Open Access
ARTICLE
Improvement of Selected Morphological, Physiological, and Biochemical Parameters of Banana (Musa acuminata L.) Using Potassium Silicate under Drought Stress Condition Grown in vitro
1
Department of Horticulture, Faculty of Agriculture, Al-Azhar University, Cairo, 11651, Egypt
2
Department of Biochemistry, Faculty of Agriculture, Al-Azhar University, Cairo, 11651, Egypt
3
Department of Biochemistry and Molecular Biology, College of Marine Life Sciences, Ocean University of China, Qingdao, 266003, China
4
Department of Agricultural Botany, Faculty of Agriculture, Al-Azhar University, Nasr City, Cairo, 11751, Egypt
5
Department of Horticulture, Faculty of Agriculture, Ain Shams University, Cairo, 11566, Egypt
6
Department of Biology, College of Science, Princess Nourah bint Abdulrahman University, Riyadh, 11671, Saudi Arabia
7
Department of Biochemistry, Faculty of Agriculture, Ain Shams University, Cairo, 11566, Egypt
8
Biology Department, College of Science, Imam Mohammad ibn Saud Islamic University (IMSIU), Riyadh, 11623, Saudi Arabia
9
Botany Department, Faculty of Science, Suez Canal University, Ismailia, 41522, Egypt
10 Botany Department, Faculty of Science, Port Said University, Port Said, 42511, Egypt
* Corresponding Author: Khadiga Alharbi. Email:
(This article belongs to the Special Issue: Selenium, Silicon and their Nanoparticles-mediated Environmental Stress Tolerance in Crop Plants)
Phyton-International Journal of Experimental Botany 2023, 92(4), 1019-1036. https://doi.org/10.32604/phyton.2023.026769
Received 25 September 2022; Accepted 14 November 2022; Issue published 06 January 2023
Abstract
Drought stress has become more common in recent years as a result of climate change impacts on the production of banana crops and other fruit trees. The growth and productivity of Musa spp are severely impacted by the gradual degradation of water resources and the erratic distribution pattern of annual precipitation amount. The aim of the work includes increased drought tolerance in light of water scarcity in the world as a result of the bananas’ being gluttonous for water needs. This investigation was carried out from 2019 to 2020 to study the effect of potassium silicate on morphological growth and biochemical parameters of Musa acuminata L under drought stress by PEG. As a result, drought stress reduced the morphological characteristics such as shoots number, shoot length, roots number, and survival percentage and biochemical characteristics such as chlorophyll a, b, carotenoids, stomatal status, and RWC. While proline content increased in the leaf of M. acuminata L. Media complemented with K2SiO3 (2 to 6 mM) either individually or in combination with PEG led to an improvement in all morphological and biochemical characteristics. The activities of CAT, POD, and PPO enzymes increased signifi- cantly compared to control. Furthermore, the lowest PPO, CAT, and POD activity were achieved. Additionally, K2SiO3 treatments under drought stress successfully enhanced the leaf stomatal behavior. Our results suggest that K2SiO3 can help to maintain plant integrity in the tested cultivar under drought stress.Keywords
Banana is the 15th most important fruit and the 4th most valuable food crop in several developing countries after wheat, rice, and maize [1]. In its primary production areas, it contributes as a source of food, employment, and revenue [1]. Tissue culturing has recently gained popularity as a viable alternative. A variety of variable environmental factors reduce commercial banana production [2]. Drought is one of the most damaging abiotic stresses in agriculture, affecting plant growth and metabolic activities such as water relations, photosynthetic assimilation, and nutrient uptake [2]. Despite its relevance, all biotic restrictions pose a threat to its production. The banana plant is susceptible to water shortages. When there is a significant water shortage, the banana pseudo-stem collapses, and all the leaves fall prematurely [3]. Tissue culture has recently grown in popularity as one of the most current breeding procedures for various crops, vegetables, and fruits [4]. Improper soil salinity causes a loss of both vegetative potential and productivity, among other environmental pressures. The low yield and output of bananas in Egypt are due to the scarcity of water in this area and the world under various biotic and abiotic factors [4].
Plants that face stress utilize several defense mechanisms to minimize the stress [5]. The plant frequently synthesizes and accumulates osmoprotectants or suitable solutes like glycine betaine, trehalose, polyamines, or proline [5]. Abiotic stress tolerance can be acquired by pre-treating with such a protective chemical [5]. Drought resistance, prevention, and tolerance are the three primary techniques plants utilize to cope with this physiologically unsustainable environment [6]. Drought stress induction is the most widely used method, which employs high molecular weight osmotic agents like polyethylene glycol (PEG) [7,8]. These compounds showed no adverse or harmful impacts on the plant [9]; nevertheless, they restrict plant development by lowering the water potential of the culture medium, like soil drying, preventing cultured explants from absorbing water [10]. Drought, too, necessitates a thorough understanding for plants to thrive. It results in a lack of water availability for growing plants. Water stress, which is often accompanied by increased oxidative damage, negatively impacts the growth and production of the banana plant as a commercial crop in the tropical and subtropical parts of the world [11].
Silicon (Si) has been shown to help plants withstand biotic and abiotic stressors such as salinity, aluminum toxicity, drought, radiation, nutritional imbalance, high temperature, heavy metal toxicity, lodging, freezing, and wounding [12]. Augmenting water transport and uptake, amendable transpiration water loss and stomata behavior, osmoregulatory substances and accumulating solutes, and triggering plant defense-associated signaling events are key mechanisms influencing Si’s ability to modulate the effects of drought stress, resulting in whole-plant water balance and helping plants cope with environmental challenges by reducing oxidative damage [12,13]. Si priming may be indirectly beneficial because it allows the plant to develop and produce more by reducing the likelihood of biotic and abiotic challenges such as insect pest attack, disease, drought, and nutrient losses [14]. In several studies, Si has been identified as a nutrient that plays an essential function in environmental stress [15]. Si improves drought tolerance in plants by reducing oxidative damage to functional molecules and amplifying antioxidant defense abilities [16]. Si aids in the development of cell strength and the power of plants to respond to environmental challenges [17].
Accumulation of silica in the polymerized silicon dioxide (SiO2) form can cause anatomical alterations in cell walls [18]. Plants that are more drought tolerant have a better chance of survival, when plants are challenged by drought, they have a limited capacity to fix carbon. This condition restricts CO2 absorption to reactive oxygen species (ROS) due to stomata closure, which prolong excess electron flow to O2 and induces overproduction of ROS [17]. During drought stress, the glycolate oxidase pathway is also activated, resulting in the formation of hydrogen peroxide (H2O2) [18]. As a result, dryness causes oxidative stress in plant cells. ROS can cause cell death by damaging chlorophyll pigments, proteins, Deoxyribonucleic Acid, and lipid membranes [19].
