Open Access
ARTICLE
MiR-194-5p suppresses the warburg effect in ovarian cancer cells through the IGF1R/PI3K/AKT axis
1 School of Basic Medicine, Ningxia Medical University, Yinchuan, 750004, China
2 The Fifth People’s Hospital of Ningxia, Shizuishan, 753000, China
* Corresponding Author: RU BAI. Email:
(This article belongs to the Special Issue: Non-Coding RNAs in the Regulation of Human Cancers)
BIOCELL 2023, 47(3), 547-554. https://doi.org/10.32604/biocell.2023.025048
Received 19 June 2022; Accepted 06 October 2022; Issue published 03 January 2023
Abstract
Background: The Warburg effect is considered as a hallmark of various types of cancers, while the regulatory mechanism is poorly understood. Our previous study demonstrated that miR-194-5p directly targets and regulates insulin-like growth factor1 receptor (IGF1R). In this study, we aimed to investigate the role of miR-194-5p in the regulation of the Warburg effect in ovarian cancer cells. Methods: The stable ovarian cell lines with miR-194-5p overexpression or silencing IGF1R expression were established by lentivirus infection. ATP generation, glucose uptake, lactate production and extracellular acidification rate (ECAR) assay were used to analyze the effects of aerobic glycolysis in ovarian cancer cells. Gene expression was analyzed by quantitative polymerase chain reaction (qPCR) and western blot. Immunohistochemistry assays were performed to assess the expression of the IGF1R protein in ovarian cancer tissues. Results: Overexpression of miR-194-5p or silencing IGF1R expression in ovarian cancer cells decreases ATP generation, glucose uptake, lactate production, and ECAR and inhibits both the mRNA and protein expression of PKM2, LDHA, GLUT1, and GLUT3. While the knockdown of miR-194-5p expression led to opposite results. Overexpression of miR-194-5p or silencing IGF1R expression suppressed the phosphatidylinositol-3-kinase/protein kinase B (PI3K/AKT) pathway, whose activation can sustain aerobic glycolysis in cancer cells, and the knockdown of miR-194-5p expression promoted the activation of the PI3K/AKT pathway. Conclusion: Our results suggest that miR- 194-5p can inhibit the Warburg effect by negative regulation of IGF1R and further repression of the PI3K/AKT pathway, which provides a theoretical basis for further test of miR-194-5p as a target in the treatment of ovarian cancer.Keywords
Ovarian cancer is a highly malignant tumor and is the number one killer among cancers of the female reproductive system that seriously threatens the health of women (Amin et al., 2019; Cabasag et al., 2020). Tumor reduction surgery combined with chemotherapy is widely applied in the treatment of ovarian cancer, while the five-year relative survival is less than 50% due to recurrence and drug resistance (Amin et al., 2019; Torre et al., 2018; Coburn et al., 2017; Tsibulak et al., 2019). Therefore, the development of new methods in the prognosis and treatment of ovarian cancer is of great importance.
Tumor cells rely on abnormal energy metabolism to promote rapid cell growth, invasion, and metastasis. Aerobic glycolysis is the common energy metabolic feature of cancer cells, and cancer cells provide energy by enhancing anaerobic fermentation (Hanahan and Weinberg, 2011). Tumor cells consume large amounts of glucose, and the glucose transporter (GLUT) transports glucose into tumor cells for glycolysis. After glucose is metabolized to pyruvate, aerobic oxidation occurs through lactate dehydrogenase (LDH), and pyruvate is catalyzed into lactic acid instead of the mitochondrial tricarboxylic acid cycle (Park et al., 2020; Vazquez et al., 2016), which was first observed by Warburg and named as the Warburg effect.
MicroRNAs (miRNAs) are endogenous short RNA of 20 to 24 nucleotides long that are widely expressed in eukaryotes and can induce the degradation of target mRNA or inhibit its translation by complementary pairing with target mRNA (Saliminejad et al., 2019; Vishnoi and Rani, 2017; He and Hannon, 2004). Accumulating evidence suggested that miRNAs are extensively involved in the regulation of ovarian cancer progression and development (Ghafouri-Farda et al., 2020). However, the regulatory mechanism of miRNA and the Warburg effect in ovarian cancer remains to be investigated.
In our study, we discovered that miR-194-5p affects the aerobic glycolysis of ovarian cancer cells, by which miR-194-5p suppresses aerobic glycolysis via the IGF1R/PI3K/AKT axis. Our finding would provide a rationale for screening the new molecular target of ovarian cancer.
