 74–91. https://doi.org/10.1016/j.reprotox.2019.10.004 [Google Scholar] [PubMed] [CrossRef]
Matthee C, Brown AR, Lange A, Tyler CR (2023). Factors determining the susceptibility of fish to effects of human pharmaceuticals. Environmental Science & Technology 57: 8845–8862. https://doi.org/10.1021/acs.est.2c09576 [Google Scholar] [PubMed] [CrossRef]
Mouche I, Malesic L, Gillardeaux O (2011). FETAX assay for evaluation of developmental toxicity. Drug Safety Evaluation: Methods and Protocols, 257–269. https://doi.org/10.1007/978-1-60761-849-2_15 [Google Scholar] [PubMed] [CrossRef]
Munirathinam P, Mathew AA, Shanmugasundaram V, Vivekananthan V, Purusothaman Y, Kim SJ, Chandrasekhar A (2023). A comprehensive review on triboelectric nanogenerators based on real-time applications in energy harvesting and self-powered sensing. Materials Science and Engineering: B 297: 116762. https://doi.org/10.1016/j.mseb.2023.116762 [Google Scholar] [CrossRef]
Piersma AH, Genschow E, Verhoef A, Spanjersberg MQ, Brown NA, Brady MC, Burns A, Clemann N, Seiler A, Spielmann H (2004). Validation of the postimplantation rat whole-embryo culture test in the international ECVAM validation study on three in vitro embryotoxicity tests. Alternatives to Laboratory Animals 32: 275–307. https://doi.org/10.1177/026119290403200307 [Google Scholar] [PubMed] [CrossRef]
Popova S, Charness ME, Burd L, Crawford A, Hoyme HE, Mukherjee R, Riley EP, Elliott EJ (2023). Fetal alcohol spectrum disorders. Nature Reviews Disease Primers 9: 11. https://doi.org/10.1038/s41572-023-00420-x [Google Scholar] [PubMed] [CrossRef]
Reddy N, Lynch B, Gujral J, Karnik K (2023). Regulatory landscape of alternatives to animal testing in food safety evaluations in the western world. Regulatory Toxicology and Pharmacology 143: 105470. https://doi.org/10.1016/j.yrtph.2023.105470 [Google Scholar] [PubMed] [CrossRef]
Rogers JM (2015). Developmental toxicology. In: Klaassen CD, Watkins JBIII (edsCasarett Doull’s Essentials of Toxicology, 3e. McGraw Hill. https://accesspharmacy.mhmedical.com/content.aspx?bookid=1540sectionid=92526062 (accessed on 05/05/2023) [Google Scholar]
Schmitz-Felten E, Kuhl K, Hougaard KS, Miranowicz-Dzierzawska K (2016). State of the art report on reproductive toxicants. In: European Risk Observatory Summary, pp. 1–61. Luxembourg: European Agency for Safety and Health at Work. [Google Scholar]
Selderslaghs IW, Blust R, Witters HE (2012). Feasibility study of the zebrafish assay as an alternative method to screen for developmental toxicity and embryotoxicity using a training set of 27 compounds. Reproductive Toxicology 33: 142–154. https://doi.org/10.1016/j.reprotox.2011.08.003 [Google Scholar] [PubMed] [CrossRef]
Selderslaghs IW, Hooyberghs J, de Coen W, Witters HE (2010). Locomotor activity in zebrafish embryos: A new method to assess developmental neurotoxicity. Neurotoxicology and Teratology 32: 460–471. https://doi.org/10.1016/j.ntt.2010.03.002 [Google Scholar] [PubMed] [CrossRef]
Selderslaghs IW, Van Rompay AR, de Coen W, Witters HE (2009). Development of a screening assay to identify teratogenic and embryotoxic chemicals using the zebrafish embryo. Reproductive Toxicology 28: 308–320. https://doi.org/10.1016/j.reprotox.2009.05.004 [Google Scholar] [PubMed] [CrossRef]
Skovmand A, Erdely A, Antonini JM, Nurkiewicz TR, Shoeb M et al. (2020). Inhalation of welding fumes reduced sperm counts and high fat diet reduced testosterone levels; differential effects in Sprague Dawley and Brown Norway rats. Particle and Fibre Toxicology 17: 1–14. https://doi.org/10.1186/s12989-019-0334-0 [Google Scholar] [PubMed] [CrossRef]
Smith D, Jheeta S, López-Cortés GI, Street B, Fuentes HV, Palacios-Pérez M (2023). On the inheritance of microbiome-deficiency: Paediatric functional gastrointestinal disorders, the immune system and the gut-brain axis. Gastrointestinal Disorders 5: 209–232. https://doi.org/10.3390/gidisord5020018 [Google Scholar] [CrossRef]