Drought stress resistance is linked to antioxidant enzyme activity [20]. Si added to the culture medium enhanced the morphogenetic capacity of plant cells, tissues, and organs, where Si increases plant tolerance to diverse stressors by changing the activity of antioxidant enzymes [21]. Among the nutritional components, Si is a component that has received little attention in plant micropropagation. However, from a physiological standpoint, Si has been found to boost the production of numerous crops by promoting plant growth and development [22]. Seedlings cultivated in vitro can benefit from a Si-supplemented culture medium, which increases the quantity of hemicellulose and lignin and hence the rigidity of the cell wall [23].
Previous research has found that adding Si to tissue culture media reduces oxidative damage and oxidative phenolic browning and increases antioxidant enzymes, secondary metabolite synthesis, and tolerance to abiotic and biotic stimuli [21]. Accordingly, the main objective of this research was to investigate Si’s potential to maintain water balance during drought stress conditions and suggest future research that will be required to utilize Si in agriculture.
2.1 Plant Material and Experimental Design
This study was executed from 2019 to 2020 at the tissue culture laboratory of Horticulture Department, Faculty of Agriculture, and Al-Azhar University, where the suckers of M. acuminata L grandinin cultivar were taken from banana trees growing on a farm in the AL-Salhiyah region, Sharkia Governorate, Egypt.
The suckers were carefully washed in tap water, leaf sheaths and roots were removed, and the basal portion of the corm was cut to 12 mm × 12 mm × 15 mm size. Then, the explants were kept for 30 min under running tap water, then soaked for 30 min in the clean sole, and continuously shacked. Then, the detergent particles were removed from the explants by washing with distilled water. Then, they were treated for 30 min with fungicide (SAAF + INDOFIL), followed by washing with distilled water. Finally, they were transferred to a laminar airflow chamber for additional sterilization as shown in Fig. 1.
Figure 1: Source and preparation explant of M. acuminata L including (A) Entire plant and saucers, (B) Shoot tip explant, and (C) Meristem proliferation
2.3 Preparation of Culture Media
The explants were treated inside the laminar flow chamber with 70% ethanol for 2 min, then washed with sterile water. Subsequently, the explants were treated for 5 min with 0.1% HgCl2, and rinised three times with sterile water 5 min each. The explants were cut to a 8 mm × 8 mm × 10 mm size, inoculated on of M. acuminata L (MS) media supplemented in sterile conditions with agar 7 g/L, sucrose 30 g/L, NAA at 0.01 mg/L, and BA at 1.0 mg/L; and incubated in dark for 21 days at 27°C ± 2°C. The media utilized for growth was replaced for 3 times after 21 days, as the growth may inhibit by the phenolic released into the medium. Current of M acuminata L grandinin cultivar shoots were incubated at 27°C ± 2°C with a 16 h photoperiod for four weeks in the starting stage. After 30 days, uniformly developed explants were excised and re-cultured on the multiplication stage where of M. acuminata L grandinin cultivar micro-shoots were cultured on MS media supplemented with sucrose 30 g/L, agar 7 g/L, 1.0 mg/L of both BA and KIN. After another 30 days, explants which are uniformly developed were chosen and transferred to the MS media consisting of 7 g/L agar, 1.0 mg/L BA, 30 g/L sucrose, 0.01 mg/L NAA, various concentrations of PEG 6000 (0.0%, 2%, 4%, and 6%) and potassium silicate, K2SiO3, at 0.0, 2.0, 4.0, and 6.0 mM with above concentration of PEG. The incubation conditions were kept the same as previous described in the multiplication stage, where (0.0) as a control is free MS media (without both PEG and K2SiO3).
2.4 Data Collection and Morphological and Biochemical Characteristics
2.4.1 Determination of Morphological Characteristics
Growth of axillary shoots under salt stress evaluated after 30 days by measuring the increase in shoots number, shoot length (cm), roots number, and survival percentage was assessed based on the following equation: We used 9 plants for each treatment to estimate the survival percentage and the remainder of the morphological characteristics.
2.4.2 Relative Water Content in Leaf
Relative water contents (RWC, %) was implemented as previously reported [24], and was calculated using the following equation: To calculate the RWC, we used 9 leaves for each treatment. Leaf discs (about 1.0 cm in diameter) to minimize the area of chopped leaf surface/sample.
where: DW is the dry weight; FW is the fresh weight; and TW is the turgid weight.
2.4.3 Determination of Photosynthetic Pigments
At the end of the experimental phase (30 days), total chlorophyll, chlorophyll a & b, and carotenoid contents of the leaves have been measured according to [25]. To do an estimation of the photosynthetic pigments, we used 9 leaves for each treatment. We took 0.5 g of the fresh weight of leaves.
2.4.4 Determination Proline Content
The rapid colorimetric method was followed [26] to estimate proline contents. Briefly, ninhydrin (1.25 g) was treated with glacial acetic acid (30 mL), followed by 6 M phosphoric acid (20 mL). The resulting mixture was heated until a clear solution was obtained and subsequently cooled and stored at 4°C (stable for 24 h). Next, the explant material (0.5 g) was homogenized in 3% aqueous sulfosalicylic acid (10 mL), and subsequently filtered. The filtrate (2 mL) was treated with freshly prepared acid ninhydrin (2 mL), followed by glacial acetic acid (2 mL). After the resultant mixture was heated at 100°C for 1 h, the reaction mixture was poured into ice. The obtained mixture was subsequently extracted with toluene (4 mL) and vigorously mixed for 15–20 s. The toluene phase was separated and the absorption of the chromophore was assessed by spectrophotometer at a wavelength of 520 nm utilizing toluene as a reference blank. The concentration of proline was finally evaluated from the standard curve and the fresh weight was defined.
2.4.5 Determination of Antioxidant Enzymes
The tissue was prepared for examining the enzymatic antioxidant. Briefly, 0.2 g of petals samples and fresh leaves was poured into liquid N2 and subsequently homogenized in an ice bath using the following homogenizing solution (4 mL) which consisted of 1% (w/v) polyvinylpyrrolidone and 50 mM potassium phosphate buffer (pH 7.8). Subsequently, the homogenate was centrifuged at 4°C for 10 min at 14,000 rpm, and the obtained supernatant was utilized for enzymatic assays. Peroxidase (POD) activity assay was measured according to the method of [27], catalase (CAT) assay was precise according to [28], polyphenol oxidase (PPO) assay was done according to Duckworth et al. [29].