Two human ovarian cancer cell lines, ES-2 and SKOV3, were cultured as previously described (Bai et al., 2020).
Lentivirus infection and establishment of stable cell lines
ES-2 and SKOV3 cells were seeded in a six-well plate overnight and infected with lentiviruses expressing LV-miR-194-5p or LV-miR-194-5p-NC, LV-miR-194-5p-inhibition or LV-miR-194-5p-inhibition-NC, LV-IGF1R-RNAi or LV-IGF1R-RNAi-NC (GeneChem, Shanghai, China). After 72 h of infection, the cells were selected with 2 μg/mL puromycin for two weeks to establish stable cell lines.
RNA isolation and reverse transcription-quantitative polymerase chain reaction (RT-qPCR)
Total RNA was extracted using RNA Easy Fast Tissue/Cell Kit (TianGen, Beijing, China), followed by reverse transcription into cDNA using PrimeScript™ RT reagent Kit (Takara, Dalian, China). TB Green® Premix Ex Taq™ II (Takara, Dalian, China) was used to run qPCR. The primer sequences (listed in Table 1) were designed and synthesized by Sangon Biotech (Shanghai, China). The expression of miRNA or mRNA was quantified by the 2–ΔΔCT method, using U6 as an internal reference gene for microRNA assays and β-actin as an internal reference gene for gene assays.
The cells were lysed and separated by sodium dodecyl sulfate-polyacrylamide gel electrophoresis. The protein was transferred to a polyvinylidene fluoride membrane and blocked using a blocking buffer. Then, the membranes were incubated with monoclonal primary antibodies respectively, including anti-insulin-like growth factor1 receptor (IGF1R; CST, USA), anti-phosphorylation of serine/threonine kinase (p-AKT; S473, CST, USA), anti-AKT (CST, USA) or β-actin (Bioss, Beijing, China). For the detection of the key genes or enzymes involved in glycolysis, we used polyclonal antibodies, including anti-pyruvate kinase M2 (anti-PKM2), anti-lactate dehydrogenase A (anti-LDHA), anti-glucose transporter 1 and 3 (anti-GLUT1, and GLUT3) (Proteintech, Wuhan, China). After washing, the membranes were incubated with horseradish peroxidase-conjugated secondary antibody (Bioss, Beijing, China) and developed using the enhanced chemiluminescence kit (NCM Biotech, Suzhou, China). The signals were captured by Amersham Imager 600 (USA). The intensity of reference gene β-actin served as the loading control.
The paraffin sections of ovarian cancer tissue were soaked in a series of xylene and graded alcohols and incubated in the boiling buffer containing ethylenediaminetetraacetic acid for antigen retrieval. To inhibit endogenous peroxidase, the sections were incubated in 3% H2O2 solution for 8 min and blocked with goat serum. Then the sections were incubated with a primary antibody against IGF1R (Bioss, Beijing, China), followed by incubation with a secondary antibody (Bioss, Beijing, China). After hematoxylin staining, tissue sections were soaked in an alcohol gradient, followed by soaking in xylene. The images were captured by a microscope, and the target signals were quantified by the Image-J software (USA).
The concentration of ATP was examined using the Enhanced ATP Assay Kit (Beyotime, Shanghai, China). Each well of a twelve-well plate was seeded with 8 × 104 cells and lysed for 42 h. The 96-well plate was preincubated with 100 μL ATP assay buffer for 5 min, and 10 μL of supernatants were added for the determination of ATP concentration. The absorbance was read by a multifunctional microplate reader (PerkinElmer, USA) and calculated by substituting the assay values into the standard curve. The linear range of the assay was between 0.1 nM to 10 µM.
Glucose uptake was detected by Glucose Uptake Colorimetric Assay Kit (Biovision, USA). The cells were cultured overnight in a growth medium without serum and 100 μL of Krebs-Ringer-Phosphate-Hepes buffer containing 2% bovine serum albumin for 40 min. The cells were treated with 0.1 mg/mL insulin to activate the glucose transporter and 10 mM 2-deoxy-D-glucose (2-DG) for 20 min separately. To degrade endogenous NAD(P) and denature enzymes, cells were lysed in 90 μL extraction buffer at 90°C for 40 min and cooled down on the ice for 5 min. Then, neutralization buffer (12 mL) was mixed with 38 μl of Mix B and added to each well, and the absorbance was measured at 412 nm at 37°C by the multifunctional microplate reader. The standard glucose uptake curve was plotted using the standard well absorbance value, and then the glucose uptake level was calculated.
