Open Access
REVIEW
Applications of Microalgae in Five Areas of Biotechnology
1 Facultad de Ciencias Agrotecnológicas, Universidad Autónoma de Chihuahua, Chihuahua, 31000, Mexico
2 Facultad de Zootecnia y Ecología, Universidad Autónoma de Chihuahua, Chihuahua, 33820, Mexico
* Corresponding Author: Ofelia Adriana Hernández-Rodríguez. Email:
Phyton-International Journal of Experimental Botany 2023, 92(10), 2737-2759. https://doi.org/10.32604/phyton.2023.029851
Received 11 March 2023; Accepted 19 June 2023; Issue published 15 September 2023
Abstract
Microalgae are mostly photoautotrophic microscopic organisms. According to their cellular structure, they are classified into two types, eukaryotes, and prokaryotes, and they are distributed in all types of ecosystems, presenting unique qualities due to the fact that they synthesize high value-added molecules used in various productive and environmental activities, and because their biomass is used as raw material to obtain various products. Therefore, the objective of this review was to collect, organize, and collate current information on the use of microalgae in the development of biotechnology involving the areas of agriculture, health, food, bioremediation, and biofuels. The results show that the microalga Chlorella stands out for its multiple uses in the five areas considered in this study. The search for healthier and more environmentally friendly technologies has contributed to the development of new biotechnological applications that require the study and incorporation of specialized microorganisms to replace and/or improve existing applications.Keywords
Microalgae are a large group of microorganisms, mostly photoautotrophic, that possess, like higher plants, the ability to transform light energy into chemical energy, producing 50% of the assimilable oxygen and with a carbon dioxide fixation of 10 to 50 times faster [1–3].
These microorganisms are present in any type of ecosystem, terrestrial and aquatic, due to their large tolerance ranges to salinity, temperature, and pH [1]. Two cell types are recognized, prokaryotes such as cyanobacteria and those with eukaryotic cell structures such as green algae and diatoms [4].
On the other hand, biotechnology can be defined as a set of techniques that take advantage of the physiological properties of living beings for the production of goods and services [5]. For its part, reference [6] explained biotechnology as any technological application that uses biological systems and living organisms or their derivatives for the creation or modification of products or processes for specific uses.
The use of a color index to classify biotechnology areas can be a useful guide to promote public perception and understanding of its applications. This index can be adapted or incorporated into more colors as it develops over time [7]. Table 1 presents a proposal for a color index for its classification.
Biotechnology is not a single and limited study discipline but depends on multidisciplinary knowledge of various subjects such as biology, chemistry, mathematics, and engineering [8]. Within the field of current biotechnology, microalgae are considered microorganisms of great interest for obtaining high-value-added products due to their physiological characteristics and biomass production [9].
This gives rise to numerous applications, such as the introduction of new biofertilizers for crops [10], the development of vaccines and anticancer compounds [11], the development of fresh, improved food [12], sustainable animal breeding [13], the production of new fuel sources [14], etc. Therefore, the objective of this review was to collect, organize and collate current information on the use of microalgae in biotechnological developments involving agriculture, health, food, bioremediation, and biofuels.
This document was generated by reviewing the literature obtained by using the keywords: microalga applications, biotechnological applications of microalgae, microalgae, microalgae applications, microalgae in biotechnology, microalgae, microalgae use, and biotechnological uses of microalgae, using the databases: ACS Publications, Dialnet, ELSEVIER, Google Scholar, IOPscience, MDPI, PLOS ONE, SciELO, ScienceDirect, Springer Nature, Taylor & Francis, and Wiley Online Library (Fig. 1). Those research articles whose publication was not older than 2016 were selected to document some of the most current biotechnological applications.
Figure 1: Information on the search methodology
The study of microalgae with a scientific approach began in the 1890s with the Dutch microbiologist Beijerinck establishing pure cultures of Chlorella vulgaris, which was used in 1919 to study photosynthesis; but it was not until World War II that scientists began to study the physiology and biochemistry of microalgae for biomass production [15]. Harder et al. [16], as well as Milner [17], proposed these microorganisms as a source of lipids for use as food and in fuel production, in what was the first approach to their biotechnological applications [18].
It is estimated that there are more than 50,000 species of microalgae, of which only approximately 30,000 have been studied and analyzed [19]. The different physiological and metabolic characteristics of microalgae have attracted the attention of researchers as they present application opportunities with diverse benefits. Biotechnological uses can be classified according to the area where they are applied, some examples are applications in agriculture [20], health [11], food [21], bioremediation [22], and biofuel production [14], among others.
The potential of microalgae in agriculture is varied since the production of specialized metabolites helps the development of the field and promotes a balance in the ecosystems [20]. The various applications of microalgae in agriculture are presented in Table 2 and can be grouped into three divisions in terms of their use: in hydroponic co-culture [23], as biofertilizers [24], and in conjunction with chemical fertilizers [25].
Among the applications of microalgae with plants of commercial interest in hydroponic co-culture in greenhouses, there is the research by Bharti et al. [26], who planted Chrysanthemum stems in the culture medium BG110 nitrogen free added with the microalgae Anabaena laxa and Anabaena torulosa, thus promoting the growth of the chrysanthemum. Likewise, Zhang et al. [27] used the modified Hoagland medium inoculated with the Chlorella sp. microalgae, obtaining, as a result, greater growth of the tomato plant compared to the control of the experiment that only contained the culture medium.
Similarly, Barone et al. [23] used the Hoagland medium together with the microalgae Chlorella vulgaris and Scenedesmus quadricauda, which promoted the growth of the tomato plant.
On the other hand, Mera et al. [32] used lettuce plants of the crespa variety in a common hydroponic solution, containing 34 g of monoammonium phosphate, 208 g of calcium nitrate, and 110 g of potassium nitrate per liter, added with the microalga Scenedesmus sp., reaching the result that the microalgae solutions increased the aerial weight of the plant.
The use of microalgae as biofertilizers has given promising results, Hernández-Reyes et al. [20] demonstrated that a consortium of microalgae composed of Anabaena sp., Leptolyngbya sp., and Synechocystis sp. inoculated in a blue corn crop influenced a higher crop height, a greater number of leaves and a greater amount of protein in grains, this compared with the use of chemical fertilizer based on urea and soluble monoammonium phosphate, and a biofertilizer commercial derivative of Azopyrillum. In addition, the microalgae by remaining in the soil increased the biodiversity of photosynthetic microorganisms, so there was an improvement in it.
Grzesik et al. [24] obtained similar results using triple foliar biofertilization with the microalgae Anabaena sp., Chlorella sp., and Microcystis aureginosa from the willow Salix viminalis previously chemically fertilized with nitrates, polyphosphates, and potassium. This biofertilization increased the stability of cytomembranes, chlorophyll content, net photosynthesis intensity, transpiration, and decreased intracellular carbon dioxide concentration, showing the potential of microalgae to increase willow production.
On the other hand, Godlewska et al. [10] investigated the use of different concentrations of a filtrate of the microalga Spirulina platensis in soaking radish seeds and applying it foliar. Their results showed greater growth and development of the plant, greater production of chlorophyll, and an increase in the microelements of the crop when compared with a control group, in which the treatment applied was a commercial biostimulant based on sodium p-nitrophenolate, ortho-sodium nitrophenolate, and sodium 5-nitroguaiacolate.
Dineshkumar et al. [29] evaluated the growth of a corn crop by applying a combination of cow dung and the microalgae Spirulina platensis and Chlorella vulgaris for 75 days inside a greenhouse. The application of cow dung and microalgae to the soil was evaluated, individually and combined, resulting in the fact that the mixture of microalgae with manure increased the height of the crops, their biochemical and mineral components, as well as the germination capacity of the seeds produced, this in comparison with a control crop.
The ammonium production of the microalga Fischerella TB22 was reported by Martínez-Rosales et al. [31], which on its ninth day of cultivation in the BG110 medium can be used as a biofertilizer in plants that need a high nitrogen supply for their development.
3.1.3 In Combination with Chemical Fertilizers
The combination of microalgae with other products helps the development of crops. Nayak et al. [33] evaluated the growth of rice through the implementation of Scenedesmus sp. together with a chemical fertilizer with the formula N-P-K, 120-50-60 Kg ha-1, in the form of urea, simple superphosphate, and potassium muriate. As a result, they obtained that half the recommended dose of nitrogenous fertilizer, that is, 0.59 g of urea per 10 kg of soil, in combination with 3.66 g of microalgae per 10 kg of soil, produced a higher yield and quality of the crop in comparison with the isolated use of chemical and organic fertilizers.