This experiment was performed to investigate the effect of MS media complemented with PEG at 0.0%, 2%, 4%, and 6% either individually or in combination with potassium silicate, K2SiO3, at (0.0, 2.0, 4.0, and 6.0 mM). Stomata characteristics were the number of stomata, inside length and width of stomata in µm, and outside length and width of stomata in µm of M. acuminata L grandinin cultivar. The samples of low surface leaves of M. acuminata L were coated by a gold sputter coater (SPI-Module). The number of stomata/mm2 (average number of 3 fields) and stomata dimensions (10 stomata randomly selected per field) of M. acuminata L grandinin cultivar were measured for each imprint. Lastly, the samples were analyzed by scanning electron microscopy (JEOL JSM-5500 LV) utilizing high vacuum mode at the Regional Center of Mycology and Biotechnology, Cairo, Egypt [30].
The design of the present study followed a complete randomized block design. The analysis of variance was accomplished employing 4 × 4 two-way ANOVA Co-stat software [31] and means were compared using the Duncan test, a significant (p ≤ 0.05). Despite it was a two-way ANOVA, mean comparisons were made by pairs among all treatments.
3.1 Effect of PEG and K2SiO3 on Growth Morphological Parameters
Exposing M. acuminata L grandinin cultivar cv. to drought stress with PEG significantly (p ≤ 0.05) decreased the growth parameters compared with unstressed M. acuminata L grandinin cv. (Fig. 2). In general, there are no significant differences between the stress treatments with the control. Increase PEG from 2% up to 6% (w/v) led to a decrease in shoots number (Fig. 1A), shoot length (Fig. 1B), roots number (Fig. 1C), and survival percentage (Fig. 1D) compared with control. At the same time, the maximum decrease was gained when the micro-plantlet of M. acuminata L grandinin cv. was cultured with PEG 6% (w/v) compared with unstressed and other treatments. In contrast, adding K2SiO3 individually to MS media from 2.0 up to 6.0 mM significantly enhanced all studied morphological growth parameters of M. acuminata L grandinin cv. compared to those without K2SiO3. In contrast, K2SiO3 at low level combined with PEG didn’t affect growth of the tested cultivar.
Figure 2: Effect of M. acuminata L grandinin cv. media supplemented with PEG either individually or in combination with K2SiO3 on morphological parameters, including (A) shoots number, (B) shoot length, (C) roots number, and (D) survival percentage. Numerical data are reported as mean ± SD. L.S.D at 5% of shoot length = 2.04, roots number = 0.98
3.2 Effect of PEG and K2SiO3 on Relative Water Content
The relative water content of M. acuminata L under drought stress is shown in Fig. 3. The MS media Supplemented with PEG at 2%, 4%, and 6% adversely affected the relative water content of M. acuminata L grandinin cv. compared with the control. The maximum adversely affected for induced drought stress was observed when plantlets of M. acuminata L grandinin cv. were cultured on MS media complemented with 6% of PEG compared with control and other treatments. It was clear that adding K2SiO3 at different concentrations from 2.0 mM up to 6.0 mM to the MS media caused an increase in RWC% compared the high level of (PEG 6%) without K2SiO3.
Figure 3: Effect of M. acuminata L grandinin cv. media supplemented with PEG individually or combined with K2SiO3 on RWC%. Numerical data are reported as mean ± SD
3.3 Effect of PEG and K2SiO3 on the Photosynthetic Pigments
The responses of the photosynthetic pigments data are illustrated in Fig. 4. In general, increased PEG concentrations from 2% up to 6% (w/v) led to a reduction in all photosynthetic pigments such as chlorophyll a (Fig. 4A), chlorophyll b (Fig. 4B), and carotenoids (Fig. 4D) compared to unstressed M. acuminata L grandinin cv media. There were no significant differences between the tested treatments and the control in relation to total chlorophyll (Fig. 4C). Furthermore, the maximum reduction in all the above-mentioned photosynthetic pigments was obtained when the plantlets of M. acuminata L grandinin cv. were cultured on MS media complemented with 6% of PEG compared with those of unstressed and the other treatments. In contrast, adding K2SiO3 at different concentrations from 2.0 up to 6.0 mM with PEG from 2% up to 6% to MS media led to accumulating all the biochemical characteristics mentioned above.
Figure 4: Effect of M. acuminata L grandinin cv. media supplemented with PEG either individually or in combination with K2SiO3 on biochemical characteristics including (A) chlorophyll a, (B) chlorophyll b, (C) total chlorophyll, and (D) carotenoids. Numerical data are reported as mean ± SD
In contrast, the maximum content of photosynthetic pigments was achieved when the plantlets of M. acuminata L grandinin cv. were cultured on MS media supplemented with 6.0 mM without any concentration of PEG and unstressed M. acuminata L grandinin cv. media (p ≤ 0.05). The increased concentrations of PEG from 2% up to 6% with low concentrations of K2SiO3 achieved the maximum reduction in all the biochemical characteristics mentioned above compared with those of the control and the other treatments.
3.4 Effect of PEG and K2SiO3 on the Proline Content
Under drought stress conditions, adding PEG from 2% to 6% caused an increase in the proline content of M. acuminata L grandinin cv. compared with unstressed one. The maximum proline content was obtained when the plantlets of M. acuminata L grandinin cv. media were cultured with 6% of PEG compared with the unstressed and the other treatments (Fig. 5). However, the proline content in the leaves of the tested cv decreased by adding Si to the MS media compared with those of control and PEG stressed. The severe reduction in proline content was achieved when the micro-shoots of the tested cv were cultured on MS media supplemented with 6 mM of K2SiO3 compared with PEG stressed (Fig. 5). On the other hand, a decrease in concentrations of K2SiO3 with increased PEG achieved an increase in the proline content compared with those of unstressed treatments (Fig. 5).
Figure 5: Effect of MS media supplemented with PEG either individually or in combination with K2SiO3 on the Proline content of M. acuminata L grandinin cv. Numerical data are reported as mean ± SD
3.5 Effect of PEG and K2SiO3 on the Antioxidant Enzymes Activities
The general tendency of the water stress induced by PEG led to a significant increase in the activity of the antioxidant enzymes activities like CAT, PPO, and POD. The rise in PEG from 2% to 6% caused an increase significantly in activity of CAT, PPO and POD (p ≤ 0.05) with an increased value of PEG compared with control (Fig. 6). Furthermore, culturing the micro-shoots of M. acuminata L on MS media supplemented with PEG and K2SiO3 caused an increase in CAT, PPO, and POD activity, especially with an increased value of both PEG and K2SiO3. On the other hand, the PPO, CAT, and POD activity decreased when adding low K2SiO3 levels alone (Fig. 6).