Assessment of lactate production
The colorimetric lactate kit (Solarbio, Beijing, China) was used to measure lactate concentration. Cells (5 × 106 cells) were lysed in 1 mL of extraction buffer I through ultrasonic disruption and then centrifuged. Supernatants (700 μL) and extraction buffer II (150 μL) were mixed, followed by centrifugation at 10,000 g at 4°C for 10 min. Then, supernatants (10 μL) were mixed with assay buffer for 20 min at 37°C. The absorbance at 570 nm was read by a multifunctional microplate reader. The standard lactate solution curve was obtained by diluting 2.5, 1.25, 0.625, 0.3125, 0.15625, and 0.078 µmol/mL of the lactate standard into the 96-well plate. The linear range of the assay was between 0.05 mM to 20 mM.
Extracellular acidification rate (ECAR) assays
Seahorse XF Glycolysis Stress Test Kit (Agilent, USA) was used to examine ECAR. Then, in the XFe24 microplate, (2 × 104) cells were seeded. The cartridge sensor was hydrated overnight in a CO2-free incubator. Before detection with seahorse, the cells were treated with 2 mM glutamine for 1 h in a CO2-free incubator. Glucose (100 mM), oligomycin (100 µM), and 2-DG (500 mM) were added sequentially into each well and detected through Seahorse XFe24 Analyzer (Agilent, USA).
Data from at least three independent experiments were expressed as the mean ± SD. The data were analyzed by SPSS 26.0 software with the two-tailed Student’s t-test; p < 0.05 was considered statistically significant.
MiR-194-5p weakens the warburg effect in ovarian cancer cells
The tumor cells have a higher glucose uptake rate and produce more ATP and lactate than normal cells because of the Warburg effect. To detect the role of miR-194-5p in the Warburg effect in ovarian cancer cells, we established ovarian cancer cells with stable overexpression of miR-194-5p or its knockdown by lentivirus infection (Fig. 1A). Further measurements showed that miR-194-5p overexpression decreases ATP generation (Fig. 1B), glucose uptake (Fig. 1C), and lactate production (Fig. 1D), whereas knocked down of miR-194-5p expression exhibited the opposite effect. Consistently, examination of glycolytic metabolism showed that ECAR and maximum glycolytic capacity are significantly decreased in the cells with miR-194-5p overexpression, while downregulation of miR-194-5p expression increased ECAR and maximum glycolytic capacity (Figs. 1E and 1F). These data thus indicated that miR-194-5p suppresses the Warburg effect in ovarian cancer cells.
Figure 1: miR-194-5p inhibits the Warburg effect in ovarian cancer cells. (A) qPCR to analyze miR-194-5p expression in stable ovarian cell lines with overexpression of miR-194-5p or knocked down miR-194-5p expression. U6 was used as the internal control. (B–F) Quantification of ATP (B), level of glucose uptake (C), lactate concentration (D), ECAR (E), and glycolytic capacity (F) in the ovarian cells with miR-194-5p overexpression or miR-194-5p expression knocked down. (*P < 0.05; **P < 0.01, ***P < 0.001). ECAR: extracellular acidification rate; qPCR: quantitative polymerase chain reaction.
MiR-194-5p suppresses the glycolytic gene expression in ovarian cancer cells
Many key enzymes or genes of glycolytic metabolism are altered by the Warburg effect. Therefore, we assessed the mRNA and protein expression of PKM2, LDHA, GLUT1, and GLUT3 in ovarian cancer cells when miR-194-5p was overexpressed or its expression was knocked down. PKM2 determines whether glucose is channeled into the lactate-producing pathway. LDHA is a step-controlling enzyme that controls the last step of glycolysis by mediating the interconversion of pyruvate and lactate. GLUT1 and GLUT3 are two important glucose transporters involved in glycolysis in tumor cells (Vaupel and Multhoff, 2021). We observed that miR-194-5p overexpression reduces the mRNA (Figs. 2A–2D) and protein (Fig. 2E) expression of PKM2, LDHA, GLUT1, and GLUT3, whereas knockdown of miR-194-5p expression gives the opposite results.