Chittapun et al. [25] tested the mixture of chemical fertilizers of formulas N-P-K 16-20-0 and 46-0-0, with the microalgae Nostoc commune and Nostoc carneum in rice crops inside pots with 12 kg of soil in a greenhouse. The treatments were: without microalgae or fertilizer as a control; T1, 12 g of N. carneum; T2, 12 g N. commune; T3, 6 g N. carneum and 6 g N. commune; T4, the recommended chemical fertilization of 2 g of 16-20-0 and 0.8 g of 46-0-0; T5, half the recommended dose of fertilizer, and from T6 to T8, half the dose of fertilizer combined with microalgae. The results showed that, compared to the other treatments, T6 to T8 increased the number of grains and the weight per spike, which reduces costs without having negative effects on the growth and cultivation of rice.
On the other hand, de Paula Pereira et al. [30] produced an organo-mineral fertilizer using granulated urea in combination with dry biomass at different proportions of the microalgae Chlorella vulgaris, Didymocystis inermis, Tetradesmus obliquus, and Navicula sp., which were macerated in mortar for mixing and transferred to a tablet mold for fertilizer compaction. The results obtained showed that the tablets with a proportion of 25% microalgal biomass with 75% urea promoted the absorption of nutrients and with this combination, the maximum nitrogen content in the corn crop was reached, in comparison with other proportions.
Maurya et al. [28] used lipid-free biomass of Chlorella variabilis and Lyngbya majuscula to replace the use of nitrogen-based chemical fertilizers in maize cultivation. The treatments were: T1, without fertilizer; T2, chemical fertilizer 120-60-40 kg ha-1 in the form of urea, simple superphosphate, and potassium muriate; T3-T6, 100-75-50 kg ha-1 and 25% biomass of Chlorella variabilis, and T7-T10, 100-75-50 kg ha-1 and 25% biomass of Lyngbya majuscula. They found in response that the grain yield was on par with the treatments that used chemical fertilizers, as well as those that applied microalgae, so both strains can be used totally or partially to reduce the use of synthetic fertilizers.
In recent years, the use of microalgae as agricultural biostimulants has been taking commercial advantage over chemical fertilizers due to the demand for organic products whose production is friendly to the environment, since they are a sustainable alternative in the improvement, production, and protection of agricultural crops by supplying nitrogen in plant-available forms, growth regulators, and high levels of essential macro and micronutrients. However, currently, some limiting factors persist in the agricultural uses of microalgae, such as the selection of the appropriate strain, its cultivation, the design of bioreactors, and its large-scale production, so it is necessary to generate more information on different microalgae strains for their applications in the agricultural area [34].
Some species of microalgae, due to the production of metabolites of interest, have become a new source of treatments against diseases. The applications that are observed in Table 3 have been grouped into four divisions indicating the bioactive compounds involved: microalgae with anticancer potential [35], antimicrobial potential [36], antiviral potential [37], and antioxidant potential [38].
One of the microalgae with therapeutic potential against cancer is Chlorella sorokiniana, which induces mitochondria-mediated apoptosis in non-small cells of lung cancer, in addition to the fact that tumor growth of a subcutaneous xenograft in vivo was inhibited after microalga oral ingestion [35]. In the case of the microalga Phaeodactylum tricornutum, the genes of three plant enzymes were introduced by means of a plasmid to modify a metabolic pathway and thus synthesize betulin and its precursor lupeol, compounds that have shown potential for the treatment of certain types of cancers [11].
Sanjeewa et al. [46] fractionated the microalga Nannochloropsis oculata to obtain sterols, which induced apoptosis of cancer cells in vitro, so this microalga could develop anticancer drugs. Suh et al. [45] evaluated the anticancer properties of the ethanol extract of the microalga Micractinium sp., obtaining as a result that the metabolite may be a potential anticancer agent for targeted therapy of colorectal cancer.
As previously mentioned, the metabolites of some microalgae have potential as treatments against diseases, such as sulfated polysaccharides, which are produced by Chlamydomonas reinhardtii. These were evaluated by Vishwakarma et al. [36] in bacterial cultures and demonstrated their capacity. therapy against infections caused by biofilm-forming bacteria. Da Silva-Ferreira et al. [42] reported that the silver chloride nanoparticles obtained from a Chlorella vulgaris culture, are a promising alternative as antimicrobial agents.
Verdugo-González et al. [47] extracted proteins from Nannochloropsis sp., which were evaluated for their antimicrobial activity, reporting that they inhibit more than 90% of growth from Escherichia coli and Staphylococcus aureus, therefore it represents a peptide extract with bacteriostatic and fungistatic properties. Furthermore, Maadane et al. [43] from the microalgae Tetraselmis sp., Dunaliella salina, Dunaliella sp., Nannochloropsis gaditana, Isochrisis sp., and Phaeodactylum tricornutum, obtained ethanolic extracts that presented antimicrobial activity, in such a way that they are considered as a natural source of bioactive compounds to fight bacteria.
The ability to produce monogalactosyl diacylglyceride from the microalgae Coccomyxa sp., this may mean a novel topical treatment for cold sores, viral keratitis, and anogenital herpes [37]. Chen et al. [39] reported that cold water extracts with the microalga Arthrospira platensis inhibit the formation of viral plaques in a wide range of influenza viruses, including influenza, so these extracts represent a potential treatment against influenza outbreaks.
Reichert et al. [40] also used the microalga Arthrospira platensis, to produce exopolysaccharides for the treatment of koi fish herpesvirus, which suppressed viral replication in vitro in the brain cells of fish even after 22 days of infection. For their part, Singab et al. [48] produced polysaccharides using the microalga Spirulina platensis, which reduced replication by up to 87.6% of the hepatitis C virus and herpes simplex virus, so it has the potential to be used as an antiviral.
Corrêa da Silva et al. [38] measured the production of phenolic compounds from the microalgae Pediastrum boryanum, which exceeded those already reported in the literature by other microalgae. Phenolic compounds can be used in the medical area for the prevention of oxidative processes caused by free radicals [50]. Guevara et al. [44] mixed edible vegetable oil with the biomass of Dunaliella salina, a microalga that contains significant amounts of β-carotenes, which have high antioxidant activity, and showed that edible vegetable oil enriched with the microalga is a source of natural antioxidants [51].
Patil et al. [49] treated rat pups with lipopolysaccharide endotoxin, which can cause increased anxiety, decreased social interactions, and memory impairment in rats, and in a subgroup, lactating mothers were given a diet enriched with Spirulina platensis, which promoted interleukin 1β expression and decreased oxidant parameters after the lipopolysaccharide, so a diet rich in this microalga may protect against neuroinflammation and decreased antioxidant defense in the brain of lactating rat pups.
Other antioxidant properties are those reported by Mohanty et al. [41], who evaluated the efficacy of a diet based on Arthrospira platensis in fish to reduce iron-induced oxidative stress and concluded that the decrease in antioxidant defense induced by excess iron, was neutralized with microalgae supplementation after 28 days due to that it contains phenolic acids, tocopherols, β-carotenes and other vitamins and minerals that exhibit antioxidant properties.
There are microalgae that produce highly nutritional metabolites such as proteins, essential fatty acids, polysaccharides, and vitamins, which, in turn, represent a developing source of bioactive compounds that may be useful in applications for the treatment of diseases [52], including sexually transmitted diseases [53] and against SARS-CoV-2 and COVID-19 [54]. However, most of the information comes from in vitro tests, while in vivo and clinical studies are very scarce, so more research is needed in this field [55].
The use of microalgae in food is very wide since it is not only based on the production of metabolites but also the dry biomass plays a very important role in its applications. Some examples are shown in Table 4, which have been grouped into four divisions according to the type of food that is prepared: the use of microalgae as feed for farmed animals [13], preparation of baked foods [56], the development of fortified foods [57] and the potentiation of the organoleptic characteristics of foods [58].
Altmann et al. [13] reported that the use of Arthrospira platensis biomass can replace the use of soybean-based feed in broilers, likewise, Zaki et al. [21], using the same microalgae, determined that fatty acid-free biomass is a viable option for aquaculture feed. Barraza-Guardado et al. [62] reported that the microalgae Arthrospira sp. and Chlorella sp. can be incorporated into 25% of the food of tilapia fish without affecting its growth, while the microalgae Isochrysis sp. and Nannochloropsis sp. negatively affect the growth of the same fish.