Figure 6: Effect of MS media supplemented with PEG either individually or in combination with K2SiO3 on M. acuminata L grandinin cv. enzymes activity
3.6 Effect of PEG and K2SiO3 on the Stomata Status
Responses of stomata morphology to drought stress and scanning electron microscope images are shown in Fig. 7. The drought stress induced by PEG affected stomata behavior such as internal length and width (µm), outside length and width (µm) (the guard cells), and the number of open or close stomata of M. acuminata L tested cv. When PEG from 2% up to 6% was added to the growth media, M. acuminata L showed a significant negative reduction in the internal length or width of stomata and guard cells. Most of the stomata were closed (Figs. 7B and 7E), in comparison with control (Figs. 7A and 7D). On the other hand, adding K2SiO3 at 2, 4, and 6 mM individually to MS media enhanced the status of stomata in leaves of M. acuminata L compared with the drought stress treatments. In contrast, MS media supplemented with all levels of PEG, either individually or in combination with of K2SiO3 especially at the low level of them, did not have a significant effect on length and width, as well as the density of stomata in leaves of M. acuminata L (Figs. 7C and 7F), in comparison with those of control and other treatments (Figs. 7A and 7D).
Figure 7: Effect of MS media supplemented with PEG either individually or in combination with K2SiO3 on stomata behavior of M. acuminata L grandinin cv. by SEM; (A and D) control, (B and E) PEG, PEG + K2SiO3 (F) and K2SiO3 at 6, 4 and 2 mM (C, G, H and I, respectively)
Data heat map analysis (Fig. 8) showed that treatment with PEG and the high it concentration reduced the morphological characteristics such as shoot number, shoot length, roots number and survival percentage and biochemical characteristics such as chlorophyll a, b, carotenoids, and RWC while proline content and the enzymatic activity of the antioxidant enzymes increased in leaf of M. acuminata L. On the contrary, it showed that treatment with K2SiO3 produced the highest morphological and biochemical characteristics in comparison with the control. The heat map also showed that the enzymatic activity of the antioxidant enzymes increased with increasing concentrations of PEG alone or with high concentrations of K2SiO3 together.
Figure 8: Cluster heat map analysis summarizing responses to drought stress by PEG at (0%, 2%, 4% and 6%) and the effect K2SiO3 at (0. 2, 4 and 6 mM) as anti-drought stress of M. acuminata L grandinin cv. growing in vitro
Previously, drought stress has been proven in various studies to impact plants’ morphological, physiological, biochemical, and molecular features [32]. Drought also disrupts electron flow, damaging the photosystem by causing oxidative stress and slowing photosynthesis [33]. In this study, drought stress decreased plant development indices such as shoots number, fresh weight, dry weight, and survival percentage compared to unstressed plants. These results are in line with the findings of [34], who reported that adding PEG to MS media had a negative effect on shoot length (cm), shoots number, leaves number, and shoot fresh weight (g), where increasing PEG from 1% to 3% possessed the lowest value of all previous parameters of the Grand Nain banana cultivar in comparison with control. Also, Sdek et al. [35] illustrated that adding PEG at 10, 20, 30, and 40 g/L to MS media caused the reduction in shoot height, leaves number, shoot fresh weight and shoot dry weight of two Musa spp. Banana cultivars, namely Grand Nain and Zeev, compared with control. In addition, MS media supplemented with PEG from 1 to 3% caused a reduction in proliferation rate and shoot fresh weight (g) of banana (M. acuminata cv. ‘Berangan’, AAA) compared with those of control. At the same time, the highest reduction in leaf proliferation rate and shoot fresh weight happened when shoot tips of banana (M. acuminata cv. ‘Berangan’, AAA) were cultured on MS supplemented with high value of PEG at 3% compared with those of control and other treatments [36]. The decrease in plant growth could be attributed to the inhibition of the rate of cell division and/or alternations of the cell cycle progression [37]. Drought stress may be handled by either generating a drought-tolerant genotype or discovering ways to mitigate the negative impacts of drought. Exogenous Si treatment is one of the most effective methods for alleviating drought stress in plants [38]. In contrast, regardless of the presence of drought stress, plants treated with potassium silicates exhibited significant improvements in all of the evaluated growth indices. So, Si plays a critical role in the defense response to various stresses (water, salts, etc.) in plant species [14]. Si is collected in cell wall apoplasts and plant tissues to create silica, preventing stressor penetration and tissue protection [39]. The inhibition of water loss from plant leaves by maintaining the plant’s hydration status might be one of the processes responsible for superior development in the presence of Si under stressful situations.
Furthermore, Si seems to be engaged in plant fortification toward cell membrane oxidation, resulting in the protection of many plant functions and structures during dry circumstances and hence preventing the penetration of stressors. Si seems to have a role in osmolyte control in drought-stressed cells. Increased gas exchange metrics such as transpiration, stomatal conductance, and improved plant development can be achieved by employing Si-based fertilizers (ex-vitro), according to Zhang et al. [40]. Upon drought stress, Si can impact relative water content, photosynthetic rate, chlorophyll content, plant growth, and other physiological responses [41]. Si-mediated drought stress reduction in higher plants is accomplished mainly by antioxidative defense system activation and osmotic adjustment. Also, creating a two-layer silica cuticle under the leaf epidermis protects the water status of plants in water deficits by limiting leaf transpiration [42]. Several researchers have reported that the observed decrease in leaf transpiration in plants treated with Si could be attributed to the formation of a silica cuticle double layer on leaf epidermal tissue [43,44]. Bidabadi et al. [36] revealed that relative water content in shoot tips of banana (M. acuminata cv. ‘Berangan’, AAA) was adversely influenced by PEG. The percentage of RWC was decreased when shoot tips were cultured on MS media supplemented with all concentrations of PEG. The high value of PEG gained the lowest percentage in RWC. In contrast, the highest percentage of RWC gained when shoot tips of banana (M. acuminata cv. ‘Berangan’, AAA) were cultured on MS media without PEG. A drop in relative water content causes stomata conductance, CO2 assimilation, and photosynthesis to be disrupted, among other physiological activities [45]. Si enhances root water absorption under water-stressed conditions by actively accumulating osmotic regulators such as amino acids and soluble sugars [46]. The chlorophyll content (chlorophyll a, B and carotenoids) of banana leaves was reduced as a result of water stress, while Si treatment retained the chlorophyll content and relieved the decline. Drought stress also affects photosynthetic pigments and causes a discrepancy in the antioxidant activity and production of ROS [47]. Both stomata closure and impairment of the photosynthetic mechanism restrict photosynthesis during drought (i.e., metabolic factors). Inherent water use efficiency (WUEi) improves during moderate drought and declines during more severe or long-term drought due to photosynthetic damage or blockage [48]. Mesophyll conductance decreases during long-term drought, reducing CO2 diffusion and decreasing photosynthesis in leaf of rice [49]. These results are in agreement with the findings of Haq et al. [50], who reported that MS media supplemented with 5% of PEG caused a non-significant decrease in chlorophyll a, while total chlorophyll contents and chlorophyll b were also decreased significantly for Basrai cultivar (Musa spp.) in comparison with control. Al-Mayahi [51] illustrated that MS media supplemented with 15% of PEG caused a reduction in chlorophyll content of Phoenix dactylifera L compared with control [51].