Figure 2: miR-194-5p mediates the expression of key glycolytic genes in ovarian cancer cells. (A–D) The mRNA expression of PKM2(A), LDHA(B), GLUT1(C), and GLUT3 (D) in the ovarian cells with miR-194-5p overexpression or miR-194-5p expression knocked down was detected by qPCR. β-actin was used as the internal control. (E) The protein expression of PKM2, LDHA, GLUT1, and GLUT3 in the ovarian cells with miR-194-5p overexpression or miR-194-5p expression knocked down was examined by western blotting. (*P < 0.05; **P < 0.01, ***P < 0.001). PKM2: pyruvate kinase M2; LDHA: lactate dehydrogenase A; GLUT: glucose transporter.
Insulin-like growth factor1 receptor promotes the warburg effect in ovarian cancer cells
In our previous studies, we showed that IGF1R is the direct target of miR-194-5p (Bai et al., 2020). Immunohistochemical analysis revealed that compared with normal ovarian tissues, IGF1R expression increased in ovarian cancer tissues (Fig. 3A). To investigate the role of IGF1R in the Warburg effect, we knocked down IGF1R expression in ovarian cancer cells (Fig. 3B) and found a decrease in ATP generation (Fig. 3C), glucose uptake (Fig. 3D), lactate production (Fig. 3E) and ECAR level (Figs. 3F and 3G). In agreement with that, ovarian cancer cells with reduced IGF1R expression showed decreased expression of PKM2, LDHA, GLUT1, and GLUT3 mRNA and proteins (Figs. 3H and 3I). These results indicate that IGF1R promotes the Warburg effect in ovarian cancer cells.
Figure 3: IGF1R enhances the Warburg effect in ovarian cancer cells. (A) IGF1R expression in ovarian cancer tissues detected by immunohistochemical analysis. (B) The protein expression of IGF1R was examined by western blotting in the ovarian cells with knocked-down IGF1R expression. (C–F) Quantification of ATP (C), level of glucose uptake (D), lactate concentration (E), ECAR (F), and glycolytic capacity (G) in the ovarian cells with inhibition of IGF1R expression. (H–I) The mRNA expression (H) and protein expression (I) of PKM2, LDHA, GLUT1, and GLUT3 in the ovarian cells with inhibition of IGF1R expression. (*P < 0.05; **P < 0.01, ***P < 0.001). IGF1R: insulin-like growth factor1 receptor; PKM2: pyruvate kinase M2; LDHA: lactate dehydrogenase A; GLUT: glucose transporter.
MiR-194-5p regulates the insulin-like growth factor1 receptor/phosphatidylinositol-3-kinase/protein kinase B (IGF1R/PI3K/AKT) pathway in ovarian cancer cells
IGF1R and its downstream PI3K-AKT pathway serve as important regulators in the energy metabolism in tumor cells (Amutha and Rajkumar, 2017). To clarify whether the function of miR-194-5p on the Warburg effect is related to the IGF1R/PI3K/AKT signaling pathway, we tested AKT activation when IGF1R expression was knocked-down in ovarian cancer cells. The level of p-AKT decreased in the cells with reduced expression of IGF1R (Fig. 4A). We further examined whether miR-194-5p contributes to the IGF1R/PI3K/AKT pathway and found that IGF1R expression and AKT activation decreased with miR-194-5p overexpression. In contrast, the knockdown of miR-194-5p expression increased the expression of these proteins (Fig. 4B). These results further suggest that miR-194-5p mediates the PI3K/AKT pathway by targeting IGF1R in ovarian cancer cells. Taken together, our result reveals that miR-194-5p suppresses the Warburg effect in ovarian cancer cells through the PI3K/AKT signaling pathway by targeting IGF1R (Fig. 4C).
Figure 4: miR-194-5p suppresses the IGF1R/PI3K/AKT pathway. (A) The protein level of AKT and p-AKT were detected by western blotting in the ovarian cells with IGF1R expression knocked down. (B) The protein level of IGF1R, p-AKT, and AKT in the ovarian cells overexpressing miR-194-5p or with knocked-down miR-194-5p expression. (C) The diagram of the mechanism of miR-194-5p regulates the Warburg effect in ovarian cancer cells. IGF1R/PI3K/AKT: insulin-like growth factor1 receptor/phosphatidylinositol-3-kinase/protein kinase B.