Guldhe et al. [64] described that the biomass of Chlorella sorokiniana has the potential to be applied as feed for farmed animals, as reported by Beigbeder et al. [67] with the microalga Chlorella vulgaris.
Kafyra et al. [66] used an oil based on the Chlorella vulgaris microalgae as a substitute for milk, butter, and eggs to bake bread, which presented improved organoleptic characteristics compared to commercial bread brands. Using the same microalgae, Graça et al. [56] came to the result that the use of a concentration of up to 3.0 g of Chlorella vulgaris per 100 g of moist wheat flour results in a positive impact on the rheological characteristics of the bread dough, and bread with an interesting appearance.
Hossain et al. [68] used dry biomass of Haematococcus pluvialis to obtain flour for the preparation of cookies, which presented potential health benefits, while Uribe-Wandurraga et al. [60] formulated cookies added with Chlorella vulgaris and Arthrospira platensis, which contained a greater number of minerals compared to microalgae-free cookies.
3.3.3 Development of Fortified Foods
There is a production of foods with high nutritional content and food supplements for humans from microalgae, Osorio-Fierros et al. [12] elaborated dietary tablets based on prickly pear and Chlorella sp., which are healthy and of good quality.
The microalga Spirulina sp. can be used in a concentration of 2.6% to make snacks with high nutritional content and sensorial acceptance by consumers as described by Lucas et al. [57]. Uribe-Wandurraga et al. [61] used the microalgae Chlorella vulgaris and Arthrospira platensis for the enrichment of cereal snacks through 3D printing of doughs; however, the use of 3% and 4% of Chlorella vulgaris may not be well accepted by consumers due to the strong green color of the food. Nunes da Silva et al. [69] made liquor from umbu leaves and fruits (Spondias tuberosa Arruda) added with Spirulina sp. to enhance its nutritional value.
3.3.4 Potentiation of the Organoleptic Characteristics of Foods
Hosoglu [58] cultivated the microalgae Chlorella protothecoides, Chlorella vulgaris, Crypthecodinium cohnii, Schizochytrium limacinum, and Tetraselmis chuii to produce aromatic compounds in food. A principal component analysis was carried out, resulting in C. cohnii producing a high level of compounds associated with aromatic notes of sulfury cabbage, fruity, roses, and boiled potato, while S. limacinum produced compounds associated with aromatic notes of mushrooms, cucumbers, and grass. The other three microalgae produced high levels of ketones and terpenes with woody and cereal aromatic notes, so these microalgae could be part of food formulations due to the aromatic compounds they contain.
Proteins produced by microalgae can also act as emulsifiers; Böcker et al. [59] reported that the proteins of the microalga Arthrospira platensis stabilize emulsions efficiently, in the same way, Grossmann et al. [63] reached the same results with the microalga Chlorella protothecoides. Ebert et al. [65] used freeze-dried soluble protein extracts from the microalgae Chlorella sorokiniana and Phaeodactylum tricornutum to evaluate the formation and stability of emulsions, showing high stabilities during 7 days of storage.
The first records of the use of microalgae in food date back approximately 2,000 years when the Chinese used these microorganisms to survive famine. Since then, most of the research on the use of microalgae in food has focused on the production of biomass as a rich source of protein for humans and animals, but as time goes by, research should focus on the improvement of microalgae. production systems to become more diverse and economically competitive [70,71].
3.4 Bioremediation Applications
The removal of contaminants from soils and bodies of water using microalgae is a technique that uses the specific characteristics of each species to carry out bioremediation more efficiently, as can be seen in Table 5. These applications can be grouped into two divisions, the use of microalgae in the remediation of wastewater from human activities [72] and the conditioning and remediation of natural areas [73].
3.4.1 Remediation of Wastewater from Human Activities
Nogueira et al. [80] used Spirulina platensis for the remediation of wastewater from fish farming, while Guldhe et al. [64] implemented this type of water as a culture medium for Chlorella sorokiniana, obtaining as a result the reduction of polluting substances.
The microalga Chlorella vulgaris was used by Lv et al. [76] for the remediation of wastewater from cattle farms, reporting that this microalga is promising in the removal of nutrients in waters with a high concentration of organic substances and nitrogen.
Navarrete-Álava et al. [74] used the microalgae Chlamydomonas sp., Desmodesmus sp., and Chlorella sp., among other treatments, for the remediation of water from shrimp farming, obtaining as a result that the microalgae consortium presented a significant difference with respect to the other treatments since the reduction of polluting compounds. Bioremediation of nitrogen-rich water from human waste was carried out by Leong et al. [78] using Chlorella vulgaris in a mixture of activated sludge, obtaining efficient nitrogen removal as a result, as well as viable biomass production.
Li et al. [22] used the microalgae Cyclotella sp., Navicula pelliculosa, and Scenedesmus sp. For the remediation of odorous sewage, reporting that the microalgae Cyclotella sp. And Navicula pelliculosa presented a higher efficiency of contaminant removal. Cheirsilp et al. [79] encapsulated Nannochloropsis sp. In the sodium alginate and was cultivated in secondary effluents from the palm oil mill, in which removal of nutrients from the medium was observed.
For their part, Pandey et al. [72] isolated the microalgae Chlorella sp., Desmodesmus sp., and Scenedesmus sp. to evaluate their impact on dairy wastewater, obtained as a result that, the isolated microalgae, Chlorella sp. and Scenedesmus sp. presented greater efficiency in the removal of nutrients. The use of Chlorella vulgaris was evaluated by Beigbeder et al. [67] for the bioremediation of wastewater from ethanol production, having as process variables the addition of antibiotics, sterilization of the substrate, pH, light intervals, and agitation speed, the last three being the ones that showed the most noticeable effects on the proliferation of microalgae and the efficiency of nutrient removal in this type of water.
3.4.2 Conditioning and Remediation of Natural Areas
Ortiz-Villota et al. [77] used the microalgae Spirulina maxima, Spirulina platensis, and Chlorella vulgaris to treat eutrophication in the Ubaque lagoon, located in Colombia, by reducing the levels of nitrates, nitrites, and phosphates, reporting the effective removal of said nutrients, where, in addition, Chlorella vulgaris was the most suitable due to its greater ability to adapt to environmental conditions.
According to Park et al. [73] microalgae crusts are considered a solution for soil restoration since they improve their fertility through mineral chelation, dust trapping, and nutrient fixation. The same research demonstrated that the use of the microalgae Nostoc sp., Phormidium sp., and Scytonema arcangeli combined with two chemical soil fixatives: a spray consisting of biopolysaccharides with sticking agents and a super absorbent polymer for water retention with a nutritional supplement, allowed the formation of crusts to combat desertification in arid areas.
Yilmaz et al. [75] studied the effect of different applications of biofertilizers and chemical fertilizers on the stability of soil aggregates, demonstrating that the inoculation of Chlorella sp. Alone or in combination with vermicompost increased the stability of the micro aggregates of clay loam soil compared to the control and the compound chemical fertilizer 15-15-15 based on 33% ammonium, phosphorus oxide, and potassium oxide.
Decesaro et al. [81] evaluated the ability to remediate soils contaminated with diesel and soybean oil by applying inactive biomass of Spirulina platensis and freeze-dried phycocyanin extracted from the microalgae as treatments. The results showed that phycocyanin was more effective in the biodegradation of soybean oil and biomass in the biodegradation of diesel, which indicates that these treatments can be effective in bioremediation processes.
Some microalgae are capable of growing in wastewater under unfavorable conditions such as salinity, alkalinity, temperatures, carbon dioxide concentrations, and light intensities, and are highly effective in their treatment by carbon dioxide fixation, use of inorganic nitrogen and phosphorus for its growth and water purification, producing oxygen and eliminating heavy metals and xenobiotic substances. These studies suggest a possible application in solid waste management. However, solid waste management is a complicated system because most government and private entities mainly use the conventional methods of landfilling, composting, and incineration, which have harmful effects on the environment [82].
However, currently, some limiting factors persist in their applications in bioremediation, such as its large-scale production and the selection of the appropriate strain, so it is necessary to generate more research for its use in wastewater remediation since that can benefit the environment and produce metabolites of interest that satisfy various productive areas [79,83].