Chlorophyll is responsible for gathering lights in plants and has an essential role in the process of photosynthesis. Several factors have been reported led to a decrease in photosynthetic pigments such as deficiency of protein complexes, increased activity of chlorophyll degrading enzymes as well as increased oxidative stress which injury the chloroplast structure. The reduction in chlorophyll as a result of stress is correlated to the enhanced ROS production in the cells [52]. The ROS radicals led to disintegration, peroxidation, and diminution of chlorophyll content. In agreement with Nahar et al. the chlorophyll content was considerably diminished under drought stress [53]. Drought stress has been shown to lower chlorophyll concentrations, although Si treatment increased chlorophyll concentrations above those of untreated plants [54]. These findings are in accordance with other researchers of Al-Mayahi [51], who reported that adding 3.6 mM Si with 15% PEG to the MS growth media under drought stress caused an increase in chlorophyll contents of P. dactylifera L, contrasted with the stressful treatment (without Si treatment) [51]. Another study stated that the Si application improved the contents of chlorophyll in wheat [16]. The effects of application of Si could be attributed to maintaining the water percentage in leaves, thus avoiding the damage of the chlorophyll in leaves and photosynthetic process [55]. Also, investigated the Si effects on dry-matter production of ten rice cultivars and discovered that the positive effects were due to photosynthesis maintenance and protection of chlorophyll from destruction in ageing leaves. They also discovered that maintaining an appropriate water status in leaves may be an important aspect of the role of Si in the prevention of photosynthetic depression and chlorophyll destruction in leaves.
Moreover, the Si accumulation in the cell wall also improves the resistance of tissue and promotes plant performance as a result of their interception of light [56]. Besides, the observed decrease in the plants subjected to drought stress in the absence of Si where could be attributed to the reduction in nitrogen absorption which is essential for production and configuration of chlorophyll. Treatment with Si demonstrated to trigger alterations to the metabolism of nitrogen [57]. Stomata closure causes less CO2 uptake and ion toxicity in the chloroplasts, which results in less chlorophyll and chlorophyll degradation by ROS [58]. In addition, one of the essential components of plant tolerance to salt and drought conditions is maintaining the water status and chlorophyll content of the plant [59].
Proline is a non-essential amino acid which obtained by the phosphorylation of glutamate. Under drought-stressed conditions, the glutamine pathway is considered as the main route for biosynthesis of proline [60]. Other studies have linked proline accumulation to the recovery of growth markers, indicating that this amino acid is involved in preserving photosynthesis-related structures and activities [61]. Al-Mayahi [51] found that adding PEG at 15% to MS media caused an increase in proline content of P. dactylifera L compared with control Re-culturing the micro-shoots of P. dactylifera L on MS media supplemented with Si at 3.6 mM combined with 15% PEG led to a decrease in proline content compared with those of control and culture media supplemented with PEG only. In addition, Singh et al. [62] illustrated that adding PEG at 1% to 3% to the growth MS media caused an increase in proline content of two banana varieties viz. Grand naine and Nalla bontha compared with those of control and other treatments [62]. In Arabidopsis, higher levels of proline accumulation were linked to improved salt and drought resistance [63].
The inorganic and organic solutes accumulated in the cytosol of plants which raises the osmotic pressure, thus preserving both turgor and the driving gradient for water uptake [51]. Furthermore, proline modulates mitochondrial functions, which play a vital role in the recovery of plant from stress [64]. Increasing the concentration of PEG was also associated with a significant increase in the proline content and a decrease in water content in the date palm callus [65]. Even though both proline and carbohydrates were accumulated in response to water stress, Si administration controlled proline and carbohydrate production, resulting in significant reductions in their levels to alleviate the stress. In addition, Si treatment reduced proline levels in sorghum plants when they were under water stress [54].
The accumulation of enzymatic and non-enzymatic components that protect cell membranes and other substances is known according to [66]. To reduce oxidative damage, plants use enzymes such (CAT), Glutathione peroxidase (GPX), Superoxide dismutase (SOD), Guaranteed Reagent (GR), and Advanced Performance X (APX) [67]. Enzymatic components that play a crucial role in the defense system include superoxide (POD), (CAT), and (SOD). These results are consistent with Patade et al. who demostrated that MS media supplemented with PEG 4% increased the antioxidant enzymes activity of sugarcane (Saccharum officinarum L cv. Co 86032) calli compared to MS media without PEG [68]. Antioxidant enzymes play an essential role in drought tolerance and other abiotic stresses. Drought stress caused a formation of lipid peroxidation that induced membrane damage, protein degradation [69], and enzyme inactivation [70] via the ROS formation such as H2O2, free radicals, and hydroxyl radicals. The plant has an endogenous mechanism which protects the cellular and sub-cellular system from free radicals, produced from antioxidant enzymes such as peroxidase (POX), APX, CAT, and SOD, that can cause toxicity [71]. The improvement of water stress effects by adding Si, thus, increases the activity of antioxidant enzymes in plants by Si addition to MS media and thereby detoxifies ROS damage stimulated by drought stress [51]. Under diverse abiotic stress conditions, the exogenous Si application boosted the osmoprotectant level and the antioxidative activity of several enzymes [72]. It has been proposed that Si-induced stress tolerance in plants could be attributed to the enhanced antioxidant enzyme activity, that led to reduce oxidative damage to membranes and enzyme activity [73]. CAT is assumed to be the most abundant enzyme that scavenges H2O2, which is accumulated through the exposure of plants to abiotic stress and devastates the photosynthetic apparatus. It also led to impairment of normal metabolism in the course of oxidative damage of nucleic acids, lipids, and proteins. Also, PPO) and POD are the most efficacious antioxidant enzymes in prohibiting cell demolition [74].