In recent years, more studies have demonstrated that the Warburg effect plays a vital role in tumor cell progression, metastasis, and chemotherapy resistance (Vaupel et al., 2019; Icard et al., 2018). Therefore, blocking the Warburg effect is a new idea of molecular therapy against cancer (Pascale et al., 2020). However, the regulons and regulatory mechanism of the Warburg effect in tumor cells still need further investigation. MiRNAs serve as a key regulator of mRNA expression at the post-transcription level, and miRNAs are widely dysregulated in various types of cancer cells (Hill and Tran, 2021). A recent study supported that miRNAs control cancer-associated glycolytic processes through interaction with the key enzymes of glycolysis and cellular signaling pathway in cancers such as ovarian cancer (Zhang and Liu, 2022). For example, the expression of miR-203 is increased in ovarian cancer and promotes aerobic glycolysis by directly targeting the pyruvate dehydrogenase B (Zhao et al., 2016). Besides, miR-383 inhibits ovarian cancer cell proliferation, invasion, and glycolysis through the negative regulation of lactate dehydrogenase A (Han et al., 2017). MiR-603 overexpression suppresses the Warburg effect, and hexokinase-2 was identified as a target of miR-603 in ovarian cancer cells (Lu et al., 2019). In our study, upregulation of miR-194-5p in ovarian cancer cells suppresses the Warburg effect by decreasing ATP generation, glucose uptake, lactate production, and ECAR. This suggests that miR-194-5p contributes to the glycolytic metabolism of ovarian cancer cells. We further illustrated that miR-194-5p overexpression could suppress the PI3K/AKT signaling activity by negative regulation of IGF1R and inhibition of aerobic glycolysis in ovarian cancer cells.
IGF1R is frequently overexpressed in several tumor types, including ovarian cancer (Somri-Gannam et al., 2020; Zorea et al., 2018). The treatment of ovarian cancer cells with IGF1R monoclonal antibodies can significantly suppress the activity of IGF1R, resulting in cell growth inhibition and sensitivity to chemotherapy (Zhang et al., 2021). IGF1R binds to its ligands IGF1 or IGF2 and activates the PI3K/AKT signaling pathway (Werner and Sarfstein, 2014). Accumulated evidence suggests that the PI3K/AKT signaling is over-activated in human malignant tumors, in which glycolysis is increased by the upregulation of metabolic enzymes (Ediriweera et al., 2019). Furthermore, AKT activity increased in 40% of breast and ovarian cancers (Liu et al., 2018). AKT activity improves the accumulation of GLUT1 on the cell membrane and thus increases the uptake of glucose, which in turn promotes the Warburg effect (Hosios and Manning, 2021). Consistently, we revealed that miR-194-5p significantly suppresses IGF1R expression and AKT activity, which maybe, would result in the inhibition of aerobic glycolysis by miR-194-5p in ovarian cancer cells.
In conclusion, the present study revealed the role of miR-194-5p and its target gene, IGF1R, on aerobic glycolysis in ovarian cancer cells. MiR-194-5p suppressed aerobic glycolysis and negatively regulated IGF1R and the PI3K/AKT pathway. IGF1R promotes glycolysis in ovarian cancer cells. Therefore, miR-194-5p regulates aerobic glycolysis of ovarian cancer cells, possibly via IGF1R, which in turn affects the PI3K/AKT signaling pathway, suggesting the potential of miR-194-5p as a target in the treatment of ovarian cancer.
Availability of Data and Materials: All data generated or analyzed during this study are included in this published article.
Author Contribution: Study conception and design: Ru Bai; data collection: Lijun Du and Kaikai Dou; analysis and interpretation of results: Lijun Du, Kaikai Dou, and Nianhai Liang; draft manuscript preparation: Lijun Du, Ru Bai, Jianmin Sun. All authors reviewed the results and approved the final version of the manuscript.
Ethics Approval: This study was approved by the Ethical Committee of Ningxia Medical University (Protocol No. 2020-007). The use of 25 fixed ovarian cancer tissues and 5 fixed normal ovarian tissues was approved by the Department of Pathology, General Hospital of Ningxia Medical University.
Funding Statement: This work was supported by the Nature Science Foundation of Ningxia (2020AAC03162).
Conflicts of Interest: The authors declare that they have no conflicts of interest to report regarding the present study.