As in bioremediation, the production of biofuels using microalgae is a technique that uses the specific characteristics of each species to carry out the process more efficiently. These applications that are presented in Table 6 can be grouped into three divisions: use of microalgae to produce biodiesel [84], bioethanol [14], and both biofuels [85].
Chauhan et al. [84] made the direct conversion of Chlorella sp. into biodiesel through transesterification with supercritical methanol, resulting in a biofuel that satisfies most of the biodiesel properties according to European standards.
Queiroz et al. [86] used Aphanothece microscopica Nägeli for fatty acids production through cultivation in bubble column bioreactors, applying cold culture intervals, which increased the production of fatty acids for their subsequent transformation into biofuel. The cultivation in photobioreactors of Scenedesmus obliquus with different cycles of light and darkness led by Maroneze et al. [93] demonstrated that the modulation of the photoperiods is efficient by improving the energy balance of the process to produce biodiesel.
On the other hand, Zaki et al. [21] cultivated Arthrospira platensis to obtain fatty acids as raw material to produce biodiesel. In the work of Pandey et al. [72] previously exposed, the lipid production for its subsequent production of biodiesel was evaluated, determining that, of the isolated microalgae, Scenedesmus sp. presented the best results in the production of raw material for biodiesel.
Satpati et al. [87] cultured Chlorella ellipsoidea and Chlorococcum infusionum under different salt concentrations to study the effect of salinity on growth and lipid accumulation. Maximum biomass and lipid accumulation were obtained at an exposure of 5 gL−1 NaCl in Chlorella ellipsoidea and 1.5 gL−1 NaCl in Chlorococcum infusionum, indicating that salinity can be considered the most important key regulator for growth and lipid accumulation in microalgae.
In the study of Satpati et al. [88] Leptolyngbya tenuis and Chlorella ellipsoidea were co-cultured, and the results of the co-culture showed a 2–3-fold increase in biomass, a two-fold increase in total lipids, and greater efficiency of bioremediation of cadmium compared to the pure culture. This suggests that the co-culture of these microalgae is a cost-effective and environmentally friendly production of biodiesel.
3.5.2 Production of Bioethanol
Khan et al. [90] made a pretreatment of Microcystis aureginosa with sulfuric acid, titanium oxide, lysozymes, invertase, and calcium oxide, and by fermentation of the resulting liquid, bioethanol was obtained, resulting in the calcium oxide is the most effective pretreatment for biofuel production. For their part, Chandra et al. [14] cultivated Scenedesmus acuminatus to generate biomass, which was treated with sulfuric acid to obtain ethanol after fermentation. Chng et al. [92] obtained biomass of Scenedesmus dimorphus through a bioreactor culture treated with various methods to obtain sugars that yield bioethanol upon fermentation.
The microalga Porphyridium cruentum was evaluated by Kim et al. [91] to gain glucose and its fermentation to obtain bioethanol, which achieved yields of 70.3% in freshwater cultures.
3.5.3 Production of Biodiesel and Bioethanol
The microalga Chlamydomonas sp. was cultivated by Kim et al. [85] in photobioreactors to synthesize fatty acids which were used in the production of biodiesel, while the free biomass of these acids was used in the production of bioethanol. Meanwhile, Sivaramakrishnan et al. [94] cultivated Scenedesmus sp. To obtain biomass, which was subjected to ultrasound treatment followed by acid hydrolysis, prior to sugar extraction and fermentation for bioethanol production. In addition, the ultrasound-treated biomass was used in a transesterification process, with which methyl esters were obtained to produce biodiesel.
Park et al. [95] tested culture media with different levels of salinity for the cultivation of the microalga Tretaselmis sp., showing that hypersaline culture media improve the production of biomass and fatty acids, which can be used for the production of biodiesel and bioethanol. Peralta-Ruiz et al. [89] carried out an energetic analysis of Chlorella sp. in the production of biodiesel and bioethanol using specialized software, which presented an 89% global energetic efficiency of the process contemplating the cultivation, harvesting, drying, transformation of oil and the transformation of the residual biomass of the microalgae, which indicates that the designed process is suitable to produce biofuels.
Since the 1960s there have been attempts to produce biofuels on a large scale, which have not reached commercial development due to the high production costs of biomass and the products obtained are expensive when compared to the price of oil and its derivatives. However, the rise in oil prices has directed attention to microalgae and has encouraged the development of biofuels [96]. However, due to the time required and the high cost of production, gene editing technology offers a cost-effective method for developing commercial mass production of biofuels for the future [97].
4 Problems, Limitations, and Potential Production of Microalgae
To address part of the problems mentioned in the study areas, the need to develop, through bioengineering, the production of biomass and metabolites derived from microalgae in a profitable manner has been indicated. Many of these technical problems lie in the need to increase the productivity of the microalgae, in addition to the selection of the strains, for which a strategy should be considered based on various criteria that are practical for the following problems: 1) speed of growth, 2) quantity and quality of metabolites, 3) response to environmental changes, 4) speed of absorption and affinity for nutrients, and 5) a simple culture [9,98].
Another fundamental need is the reduction of production costs, which can be achieved through 1) prevention of crop contamination, 2) optimization of key production equipment, such as ponds, photobioreactors, and clarifiers, among others, and 3) effective recovery of the products of interest produced [99].
However, despite so many limitations, the production of microalgae can become a profitable process, since in 2004 the market for microalgae-based products had a size of approximately 5,000 tons of dry matter per year and generated a sales volume of 1.25 trillion dollars a year, in 2017 it was estimated at 32.6 trillion dollars and it is projected that by 2026 it will reach 53.43 trillion dollars, so the correct attention to the mentioned problems can have a positive impact on the increase in the market [50,100–103].
According to what is documented in this work, the genus Chlorella stands out for its biotechnological applications in the five areas studied: in agriculture as a hydroponic crop to promote plant growth and as a biofertilizer that promotes the production of certain crops; in health, it presents a therapeutic potential against cancer, as well as the production of antimicrobial agents; in food, its biomass can be used as feed for farm animals, in the development of food supplements and as proteins to obtain emulsifiers; in bioremediation, it is used to eliminate nutrients in water with a high concentration of organic substances; Finally, the production of fatty acids is used for its transformation into biofuels.
The development of biotechnological applications for microalgae has gained importance, however, the studies have focused on a small number of them. The growing interest in more environmentally friendly technologies makes it necessary to promote research in a greater number of microalgae in search of novel biotechnological applications that can replace and/or improve some existing processes.
Acknowledgement: None.
Funding Statement: The authors received no specific funding for this study.
Author Contributions: The authors confirm contribution to the paper as follows: conceptualization, methods, information analysis, writing, proofreading, and edition: Reza-Solis Héctor Alejandro; conceptualization, methods, information analysis, writing, proofreading, and edition: Hernández-Rodríguez Ofelia Adriana; conceptualization, methods, writing, proofreading, and edition: Martínez-Rosales Andrés Francisco; validation, writing, and proofreading: Ojeda-Barrios Dámaris Leopoldina.
Availability of Data and Materials: All related information is available in this manuscript.
Ethics Approval: Not applicable.
Conflicts of Interest: The authors declare that they have no conflicts of interest to report regarding the present study.