Spielmann H, Genschow E, Brown NA, Piersma AH, Verhoef A, Spanjersberg MQI, Huuskonen H, Paillard F, Seiler A (2004). Validation of the rat limb bud micromass test in the international ECVAM validation study on three in vitro embryotoxicity tests. Alternatives to Laboratory Animals 32: 245–274. https://doi.org/10.1177/026119290403200306 [Google Scholar] [PubMed] [CrossRef]
Stummann TC, Hareng L, Bremer S (2007). Embryotoxicity hazard assessment of methylmercury and chromium using embryonic stem cells. Toxicology 242: 130–143. https://doi.org/10.1016/j.tox.2007.09.022 [Google Scholar] [PubMed] [CrossRef]
Sujan AC, Pal A, Avalos LA, Young-Wolff KC (2023). A systematic review of in utero cannabis exposure and risk for structural birth defects. Frontiers in Pediatrics 11: 1149401. https://doi.org/10.3389/fped.2023.1149401 [Google Scholar] [PubMed] [CrossRef]
Tait S, Luconi M, Straccia M, Sogorb M (2021). A proposal of an innovative microfluidic platform for testing developmental toxicity. Toxicology Letters 350: S143–S144. https://doi.org/10.1016/S0378-4274(21)00580-4 [Google Scholar] [CrossRef]
Tanteles GA, Suri M (2007). Classification and aetiology of birth defects. Paediatrics and Child Health 17: 233–243. https://doi.org/10.1016/j.paed.2007.03.005 [Google Scholar] [CrossRef]
Ujházy E, Mach M, Navarová J, Brucnerová I, Dubovicktfytf M (2012). Teratology-past, present and future. Interdisciplinary Toxicology 5: 163–168. https://doi.org/10.2478/v10102-012-0027-0 [Google Scholar] [PubMed] [CrossRef]
van Dartel DA, Pennings JL, de la Fonteyne LJ, Brauers KJ, Claessen S, van Delft JH, Kleinjans J, Piersma AH (2011). Evaluation of developmental toxicant identification using gene expression profiling in embryonic stem cell differentiation cultures. Toxicological Sciences 119: 126–134. https://doi.org/10.1093/toxsci/kfq291 [Google Scholar] [PubMed] [CrossRef]
Vračko M, Stanojević M, Sollner Dolenc M (2022). Comparison of predictions of developmental toxicity for compounds of solvent data set. SAR and QSAR in Environmental Research 33: 35–48. https://doi.org/10.1080/1062936X.2022.2025614 [Google Scholar] [PubMed] [CrossRef]
Warkany J (1971). Congenital Malformations: Notes and Comments. Chicago: Year Book Medical Publishers. [Google Scholar]
Warkany J (1977). Congenital malformations in the past, pp. 5–17. Netherlands: Springer. https://doi.org/10.1007/978-94-011-6621-8_1 [Google Scholar] [CrossRef]
WHO (2023). Congenital disorders. https://www.who.int/news-room/factsheets/detail/birth-defects (accessed on 05/05/2023) [Google Scholar]
Wilson JG (1959). Experimental studies on congenital malformations. Journal of Chronic Diseases 10: 111–130. https://doi.org/10.1016/0021-9681(59)90026-8 [Google Scholar] [PubMed] [CrossRef]
Wilson JG (1973). Environment and Birth Defects Environment and Birth Defects. New York, NY: Academic Press Location. [Google Scholar]
Xie J, Wettschurack K, Yuan C (2020). In vitro Cell platform for understanding developmental toxicity. Frontiers in Genetics 11: 623117. https://doi.org/10.3389/fgene.2020.623117 [Google Scholar] [PubMed] [CrossRef]
Zacharias F, Antonios K, Panos A, Theodora M, Asimina V, George D, Emmanuel KN (2023). Intrauterine growth restriction due to gestational diabetes: From pathophysiology to diagnosis and management. Medicina 59: 1139. https://doi.org/10.3390/medicina59061139 [Google Scholar] [PubMed] [CrossRef]
Zimmer B, Kuegler PB, Baudis B, Genewsky A, Tanavde V, Koh W, Tan B, Waldmann T, Kadereit S, Leist M (2011a). Coordinated waves of gene expression during neuronal differentiation of embryonic stem cells as basis for novel approaches to developmental neurotoxicity testing. Cell Death & Differentiation 18: 383–395. https://doi.org/10.1038/cdd.2010.109 [Google Scholar] [PubMed] [CrossRef]
Zimmer B, Schildknecht S, Kuegler PB, Tanavde V, Kadereit S, Leist M (2011b). Sensitivity of dopaminergic neuron differentiation from stem cells to chronic low-dose methylmercury exposure. Toxicological Sciences 121: 357–367. https://doi.org/10.1093/toxsci/kfr054 [Google Scholar] [PubMed] [CrossRef]
Cite This Article
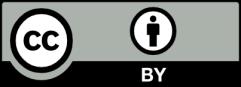