Previous studies by Yadollahi et al. [75] demonstrated that the smaller stomata size in cultivated almonds could be associated to drought resistance. In the previous studies, P. haussknechtii showed the smallest stomata length in relation to drought stress compared with untreated with drought stress [75]. In addition, Serra et al. reported that stomata size and density are influenced by light and water constraints. Further, this study showed that different rootstock cultivars onto grape with the same scion grafted caused a various stomata sizes and densities [76]. Shortage of water can affect the development of stomata which resulted in a decrease of the diameter of pore. Various grapevine genotypes demonstrate a variety of stomata sensitivities to shortage of water, where some varieties be likely to close their stomata earlier than others [77]. The closure of stomata in advance of πTLP has been considered as a tolerance mechanism for drought stress which facilitate the plant to survive based on stored water [78]. In addition, Soundararajan et al. reported that MS media complemented with potassium silicate K2SiO3 at 1.8, or 3.6 mM enhanced the stomata morphological characteristic of Dianthus caryophyllus L compared with control. We recommend to adding potassium silicate with both fertigation system as a soil application or foliar spray application to increase the plant’s ability to withstand stress under in vivo condition in light of the scarcity of water [79].
This work provided evidence that some morphological characteristics and photosynthesis pigments such as chlorophyll a and b, RWC percentage, and proline content in the leaves of the tested cultivar were affected by the applied PEG treatments as a drought stress under tissue culture technique compared with control. MS media supplemented with PEG, especially at high levels, caused a reduction in both morphological and biochemical characteristics except proline content, which increased with increasing PEG concentrations compared with control. At the same time, adding PEG to MS media caused an increase in the leaf content of antioxidants such as CAT, POX, and PPO enzyme activity. On the other hand, adding K2SiO3 either individually or in combination with PEG to MS media caused an improvement in all morphological and biochemical characteristics and led to an increase in proline content and antioxidants such as CAT, POX, and PPO enzyme activity in the leaves of the tested cultivar, especially when adding K2SiO3 to MS media without any concentrations of PEG compared with control. The K2SiO3 compound was effective in reducing the degree of drought stress and in mitigating the adverse effects of water deficit. Thus, Si-particle has been considered a low-cost technology to mitigate the adverse effects of drought stress and enhance the growth of the tested cultivars, especially under water scarcity conditions.
Acknowledgement: The authors extend their appreciation to Princess Nourah bint Abdulrahman University for funding this work through the Researchers Supporting Project No. (PNURSP2022R188), Princess Nourah bint Abdulrahman University, Riyadh, Saudi Arabia. The authors also thank the excellent doctoral scholarship award by the Chinese Scholarship Council and a full scholarship from the Ministry of Higher Education of the Arab Republic of Egypt to Mohamed Sharaf.
Authorship:: Conceptualization, H.A., M.K., M.O., and M.T.; methodology, H.A., M.K., M.O., K.A., and A.A.E-Y; software, H.A., M.K., N.I.A., and K.A.; validation, H.A., M.K., M.O., K.A., and M.T.; formal analysis, H.A., M.K., M.O., A.A.E-Y, and N.I.A.; investigation, H.A., M.K., M.O., and M.T.; resources, K.A., and N.I.A.; data curation, K.A., A.A.E-Y, N.I.A., A.M.A., and M.T.; writing—original draft preparation, H.A., M.K., M.O., and M.T.; writing—review and editing, H.A., M.K., M.O., K.A., A.A.E-Y, N.I.A., and M.T.; visualization, K.A., A.A.E-Y, N.I.A., and M.T.; supervision, M.O., K.A., A.A.E-Y, N.I.A., and K.A.; project administration, H.A., M.K., M.O., and M.T.; funding acquisition, K.A., and N.I.A. All authors have read and agreed to the published version of the manuscript.
Funding Statement: This research was funded by Princess Nourah bint Abdulrahman University Researchers Supporting Project No. (PNURSP2022R188), Princess Nourah bint Abdulrahman University, Riyadh, Saudi Arabia.
Conflicts of Interest: The authors declare that they have no conflicts of interest to report regarding the present study.
References
1. Heslop-Harrison, J. S., Schwarzacher, T. (2007). Domestication, genomics and the future for banana. Annals of botany, 100(5), 1073–1084. DOI 10.1093/aob/mcm191.
2. Xiong, J., Zhang, L., Fu, G., Yang, Y., Zhu, C. (2012). Drought-induced proline accumulation is uninvolved with increased nitric oxide, which alleviates drought stress by decreasing transpiration in rice. Journal of Plant Research, 125(1), 155–164. DOI 10.1007/s10265-011-0417-y.
3. Stover, R. H., Simmonds, N. W. (1987). Classification of banana cultivars. Bananas, 3, 97–103.
4. Neumann, P. (1997). Salinity resistance and plant growth revisited. Plant, Cell & Environment, 20(9), 1193–1198. DOI 10.1046/j.1365-3040.1997.d01-139.x.
5. Stolker, J. M., McCullough, P. A., Rao, S., Inzucchi, S. E., Spertus, J. A. et al. (2010). Pre-procedural glucose levels and the risk for contrast-induced acute kidney injury in patients undergoing coronary angiography. Journal of the American College of Cardiology, 55(14), 1433–1440. DOI 10.1016/j.jacc.2009.09.072.
6. Zhang, F., Cui, F., Zhang, L., Wen, X., Luo, X. et al. (2014). Development and identification of a introgression line with strong drought resistance at seedling stage derived from Oryza sativa L. mating with Oryza rufipogon Griff. Euphytica, 200(1), 1–7.
7. Türkan, I., Bor, M., Özdemir, F., Koca, H. (2005). Differential responses of lipid peroxidation and antioxidants in the leaves of drought-tolerant P. acutifolius Gray and drought-sensitive P. vulgaris L. subjected to polyethylene glycol mediated water stress. Plant Science, 168(1), 223–231.
8. Landjeva, S., Neumann, K., Lohwasser, U., Börner, A. (2008). Molecular mapping of genomic regions associated with wheat seedling growth under osmotic stress. Biologia Plantarum, 52(2), 259–266.
9. Ober, E. S., Sharp, R. E. (2003). Electrophysiological responses of maize roots to low water potentials: Relationship to growth and ABA accumulation. Journal of Experimental Botany, 54(383), 813–824.
10. Matheka, J. M., Magiri, E., Rasha, A. O., Machuka, J. (2008). In vitro selection and characterization of drought tolerant somaclones of tropical maize (Zea mays L.). Biotechnology, 7(4), 641–650.