References
1. Barsanti, L., Gualtieri, P. (2014). Algae: Anatomy, biochemistry, and biotechnology. Boca Ratón, USA: CRC Press. https://dx.doi.org/10.1201/b16544 [Google Scholar]
2. Dixit, S., Singh, D. (2015). Phycoremediation: Future perspective of green technology. In: Algae and environmental sustainability, pp. 9–21. New Delhi: Springer. https://dx.doi.org/10.1007/978-81-322-2641-3_2 [Google Scholar]
3. Subashchandrabose, S., Ramakrishnan, B., Megharaj, M., Venkateswarlu, K., Naidu, R. (2013). Mixotrophic cyanobacteria and microalgae as distinctive biological agents for organic pollutant degradation. Environment International, 51, 59–72. https://doi.org/10.1016/j.envint.2012.10.007 [Google Scholar] [PubMed] [CrossRef]
4. Gómez-Luna, L. (2007). Microalgas: Aspectos ecológicos y biotecnológicos. Revista Cubana de Química, 19(2), 3–20. [Google Scholar]
5. Muñoz, E. (2001). Biotecnología y Sociedad: Encuentros y desencuentros, vol. 1. Madrid, España: Ediciones AKAL, Cambridge University Press. [Google Scholar]
6. UN (1992). Convention on biological diversity, Río de Janeiro. https://www.cbd.int/doc/legal/cbd-en.pdf [Google Scholar]
7. DaSilva, E. J. (2004). The colours of biotechnology: Science, development and humankind. Electronic Journal of Biotechnology, 7(3), 2. [Google Scholar]
8. Thieman, W. J., Palladino, M. A. (2008). Introduction to biotechnology, 2nd edition. USA: Pearson. [Google Scholar]
9. García-Romeral, J., Pavía-Gómez, M., García-Sanz, T., Chirivella-Martorell, J., Serrano-Aroca, Á. (2017). Principles of biotechnology and bioengineering in microalgae cultures: Importance, technological problems, culture types and systems, growth, limiting factors, selection, isolation, grading and biochemical characterization. Nereis Revista Iberoamericana Interdisciplinar de Métodos, Modelización y Simulación, 9, 115–130. https://revistas.ucv.es/nereis/index.php/Nereis/article/view/100/80 [Google Scholar]
10. Godlewska, K., Michalak, I., Pacyga, P., Baśladyńska, S., Chojnacka, K. (2019). Potential applications of cyanobacteria: Spirulina platensis filtrates and homogenates in agriculture. World Journal of Microbiology and Biotechnology, 35(6), 1–18. https://doi.org/10.1007/s11274-019-2653-6 [Google Scholar] [PubMed] [CrossRef]
11. D’Adamo, S., Schiano di Visconte, G., Lowe, G., Szaub-Newton, J., Beacham, T. et al. (2019). Engineering the unicellular alga Phaeodactylum tricornutum for high-value plant triterpenoid production. Plant Biotechnology Journal, 17(1), 75–87. https://doi.org/10.1111/pbi.12948 [Google Scholar] [PubMed] [CrossRef]
12. Osorio-Fierros, A., Cronin, K., Ring, D., Méndez-Zavala, A., Morales-Oyervides, L. et al. (2017). Influence of granulation process parameters on food tablet properties formulated using natural powders (Opuntia ficus and Chlorella spp.). Powder Technology, 317, 281–286. https://doi.org/10.1016/j.powtec.2017.04.057 [Google Scholar] [CrossRef]
13. Altmann, B., Wigger, R., Ciulu, M., Mörlein, D. (2020). The effect of insect or microalga alternative protein feeds on broiler meat quality. Journal of the Science of Food and Agriculture, 100(11), 4292–4302. https://doi.org/10.1002/jsfa.10473 [Google Scholar] [PubMed] [CrossRef]
14. Chandra, N., Shukla, P., Mallick, N. (2020). Role of cultural variables in augmenting carbohydrate accumulation in the green microalga Scenedesmus acuminatus for bioethanol production. Biocatalysis and Agricultural Biotechnology, 26, 101632. https://doi.org/10.1016/j.bcab.2020.101632 [Google Scholar] [CrossRef]
15. Abalde, J., Cid, A., Fidalgo-Paredes, P., Torres, E., Herrero, C. (1995). Microalgas: Cultivo y aplicaciones. España: A Coruña Universidade, Servizo de Publicacións. https://dx.doi.org/10.17979/spudc.9788497497695 [Google Scholar]
16. Harder, V. R., Von Witsch, H. (1942). Bericht über Versuche zur Fettsynthese mittels autotropher Microorganismen. Forschungsdienst Sonderheft, 16, 270–275. [Google Scholar]
17. Milner, H. W. (1951). Possibilities in photosynthetic methods for production of oils and proteins. Journal of the American Oil Chemists’ Society, 28(8), 363–367. https://doi.org/10.1007/BF02589717 [Google Scholar] [CrossRef]
18. Borowitzka, M. A. (2013). Energy from microalgae: A short history. In: Borowitzka, M., Moheimani, N. (Eds.Algae for biofuels and energy, vol. 5, pp. 1–15. Dordrecht, Netherlands: Springer. https://dx.doi.org/10.1007/978-94-007-5479-9_1 [Google Scholar]
19. Richmond, A. (2008). Handbook of microalgal culture: Biotechnology and applied phycology. Cornwall, England: John Wiley & Sons, Blackwell Publishing. [Google Scholar]
20. Hernández-Reyes, B., Rodríguez-Palacio, M., Castilla-Hernández, P., Sánchez-Robles, J., Vela-Correa, G. et al. (2019). Uso potencial de cianobacterias como biofertilizante para el cultivo de maíz azul en la Ciudad de México. Revista Latinoamericana de Biotecnología Ambiental y Algal, 10(1), 13–27. [Google Scholar]
21. Zaki, M., Ashour, M., Heneash, A., Mabrouk, M., Alprol, A. et al. (2021). Potential applications of native cyanobacterium isolate (Arthrospira platensis NIOF17/003) for biodiesel production and utilization of its byproduct in marine rotifer (Brachionus plicatilis) production. Sustainability, 13(4), 1769. https://doi.org/10.3390/su13041769 [Google Scholar] [CrossRef]
22. Li, L., Li, X., Hong, Y., Jiang, M., Lu, S. (2020). Use of microalgae for the treatment of black and odorous water: Purification effects and optimization of treatment conditions. Algal Research, 47, 101851. https://doi.org/10.1016/j.algal.2020.101851 [Google Scholar] [CrossRef]
23. Barone, V., Puglisi, I., Fragalà, F., Piero, A., Giuffrida, F. et al. (2019). Novel bioprocess for the cultivation of microalgae in hydroponic growing system of tomato plants. Journal of Applied Phycology, 31(1), 465–470. https://doi.org/10.1007/s10811-018-1518-y [Google Scholar] [CrossRef]
24. Grzesik, M., Romanowska-Duda, Z., Kalaji, H. (2017). Effectiveness of cyanobacteria and green algae in enhancing the photosynthetic performance and growth of willow (Salix viminalis L.) plants under limited synthetic fertilizers application. Photosynthetica, 55(3), 510–521. https://doi.org/10.1007/s11099-017-0716-1 [Google Scholar] [CrossRef]
25. Chittapun, S., Limbipichai, S., Amnuaysin, N., Boonkerd, R., Charoensook, M. (2018). Effects of using cyanobacteria and fertilizer on growth and yield of rice, Pathum Thani I: A pot experiment. Journal of Applied Phycology, 30(1), 79–85. https://doi.org/10.1007/s10811-017-1138-y [Google Scholar] [CrossRef]
26. Bharti, A., Prasanna, R., Kumar, G., Kumar, A., Nain, L. (2019). Co-cultivation of cyanobacteria for raising nursery of chrysanthemum using a hydroponic system. Journal of Applied Phycology, 31(6), 3625–3635. https://doi.org/10.1007/s10811-019-01830-9 [Google Scholar] [CrossRef]
27. Zhang, J., Wang, X., Zhou, Q. (2017). Co-cultivation of Chlorella spp and tomato in a hydroponic system. Biomass and Bioenergy, 97, 132–138. https://doi.org/10.1016/j.biombioe.2016.12.024 [Google Scholar] [CrossRef]
28. Maurya, R., Chokshi, K., Ghosh, T., Trivedi, K., Pancha, I. et al. (2016). Lipid extracted microalgal biomass residue as a fertilizer substitute for Zea mays L. Frontiers in Plant Science, 6, 1266. https://doi.org/10.3389/fpls.2015.01266 [Google Scholar] [PubMed] [CrossRef]