References
Amin RW, Ross AM, Lee J, Guy J, Stafford B (2019). Patterns of ovarian cancer and uterine cancer mortality and incidence in the contiguous USA. Science of the Total Environment 697: 134128. DOI 10.1016/j.scitotenv.2019.134128. [Google Scholar] [CrossRef]
Amutha P, Rajkumar T (2017). Role of insulin-like growth factor, insulin-like growth factor receptors, and insulin-like growth factor-binding proteins in ovarian cancer. Indian Journal of Medical and Paediatric Oncology 38: 198–206. DOI 10.4103/ijmpo.ijmpo_3_17. [Google Scholar] [CrossRef]
Bai R, Dou K, Wu Y, Ma Y, Sun J (2020). The NF-κB modulated miR-194-5p/IGF1R/PPFIBP axis is crucial for the tumorigenesis of ovarian cancer. Journal of Cancer 11: 3433–3445. DOI 10.7150/jca.40604. [Google Scholar] [CrossRef]
Cabasag CJ, Arnold M, Butler J, Inoue M, Trabert B, Webb PM, Bray F, Soerjomataram I (2020). The influence of birth cohort and calendar period on global trends in ovarian cancer incidence. International Journal of Cancer 146: 749–758. DOI 10.1002/ijc.32322. [Google Scholar] [CrossRef]
Coburn SB, Bray F, Sherman ME, Trabert B (2017). International patterns and trends in ovarian cancer incidence, overall and by histologic subtype. International Journal of Cancer 140: 2451–2460. DOI 10.1002/ijc.30676. [Google Scholar] [CrossRef]
Ediriweera MK, Tennekoon KH, Samarakoon SR (2019). Role of the PI3K/AKT/mTOR signaling pathway in ovarian cancer: Biological and therapeutic significance. Seminars in Cancer Biology 59: 147–160. DOI 10.1016/j.semcancer.2019.05.012. [Google Scholar] [CrossRef]
Ghafouri-Farda S, Shooreib H, Taheric M (2020). miRNA profile in ovarian cancer. Experimental and Molecular Pathology 113: 104381. DOI 10.1016/j.yexmp.2020.104381. [Google Scholar] [CrossRef]
Hanahan D, Weinberg RA (2011). Hallmarks of cancer: The next generation. Cell 144: 646–674. DOI 10.1016/j.cell.2011.02.013. [Google Scholar] [CrossRef]
Han RL, Wang FP, Zhang PA, Zhou XY, Li Y (2017). miR-383 inhibits ovarian cancer cell proliferation, invasion and aerobic glycolysis by targeting LDHA. Neoplasma 64: 244–252. DOI 10.4149/neo_2017_211. [Google Scholar] [CrossRef]
He L, Hannon GJ (2004). MicroRNAs: Small RNAs with a big role in gene regulation. Nature Reviews Genetics 5: 522–531. DOI 10.1038/nrg1379. [Google Scholar] [CrossRef]
Hill M, Tran N (2021). miRNA interplay: Mechanisms and consequences in cancer. Disease Models & Mechanisms 14: 1–9. DOI 10.1242/dmm.047662. [Google Scholar] [CrossRef]
Hosios AM, Manning BD (2021). Cancer signaling drives cancer metabolism: AKT and the warburg effect. Cancer Research 81: 4896– 4898. DOI 10.1158/0008-5472.CAN-21-2647. [Google Scholar] [CrossRef]
Icard P, Shulman S, Farhat D, Steyaert JM, Alifano M, Lincet H (2018). How the Warburg effect supports aggressiveness and drug resistance of cancer cells? Drug Resistance Updates 38: 1–11. DOI 10.1016/j.drup.2018.03.001. [Google Scholar] [CrossRef]
Liu HW, Bi WT, Huang HT, Li RX, Xi Q, Feng L, Bo W, Hu M, Wen WS (2018). Satb1 promotes Schwann cell viability and migration via activation of PI3K/AKT pathway. European Review for Medical and Pharmacological Sciences 22: 4268–4277. DOI 10.26355/eurrev_201807_15423. [Google Scholar] [CrossRef]
Lu J, Wang L, Chen W, Wang Y, Zhen S, Chen H, Cheng J, Zhou Y, Li X, Zhao L (2019). miR-603 targeted hexokinase-2 to inhibit the malignancy of ovarian cancer cells. Archives of Biochemistry and Biophysics 661: 1–9. DOI 10.1016/j.abb.2018.10.014. [Google Scholar] [CrossRef]