11. Turner, B. L., Lambin, E. F., Reenberg, A. (2007). The emergence of land change science for global environmental change and sustainability. PNAS, 104(52), 20666–20671. DOI 10.1073/pnas.0704119104.
12. Van Bockhaven, J., de Vleesschauwer, D., Höfte, M. (2013). Towards establishing broad-spectrum disease resistance in plants: Silicon leads the way. Journal of Experimental Botany, 64(5), 1281–1293. DOI 10.1093/jxb/ers329.
13. Wang, M., Wang, R., Mur, L. A. J., Ruan, J., Shen, Q. et al. (2021). Functions of silicon in plant drought stress responses. Horticulture Research, 8(1), 254. DOI 10.1038/s41438-021-00681-1.
14. Ma, J. F., Yamaji, N. (2006). Silicon uptake and accumulation in higher plants. Trends in plant science, 11(8), 392–397. DOI 10.1016/j.tplants.2006.06.007.
15. Epstein, E. (1999). Silicon. Annual Review of Plant Biology, 50(1), 641–664. DOI 10.1146/annurev.arplant.50.1.641.
16. Gong, H., Zhu, X., Chen, K., Wang, S., Zhang, C. (2005). Silicon alleviates oxidative damage of wheat plants in pots under drought. Plant Science, 169(2), 313–321.
17. Cooke, J., Leishman, M. R. (2011). Is plant ecology more siliceous than we realise? Trends in Plant Science, 16(2), 61–68.
18. Ma, J. F. (2004). Role of silicon in enhancing the resistance of plants to biotic and abiotic stresses. Soil Science and Plant Nutrition, 50(1), 11–18.
19. Simova-Stoilova, L., Demirevska, K., Petrova, T., Tsenov, N., Feller, U. (2008). Antioxidative protection in wheat varieties under severe recoverable drought at seedling stage. Plant Soil and Environment, 54(12), 529–536.
20. Uzilday, B., Turkan, I., Sekmen, A. H., Ozgur, R. E. N. G., Karakaya, H. C. (2012). Comparison of ROS formation and antioxidant enzymes in Cleome gynandra (C4) and Cleome spinosa (C3) under drought stress. Plant Science, 182, 59–70.
21. Sivanesan, I., Park, S. W. (2014). The role of silicon in plant tissue culture. Frontiers in Plant Science, 5(10), 571. DOI 10.3389/fpls.2014.00571.
22. Palla-Rubio, B., Araújo-Gomes, N., Fernández-Gutiérrez, M., Rojo, L., Suay, J. et al. (2019). Synthesis and characterization of silica-chitosan hybrid materials as antibacterial coatings for titanium implants. Carbohydrate Polymers, 203(2), 331–341. DOI 10.1016/j.carbpol.2018.09.064.
23. Camargo, S. J., Emanuel, K. A., Sobel, A. H. (2007). Use of a genesis potential index to diagnose ENSO effects on tropical cyclone genesis. Journal of Climate, 20(19), 4819–4834. DOI 10.1175/JCLI4282.1.
24. Garcı́a-Mata, C., Lamattina, L. (2001). Nitric oxide induces stomatal closure and enhances the adaptive plant responses against drought stress. Plant Physiology, 126(3), 1196–1204. DOI 10.1104/pp.126.3.1196.
25. Lichtenthaler, H. K., Buschmann, C. (2001). Chlorophylls and carotenoids: Measurement and characterization by UV-VIS spectroscopy. Current Protocols in Food Analytical Chemistry, 1(1), F4.3.1–F4.3.8. DOI 10.1002/(ISSN)2572-5602.
26. Bates, L. S., Waldren, R. P., Teare, I. D. (1973). Rapid determination of free proline for water-stress studies. Plant and Soil, 39(1), 205–207.
27. Chance, B., Maehly, A. C. (1955). [136] Assay of catalases and peroxidases. Methods in Enzymology, 2, 773–775.
28. Aebi, H. (1984). [13] Catalase in vitro. In: Methods in enzymology, vol. 105, pp. 121–126. Oxygen Radicals in Biological Systems: Academic Press.
29. Duckworth, H. W., Coleman, J. E. (1970). Physicochemical and kinetic properties of mushroom tyrosinase. Journal of Biological Chemistry, 245(7), 1613–1625.
30. Theodorou, N., Koundouras, S., Zioziou, E., Nikolaou, N. (2013). Responses of leaf stomatal density and anatomy to water deficit in four winegrape cultivars (Vitis vinifera L.). AMPELOS 2013-3rd International Symposium on Trends in World Vitiviniculture Development Conference, Adelaide, Australia.
31. Stern, R. D. (1991). CoStat-statutical software. California: CoHort software (1989pp. 302, $76.00. Experimental Agriculture, 27(1), 87. DOI 10.1017/S0014479700019232.
32. Kamal, K. Y., Khodaeiaminjan, M., Yahya, G., El-Tantawy, A. A., Abdel El-Moneim, D. et al. (2021). Modulation of cell cycle progression and chromatin dynamic as tolerance mechanisms to salinity and drought stress in maize. Physiologia Plantarum, 172(2), 684–695.
33. Ahammed, G. J., Li, X., Wan, H., Zhou, G., Cheng, Y. (2020). SlWRKY81 reduces drought tolerance by attenuating proline biosynthesis in tomato. Scientia Horticulturae, 270, 109444.
34. El-Mahdy, M. T., Abdel-Wahab, D. A., Youssef, M. (2021). In vitro morpho-physiological performance and DNA stability of banana under cadmium and drought stresses. Vitro Cellular & Developmental Biology-Plant, 57(3), 460–469.
35. Sdek, W. Z., Elazab, D. S., Aboul-Nasr, M. H., Elmahdy, T. K. (2017). Effect of salinity and drought stress on potassium uptake in Musa spp. in vitro. Assiut Journal of Agricultural Science, 48(3), 198–211.
36. Bidabadi, S. S., Ghobadi, C., Baninasab, B. (2012). Influence of salicylic acid on morphological and physiological responses of banana (‘Musa acuminata’ cv. ‘Berangan’, AAA) shoot tips to in vitro water stress induced by polyethylene glycol. Plant Omics, 5(1), 33–39.
37. Netondo, G. W., Onyango, J. C., Beck, E. (2004). Sorghum and salinity: II. Gas exchange and chlorophyll fluorescence of sorghum under salt stress. Crop Science, 44(3), 806–811.