29. Dineshkumar, R., Subramanian, J., Gopalsamy, J., Jayasingam, P., Arumugam, A. et al. (2019). The impact of using microalgae as biofertilizer in maize (Zea mays L.). Waste and Biomass Valorization, 10(5), 1101–1110. https://doi.org/10.1007/s12649-017-0123-7 [Google Scholar] [CrossRef]
30. de Paula Pereira, A. S. A., de Siqueira Castro, J., Ribeiro, V. J., Calijuri, M. L. (2021). Organo-mineral fertilizers pastilles from microalgae grown in wastewater: Ammonia volatilization and plant growth. Science of the Total Environment, 779, 146205. https://doi.org/10.1016/j.scitotenv.2021.146205 [Google Scholar] [PubMed] [CrossRef]
31. Martínez-Rosales, A., Trujillo-Tapia, M., Ramírez-Fuentes, E. (2020). Growth and metabolism of Fischerella TB22 in BG110 culture medium. Boletín Micológico, 35(1), 26–34. https://doi.org/10.22370/bolmicol.2020.35.1.2425 [Google Scholar] [CrossRef]
32. Mera, M., Recalde, E., Lema, K. (2019). Evaluación de soluciones de microalgas (Scenedesmus sp) como bioestimulante natural en el cultivo hidropónico de lechuga (Lactuca sativa). Axioma, (21), 53–60. https://doi.org/10.26621/XV21.2019.12.A06.PUCESI.2550.6684 [Google Scholar] [CrossRef]
33. Nayak, M., Kumar-Swain, D., Sen, R. (2019). Strategic valorization of de-oiled microalgal biomass waste as biofertilizer for sustainable and improved agriculture of rice (Oryza sativa L.) crop. Science of the Total Environment, 682, 475–484. https://doi.org/10.1016/j.scitotenv.2019.05.123 [Google Scholar] [PubMed] [CrossRef]
34. Collahuazo-Reinoso, Y., Araujo-Abad, S. (2019). Biofertilizer production from microalgae. Revista del Centro de Estudio y Desarrollo de la Amazonia, 9(2), 81–87. [Google Scholar]
35. Lin, P., Tsai, C., Chuang, W., Chao, Y., Pan, I. et al. (2017). Chlorella sorokiniana induces mitochondrial-mediated apoptosis in human non-small cell lung cancer cells and inhibits xenograft tumor growth in vivo. BMC Complementary and Alternative Medicine, 17(1), 1–8. https://doi.org/10.1186/s12906-017-1611-9 [Google Scholar] [PubMed] [CrossRef]
36. Vishwakarma, J., Sirisha, V. L. (2020). Unraveling the anti-biofilm potential of green algal sulfated polysaccharides against Salmonella enterica and Vibrio harveyi. Applied Microbiology and Biotechnology, 104(14), 6299–6314. https://doi.org/10.1007/s00253-020-10653-5 [Google Scholar] [PubMed] [CrossRef]
37. Hayashi, K., Lee, J., Atsumi, K., Kanazashi, M., Shibayama, T. et al. (2019). In vitro and in vivo anti-herpes simplex virus activity of monogalactosyl diacylglyceride from Coccomyxa sp. KJ (IPOD FERM BP-22254a green microalga. PLoS One, 14(7), e0219305. https://doi.org/10.1371/journal.pone.0219305 [Google Scholar] [PubMed] [CrossRef]
38. Corrêa da Silva, M., Pires-Ferreira, S., Dora, C., Hort, M., Giroldo, D. et al. (2020). Phenolic compounds and antioxidant capacity of Pediastrum boryanum (Chlorococcales) biomass. International Journal of Environmental Health Research, 32(1), 1–31. https://doi.org/10.1080/09603123.2020.1744113 [Google Scholar] [PubMed] [CrossRef]
39. Chen, Y. H., Chang, G. K., Kuo, S. M., Huang, S. Y., Hu, I. et al. (2016). Well-tolerated Spirulina extract inhibits influenza virus replication and reduces virus-induced mortality. Scientific Reports, 6(1), 1–11. https://doi.org/10.1038/srep24253 [Google Scholar] [PubMed] [CrossRef]
40. Reichert, M., Bergmann, S. M., Hwang, J., Buchholz, R., Lindenberger, C. (2017). Antiviral activity of exopolysaccharides from Arthrospira platensis against koi herpesvirus. Journal of Fish Diseases, 40(10), 1441–1450. https://doi.org/10.1111/jfd.12618 [Google Scholar] [PubMed] [CrossRef]
41. Mohanty, D., Samanta, L. (2018). Dietary supplementation of Spirulina ameliorates iron-induced oxidative stress in Indian knife fish Notopterus Notopterus. Environmental Toxicology and Pharmacology, 61, 71–78. https://doi.org/10.1016/j.etap.2018.05.007 [Google Scholar] [PubMed] [CrossRef]
42. da Silva-Ferreira, V., Ferreira-Conz, M., Lima, L., Frasés, S., de Souza, W. et al. (2017). Green production of microalgae-based silver chloride nanoparticles with antimicrobial activity against pathogenic bacteria. Enzyme and Microbial Technology, 97, 114–121. https://doi.org/10.1016/j.enzmictec.2016.10.018 [Google Scholar] [PubMed] [CrossRef]
43. Maadane, A., Merghoub, N., Mernissi, N. E., Ainane, T., Amzazi, S. et al. (2017). Antimicrobial activity of marine microalgae isolated from Moroccan coastlines. Journal of Microbiology, Biotechnology and Food Sciences, 6(6), 1257–1260. https://doi.org/10.15414/jmbfs.2017.6.6.1257-1260 [Google Scholar] [CrossRef]
44. Guevara, M., Zapata-Vívenes, E., León, M., Acosta, M. (2019). Antioxidant activity of oil vegetable enriched with the cultured microalgae Dunaliella salina (Chlorophyceae). AquaTechnica, 1(1), 40–46. https://doi.org/10.33936/at.v1i1.2150 [Google Scholar] [CrossRef]
45. Suh, S. S., Hong, J. M., Kim, E. J., Jung, S. W., Kim, S. M. et al. (2018). Anti-inflammation and anti-cancer activity of ethanol extract of antarctic freshwater microalga, Micractinium sp. International Journal of Medical Sciences, 15(9), 929–936. https://doi.org/10.7150/ijms.26410 [Google Scholar] [PubMed] [CrossRef]
46. Sanjeewa, K. K. A., Fernando, I. P. S., Samarakoon, K. W., Lakmal, H. H. C., Kim, E. A. et al. (2016). Anti-inflammatory and anti-cancer activities of sterol rich fraction of cultured marine microalga Nannochloropsis oculata. Algae, 31(3), 277–287. https://doi.org/10.4490/algae.2016.31.6.29 [Google Scholar] [CrossRef]
47. Verdugo-González, L., Acosta-Cárdenas, A., Zapata-Zapata, A. D., Cadena-Chamorro, E. M., Pulido-Muñoz, S. A. (2019). Antimicrobial potential of a hydrolyzed protein extract of the microalgae Nannochloropsis sp. Dyna, 86(211), 192. https://doi.org/10.15446/dyna.v86n211.78865 [Google Scholar] [CrossRef]
48. Singab, A. N., Ibrahim, N., Elsayed, A. E. K., El-Senousy, W., Aly, H. et al. (2018). Antiviral, cytotoxic, antioxidant and anti-cholinesterase activities of polysaccharides isolated from microalgae Spirulina platensis, Scenedesmus obliquus and Dunaliella salina. Archives of Pharmaceutical Sciences Ain Shams University, 2(2), 121–137. https://doi.org/10.21608/aps.2018.18740 [Google Scholar] [CrossRef]
49. Patil, J., Matte, A., Mallard, C., Sandberg, M. (2016). Spirulina diet to lactating mothers protects the antioxidant system and reduces inflammation in post-natal brain after systemic inflammation. Nutritional Neuroscience, 21(1), 59–69. https://doi.org/10.1080/1028415X.2016.1221496 [Google Scholar] [PubMed] [CrossRef]
50. Sánchez-Rodríguez, E., Mesa-García, M. D. (2018). Compuestos bioactivos del aceite de oliva virgen. Nutrición Clínica, 12, 80–94. https://doi.org/10.7400/NCM.2018.12.2.5064 [Google Scholar] [CrossRef]
51. Chatterjee, D., Bhattacharjee, P., Satpati, G. G., Pal, R. (2014). Spray dried extract of Phormidium valderianum as source of natural antioxidant. International Journal of Food Science, 2014, 1–8. https://doi.org/10.1155/2014/897497 [Google Scholar] [PubMed] [CrossRef]
52. Ramírez-Mérida, L. G., de Menezes, C. R., Queiroz-Zepka, L., Jacob-Lopes, E. (2015). Microalgas: Potencial para la producción de compuestos bioactivos nanoencapsulados. Ciência e Natura, 37(5), 7–17. https://doi.org/10.5902/2179-460X19690 [Google Scholar] [CrossRef]