Park JH, Pyun WY, Park HW (2020). Cancer metabolism: Phenotype, signaling and therapeutic targets. Cells 9: 2308. DOI 10.3390/cells9102308. [Google Scholar] [CrossRef]
Pascale RM, Calvisi DF, Simile MM, Feo CF, Feo F (2020). The Warburg effect 97 years after its discovery. Cancers 12: 2819. DOI 10.3390/cancers12102819. [Google Scholar] [CrossRef]
Saliminejad K, Khorram Khorshid HR, Soleymani Fard S, Ghaffari SH (2019). An overview of microRNAs: Biology, functions, therapeutics, and analysis methods. Journal of Cellular Physiology 234: 5451–5465. DOI 10.1002/jcp.27486. [Google Scholar] [CrossRef]
Somri-Gannam L, Meisel-Sharon S, Hantisteanu S, Groisman G, Limonad O, Hallak M, Bruchim I (2020). IGF1R axis inhibition restores dendritic cell antitumor response in ovarian cancer. Translational Oncology 13: 100790. DOI 10.1016/j.tranon.2020.100790. [Google Scholar] [CrossRef]
Torre LA, Trabert B, DeSantis CE, Miller KD, Samimi G, Runowicz CD, Gaudet MM, Jemal A, Siegel RL (2018). Ovarian cancer statistics, 2018. CA: A Cancer Journal for Clinicians 68: 284–296. DOI 10.3322/caac.21456. [Google Scholar] [CrossRef]
Tsibulak I, Zeimet AG, Marth C (2019). Hopes and failures in front-line ovarian cancer therapy. Critical Reviews in Oncology/Hematology 143: 14–19. DOI 10.1016/j.critrevonc.2019.08.002. [Google Scholar] [CrossRef]
Vaupel P, Multhoff G (2021). Revisiting the Warburg effect: Historical dogma vs. current understanding. The Journal of Physiology 599: 1745–1757. DOI 10.1113/JP278810. [Google Scholar] [CrossRef]
Vaupel P, Schmidberger H, Mayer A (2019). The Warburg effect: Essential part of metabolic reprogramming and central contributor to cancer progression. International Journal of Radiation Biology 95: 912–919. DOI 10.1080/09553002.2019.1589653. [Google Scholar] [CrossRef]
Vazquez A, Kamphorst JJ, Markert EK, Schug ZT, Tardito S, Gottlieb E (2016). Cancer metabolism at a glance. Journal of Cell Science 129: 3367–3373. DOI 10.1242/jcs.181016. [Google Scholar] [CrossRef]
Vishnoi A, Rani S (2017). MiRNA biogenesis and regulation of diseases: An overview. Methods in Molecular Biology 1509: 1–10. DOI 10.1007/978-1-4939-6524-3. [Google Scholar] [CrossRef]
Werner H, Sarfstein R (2014). Transcriptional and epigenetic control of IGF1R gene expression: Implications in metabolism and cancer. Growth Hormone & IGF Research 24: 112–118. DOI 10.1016/j.ghir.2014.03.006. [Google Scholar] [CrossRef]
Zhang C, Liu N (2022). Noncoding RNAs in the glycolysis of ovarian cancer. Frontiers in Pharmacology 13: 855488. DOI 10.3389/fphar.2022.855488. [Google Scholar] [CrossRef]
Zhang W, Xie Y, Wang W, Jia J, Bai J, Zhang R, Wang C, Guo R (2021). IGF-1R anti-idiotypic antibody antagonist exhibited anti-ovarian cancer bioactivity and reduced cisplatin resistance. Human Cell 3: 1197–1214. DOI 10.1007/s13577-021-00535-x. [Google Scholar] [CrossRef]
Zhao X, Fan L, Xie N, Zou K, Xiao X, Wang S (2016). MiR-203 promotes the growth and migration of ovarian cancer cells by enhancing glycolytic pathway. Tumor Biology 37: 14989–14997. DOI 10.1007/s13277-016-5415-1. [Google Scholar] [CrossRef]
Zorea J, Prasad M, Cohen L, Li N, Schefzik R, Ghosh S, Rotblat B, Brors B, Elkabets M (2018). IGF1R upregulation confers resistance to isoform-specific inhibitors of PI3K in PIK3CA-driven ovarian cancer. Cell Death & Disease 9: 944. DOI 10.1038/s41419-018-1025-8. [Google Scholar] [CrossRef]
Cite This Article
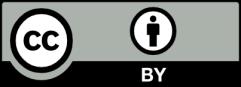