53. Sen, S., Satpati, G. G., Basu, P. (2022). Algal bioactive compounds against sexually transmitted diseases. Biosciences Biotechnology Research Asia, 19(3), 553–559. https://doi.org/10.13005/bbra/3009 [Google Scholar] [CrossRef]
54. Satpati, G. G. (2020). Algal sulfated polysaccharides: Potent immunomodulators against COVID-19 in pandemic 2020. Biosciences Biotechnology Research Asia, 17(3), 601–605. https://doi.org/10.13005/bbra/2863 [Google Scholar] [CrossRef]
55. Li, Y., Lammi, C., Boschin, G., Arnoldi, A., Aiello, G. (2019). Recent advances in microalgae peptides: Cardiovascular health benefits and analysis. Journal of Agricultural and Food Chemistry, 67(43), 11825–11838. https://doi.org/10.1021/acs.jafc.9b03566 [Google Scholar] [PubMed] [CrossRef]
56. Graça, C., Fradinho, P., Sousa, I., Raymundo, A. (2018). Impact of Chlorella vulgaris on the rheology of wheat flour dough and bread texture. LWT, 89, 466–474. https://doi.org/10.1016/j.lwt.2017.11.024 [Google Scholar] [CrossRef]
57. Lucas, B., de Morais, M., Santos, T., Costa, J. (2018). Spirulina for snack enrichment: Nutritional, physical and sensory evaluations. LWT, 90, 270–276. https://doi.org/10.1016/j.lwt.2017.12.032 [Google Scholar] [CrossRef]
58. Hosoglu, M. (2018). Aroma characterization of five microalgae species using solid-phase microextraction and gas chromatography-mass spectrometry/olfactometry. Food Chemistry, 240, 1210–1218. https://doi.org/10.1016/j.foodchem.2017.08.052 [Google Scholar] [PubMed] [CrossRef]
59. Böcker, L., Bertsch, P., Wenner, D., Teixeira, S., Bergfreund, J. et al. (2021). Effect of Arthrospira platensis microalgae protein purification on emulsification mechanism and efficiency. Journal of Colloid and Interface Science, 584, 344–353. https://doi.org/10.1016/j.jcis.2020.09.067 [Google Scholar] [PubMed] [CrossRef]
60. Uribe-Wandurraga, Z. N., Igual, M., García-Segovia, P., Martínez-Monzó, J. (2020). In vitro bioaccessibility of minerals from microalgae-enriched cookies. Food & Function, 11(3), 2186–2194. https://doi.org/10.1039/C9FO02603G [Google Scholar] [PubMed] [CrossRef]
61. Uribe-Wandurraga, Z. N., Zhang, L., Noort, M. W., Schutyser, M. A., García-Segovia, P. et al. (2020). Printability and physicochemical properties of microalgae-enriched 3D-printed snacks. Food and Bioprocess Technology, 13(11), 2029–2042. https://doi.org/10.1007/s11947-020-02544-4 [Google Scholar] [CrossRef]
62. Barraza-Guardado, R., Pérez-Villalba, A., González-Félix, M., Ortega-Urbina, J., Muñoz-Hernández, R. et al. (2020). The use of microalgae as partial constituents of growout feeds for tilapia (Oreochromis niloticus). Biotecnia, 22(1), 135–141. https://doi.org/10.18633/biotecnia.v22i1.1161 [Google Scholar] [CrossRef]
63. Grossmann, L., Ebert, S., Hinrichs, J., Weiss, J. (2019). Formation and stability of emulsions prepared with a water-soluble extract from the microalga Chlorella protothecoides. Journal of Agricultural and Food Chemistry, 67(23), 6551–6558. https://doi.org/10.1021/acs.jafc.8b05337 [Google Scholar] [PubMed] [CrossRef]
64. Guldhe, A., Ansari, F., Singh, P., Bux, F. (2017). Heterotrophic cultivation of microalgae using aquaculture wastewater: A biorefinery concept for biomass production and nutrient remediation. Ecological Engineering, 99, 47–53. https://doi.org/10.1016/j.ecoleng.2016.11.013 [Google Scholar] [CrossRef]
65. Ebert, S., Grossmann, L., Hinrichs, J., Weiss, J. (2019). Emulsifying properties of water-soluble proteins extracted from the microalgae Chlorella sorokiniana and Phaeodactylum tricornutum. Food & Function, 10(2), 754–764. https://doi.org/10.1039/C8FO02197J [Google Scholar] [PubMed] [CrossRef]
66. Kafyra, M., Papadaki, S., Chronis, M., Krokida, M. (2018). Microalgae based innovative animal fat and proteins replacers for application in functional baked products. Open Agriculture, 3(1), 427–436. https://doi.org/10.1515/opag-2018-0047 [Google Scholar] [CrossRef]
67. Beigbeder, J., Boboescu, I., Lavoie, J. (2019). Thin stillage treatment and co-production of bio-commodities through finely tuned Chlorella vulgaris cultivation. Journal of Cleaner Production, 216, 257–267. https://doi.org/10.1016/j.jclepro.2019.01.111 [Google Scholar] [CrossRef]
68. Hossain, A., Brennan, M., Mason, S., Guo, X., Zeng, X. et al. (2017). The effect of astaxanthin-rich microalgae Haematococcus pluvialis and wholemeal flours incorporation in improving the physical and functional properties of cookies. Foods, 6(8), 57. https://doi.org/10.3390/foods6080057 [Google Scholar] [PubMed] [CrossRef]
69. Nunes da Silva, V. D., do Moraes Gatti, V. C., Martins Carvalho, F. I., Teixeira de Oliveira, J., Andrade Silva, P. et al. (2021). Sensory characteristics of umbu handmade liquor with microalgae. Natural Resources, 11(2), 7–12. https://doi.org/10.6008/CBPC2237-9290.2021.002.0002 [Google Scholar] [CrossRef]
70. Spolaore, P., Joannis-Cassan, C., Duran, E., Isambert, A. (2006). Commercial applications of microalgae. Journal of Bioscience and Bioengineering, 101(2), 87–96. https://doi.org/10.1263/jbb.101.87 [Google Scholar] [PubMed] [CrossRef]
71. Olmedo-Galarza, V. (2019). Carbohidratos y proteínas en microalgas: Potenciales alimentos funcionales. Brazilian Journal of Food Technology, 22. https://doi.org/10.1590/1981-6723.04319 [Google Scholar] [CrossRef]
72. Pandey, A., Srivastava, S., Kumar, S. (2019). Isolation, screening and comprehensive characterization of candidate microalgae for biofuel feedstock production and dairy effluent treatment: A sustainable approach. Bioresource Technology, 293, 121998. https://doi.org/10.1016/j.biortech.2019.121998 [Google Scholar] [PubMed] [CrossRef]
73. Park, C., Li, X., Zhao, Y., Jia, R., Hur, J. (2017). Rapid development of cyanobacterial crust in the field for combating desertification. PLoS One, 12(6), e0179903. https://doi.org/10.1371/journal.pone.0179903 [Google Scholar] [PubMed] [CrossRef]
74. Navarrete-Álava, J., Noles-Aguilar, P., Delgado-Villafuerte, C., Hernández de Guerrero, N., Guerrero-Ríos, R. (2022). Bioremediation of shrimp farming effluents by means of autochthonous microbial consortia and native microalgae in Manabí, Ecuador. AquaTechnica, 4(1), 53–65. https://doi.org/10.33936/at.v4i1.4635 [Google Scholar] [CrossRef]
75. Yilmaz, E., Sönmez, M. (2017). The role of organic/bio-fertilizer amendment on aggregate stability and organic carbon content in different aggregate scales. Soil and Tillage Research, 168, 118–124. https://doi.org/10.1016/j.still.2017.01.003 [Google Scholar] [CrossRef]
76. Lv, J., Liu, Y., Feng, J., Liu, Q., Nan, F. et al. (2018). Nutrients removal from undiluted cattle farm wastewater by the two-stage process of microalgae-based wastewater treatment. Bioresource Technology, 264, 311–318. https://doi.org/10.1016/j.biortech.2018.05.085 [Google Scholar] [PubMed] [CrossRef]
77. Ortiz-Villota, M., Romero-Morales, M., Meza-Rodríguez, L. (2018). The bioremediation with microalgae (Spirulina maxima, Spirulina platensis y Chlorella vulgaris) as an alternative to treat the eutrophization of the Ubaque lagoon, Colombia. Revista de Investigación Desarrollo e Innovación, 9(1), 163–176. https://doi.org/10.19053/20278306.v9.n1.2018.8153 [Google Scholar] [CrossRef]
78. Leong, W., Lim, J., Lam, M., Uemura, Y., Ho, C. et al. (2018). Co-cultivation of activated sludge and microalgae for the simultaneous enhancements of nitrogen-rich wastewater bioremediation and lipid production. Journal of the Taiwan Institute of Chemical Engineers, 87, 216–224. https://doi.org/10.1016/j.jtice.2018.03.038 [Google Scholar] [CrossRef]
79. Cheirsilp, B., Thawechai, T., Prasertsan, P. (2017). Immobilized oleaginous microalgae for production of lipid and phytoremediation of secondary effluent from palm oil mill in fluidized bed photobioreactor. Bioresource Technology, 241, 787–794. https://doi.org/10.1016/j.biortech.2017.06.016 [Google Scholar] [PubMed] [CrossRef]
80. Nogueira, S., Junior, J., Maia, H., Saboya, J., Farias, W. (2018). Use of Spirulina platensis in treatment of fish farming wastewater. Revista Ciência Agronômica, 49, 599–606. https://doi.org/10.5935/1806-6690.20180068 [Google Scholar] [CrossRef]
81. Decesaro, A., Rampel, A., Machado, T. S., Thomé, A., Reddy, K. et al. (2017). Bioremediation of soil contaminated with diesel and biodiesel fuel using biostimulation with microalgae biomass. Journal of Environmental Engineering, 143(4), 04016091. https://doi.org/10.1061/(ASCE)EE.1943-7870.0001165 [Google Scholar] [CrossRef]
82. Satpati, G. G. (2021). Solid waste management by algae: Current applications and future perspectives. Pollution Research, 40(1), 260–265. [Google Scholar]
83. An, J. Y., Sim, S. J., Lee, J. S., Kim, B. W. (2003). Hydrocarbon production from secondarily treated piggery wastewater by the green alga Botryococcus braunii. Journal of Applied Phycology, 15(2), 185–191. https://doi.org/10.1023/A:1023855710410 [Google Scholar] [CrossRef]
84. Chauhan, D., Goswami, G., Dineshbabu, G., Palabhanvi, B., Das, D. (2020). Evaluation and optimization of feedstock quality for direct conversion of microalga Chlorella sp. FC2 IITG into biodiesel via supercritical methanol transesterification. Biomass Conversion and Biorefinery, 10(2), 339–349. https://doi.org/10.1007/s13399-019-00432-2 [Google Scholar] [CrossRef]
85. Kim, E. J., Kim, S., Choi, H. G., Han, S. (2020). Co-production of biodiesel and bioethanol using psychrophilic microalga Chlamydomonas sp. KNM0029C isolated from Arctic sea ice. Biotechnology for Biofuels, 13(1), 1–13. https://doi.org/10.1186/s13068-020-1660-z [Google Scholar] [PubMed] [CrossRef]
86. Queiroz, M., Maroneze, M., Manetti, A., Vieira, J., Zepka, L. et al. (2018). Enhanced single-cell oil production by cold shock in cyanobacterial cultures. Ciência Rural, 48. https://doi.org/10.1590/0103-8478cr20180366 [Google Scholar] [CrossRef]
87. Satpati, G. G., Gorain, P. C., Paul, I., Pal, R. (2016). An integrated salinity-driven workflow for rapid lipid enhancement in green microalgae for biodiesel application. RSC Advances, 6, 112340–112355. https://doi.org/10.1039/C6RA23933A [Google Scholar] [CrossRef]
88. Satpati, G. G., Pal, R. (2021). Co-cultivation of Leptolyngbya tenuis (Cyanobacteria) and Chlorella ellipsoidea (Green alga) for biodiesel production, carbon sequestration, and cadmium accumulation. Current Microbiology, 78, 1466–1481. https://doi.org/10.1007/s00284-021-02426-8 [Google Scholar] [PubMed] [CrossRef]
89. Peralta-Ruiz, Y., Obregon, L. G., Gonzalez-Delgado, A. D. (2018). Design of biodiesel and bioethanol production process from microalgae biomass using exergy analysis methodology. Chemical Engineering Transactions, 70, 1045–1050. https://doi.org/10.3303/CET1870175 [Google Scholar] [CrossRef]
90. Khan, M., Lee, M., Shin, J., Kim, J. (2017). Pretreatment optimization of the biomass of Microcystis aeruginosa for efficient bioethanol production. AMB Express, 7(1), 1–9. https://doi.org/10.1186/s13568-016-0320-y [Google Scholar] [PubMed] [CrossRef]
91. Kim, H. M., Oh, C. H., Bae, H. J. (2017). Comparison of red microalgae (Porphyridium cruentum) culture conditions for bioethanol production. Bioresource Technology, 233, 44–50. https://doi.org/10.1016/j.biortech.2017.02.040 [Google Scholar] [PubMed] [CrossRef]
92. Chng, L. M., Lee, K. T., Chan, D. C. J. (2017). Evaluation on microalgae biomass for bioethanol production. IOP Conference Series: Materials Science and Engineering, vol. 206, no. 1, 012018. Malaysia. https://doi.org/10.1088/1757-899X/206/1/012018 [Google Scholar] [CrossRef]
93. Maroneze, M., Deprá, M., Zepka, L., Jacob-Lopes, E. (2019). Artificial lighting strategies in photobioreactors for bioenergy production by Scenedesmus obliquus CPCC05. SN Applied Sciences, 1(12), 1–12. https://doi.org/10.1007/s42452-019-1761-0 [Google Scholar] [CrossRef]
94. Sivaramakrishnan, R., Incharoensakdi, A. (2018). Utilization of microalgae feedstock for concomitant production of bioethanol and biodiesel. Fuel, 217, 458–466. https://doi.org/10.1016/j.fuel.2017.12.119 [Google Scholar] [CrossRef]
95. Park, H., Jung, D., Lee, J., Kim, P., Cho, Y. et al. (2018). Improvement of biomass and fatty acid productivity in ocean cultivation of Tetraselmis sp. using hypersaline medium. Journal of Applied Phycology, 30(5), 2725–2735. https://doi.org/10.1007/s10811-018-1388-3 [Google Scholar] [CrossRef]
96. Acién, F. G., Fernández-Sevilla, J. M., Magán, J. J., González-Céspedes, A., Molina, E. (2012). Evaluación global de la producción de biocombustibles con microalgas: Establecimiento de capacidades, limitaciones y factores determinantes de la viabilidad del proceso. Revista Latinoamericana de Biotecnología Ambiental y Algal, 3(1), 41–58. [Google Scholar]
97. Sen, S., Satpati, G. G. (2021). CRISPR mediated lipid enhancement in microalgae. Research Journal of Biotechnology, 16(1), 213–219. [Google Scholar]
98. Fernández-Linares, L. C., Montiel-Montoya, J., Millán-Oropeza, A., Badillo-Corona, J. A. (2012). Production of biofuels obtained from microalgae. Ra Ximhai, 8(3), 101–115. [Google Scholar]
99. Barclay, W., Apt, K. (2013). Strategies for bioprospecting microalgae for potential commercial applications. In: Handbook of microalgal culture: Applied phycology and biotechnology, pp. 69–79. West Sussex, England: Blackwell Publishing. https://dx.doi.org/10.1002/9781118567166.ch4 [Google Scholar]
100. Pulz, O., Gross, W. (2004). Valuable products from biotechnology of microalgae. Applied Microbiology and Biotechnology, 65(6), 635–648. https://doi.org/10.1007/s00253-004-1647-x [Google Scholar] [PubMed] [CrossRef]
101. Levasseur, W., Perré, P., Pozzobon, V. (2020). A review of high value-added molecules production by microalgae in light of the classification. Biotechnology Advances, 41, 107545. https://doi.org/10.1016/j.biotechadv.2020.107545 [Google Scholar] [PubMed] [CrossRef]
102. Rahman, K. M. (2020). Food and high value products from microalgae: Market opportunities and challenges. In: Alam, M. A., Xu, J. L., Wang, Z. (Eds.Microalgae biotechnology for food, health and high value products, pp. 3–27. Singapore: Springer. https://dx.doi.org/10.1007/978-981-15-0169-2_1 [Google Scholar]
103. Sánchez, J., Loaña, J., Agualongo, M., Espinoza, K. (2020). Cultivation techniques and methods of extraction of fatty acids based on microalgae for the benefit of humanity. Agroindustrial Science, 10(3), 319–328. https://doi.org/10.17268/agroind.sci.2020.03.15 [Google Scholar] [CrossRef]
Cite This Article
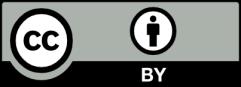