38. Ning, D., Qin, A., Liu, Z., Duan, A., Xiao, J. et al. (2020). Silicon-mediated physiological and agronomic responses of maize to drought stress imposed at the vegetative and reproductive stages. Agronomy, 10(8), 1136.
39. Kim, S. G., Kim, K. W., Park, E. W., Choi, D. (2002). Silicon-induced cell wall fortification of rice leaves: A possible cellular mechanism of enhanced host resistance to blast. Phytopathology, 92(10), 1095–1103.
40. Zhang, C., Moutinho-Pereira, J. M., Correia, C., Coutinho, J., Gonçalves, A. et al. (2013). Foliar application of Sili-K® increases chestnut (Castanea spp.) growth and photosynthesis, simultaneously increasing susceptibility to water deficit. Plant and Soil, 365(1), 211–225.
41. Helaly, M. N., El-Hoseiny, H., El-Sheery, N. I., Rastogi, A., Kalaji, H. M. (2017). Regulation and physiological role of silicon in alleviating drought stress of mango. Plant Physiology and Biochemistry, 118, 31–44.
42. Zhang, Y., Yu, S. H. I., Gong, H. J., Zhao, H. L., Li, H. L. et al. (2018). Beneficial effects of silicon on photosynthesis of tomato seedlings under water stress. Journal of Integrative Agriculture, 17(10), 2151–2159.
43. Matoh, T., Murata, S., Takahashi, E. (1991). Effect of silicate application on photosynthesis of rice [Oryza sativa] plants. Japanese Journal of Soil Science and Plant Nutrition, 62(3), 248–251.
44. Gong, H. J., Chen, K. M., Chen, G. C., Wang, S. M., Zhang, C. L. (2003). Effects of silicon on growth of wheat under drought. Journal of Plant Nutrition, 26(5), 1055–1063.
45. Mostofa, M. G., Hossain, M. A., Fujita, M. (2015). Trehalose pretreatment induces salt tolerance in rice (Oryza sativa L.) seedlings: Oxidative damage and co-induction of antioxidant defense and glyoxalase systems. Protoplasma, 252(2), 461–475.
46. Sonobe, K., Hattori, T., An, P., Tsuji, W., Eneji, A. E. et al. (2010). Effect of silicon application on sorghum root responses to water stress. Journal of Plant Nutrition, 34(1), 71–82.
47. Rangani, J., Panda, A., Parida, A. K. (2020). Metabolomic study reveals key metabolic adjustments in the xerohalophyte Salvadora persica L. during adaptation to water deficit and subsequent recovery conditions. Plant Physiology and Biochemistry, 150, 180–195.
48. Bota, J., Tomás, M., Flexas, J., Medrano, H., Escalona, J. M. (2016). Differences among grapevine cultivars in their stomatal behavior and water use efficiency under progressive water stress. Agricultural Water Management, 164, 91–99.
49. Ouyang, W., Struik, P. C., Yin, X., Yang, J. (2017). Stomatal conductance, mesophyll conductance, and transpiration efficiency in relation to leaf anatomy in rice and wheat genotypes under drought. Journal of Experimental Botany, 68(18), 5191–5205.
50. Haq, I., Fatima, M., Shaikh, H., Dahot, M. U., Rajput, M. T. et al. (2011). Characteristics of micro-propagated banana (Musa spp.) cultures stressed with NaCl and polyethylene glycol. African Journal of Biotechnology, 10(21), 4387–4391.
51. Al-Mayahi, A. M. W. (2016). Effect of silicon (Si) application on Phoenix dactylifera L. growth under drought stress induced by polyethylene glycol (PEG) in vitro. American Journal of Plant Sciences, 7(13), 1711–1728.
52. Gill, S. S., Tuteja, N. (2010). Reactive oxygen species and antioxidant machinery in abiotic stress tolerance in crop plants. Plant Physiology and Biochemistry, 48(12), 909–930.
53. Nahar, K., Hasanuzzaman, M., Alam, M., Fujita, M. (2015). Glutathione-induced drought stress tolerance in mung bean: Coordinated roles of the antioxidant defence and methylglyoxal detoxification systems. AoB PLANTS, 7, plv069.
54. Yin, L., Wang, S., Liu, P., Wang, W., Cao, D. et al. (2014). Silicon-mediated changes in polyamine and 1-aminocyclopropane-1-carboxylic acid are involved in silicon-induced drought resistance in Sorghum bicolor L. Plant Physiology and Biochemistry, 80, 268–277.
55. Mali, M., Aery, A. N. C. (2008). Silicon effects on nodule growth, dry-matter production, and mineral nutrition of cowpea (Vigna unguiculata). Journal of Plant Nutrition and Soil Science, 171(6), 835–840.
56. Lana, R., Korndorfer, G., Zanao-Junior, L., Silva, A., Lana, A. (2003). Effect of calcium silicate on the productivity and silicon accumulation in the tomato plant. Bioscience Journal, 19, 15–20.
57. Watanabe, S., Fujiwara, T., Yoneyama, T., Hayashi, H. (2001). Effects of silicon nutrition on metabolism and translocation of nutrients in rice plants. In: Plant nutrition, pp. 174–175. Dordrecht: Springer.
58. Senay, S., Fikret, Y., Sebnem, K., Sebnem, E. (2011). The effect of salt stress on growth, chlorophyll content, lipid peroxidation and antioxidative enzymes of pumpkin seedling. African Journal of Agricultural Research, 6(21), 4920–4924.
59. Poór, P., Gémes, K., Horváth, F., Szepesi, A., Simon, M. L. et al. (2011). Salicylic acid treatment via the rooting medium interferes with stomatal response, CO2 fixation rate and carbohydrate metabolism in tomato, and decreases harmful effects of subsequent salt stress. Plant Biology, 13(1), 105–114.
60. Rady, M. M., Elrys, A. S., El-Maati, M. F. A., Desoky, E. S. M. (2019). Interplaying roles of silicon and proline effectively improve salt and cadmium stress tolerance in Phaseolus vulgaris plant. Plant Physiology and Biochemistry, 139, 558–568.
61. Silva, D. C. D., Melo, A. S. D., Melo, Y. L., Andrade, W. L. D., Lima, L. M. D. et al. (2019). Silicon foliar application attenuates the effects of water suppression on cowpea cultivars. Ciência e Agrotecnologia, 43. DOI 10.1590/1413-7054201943023019.
62. Singh, A., Shukla, P. K., Sengar, R. S., Mishra, P. (2021). In vitro effect of polyethylene glycol and sorbitol on two banana varieties viz. Grand naine and nalla bontha to study drought stress. Journal of Applied and Natural Science, 13(2), 482–490.
63. Li, G., Ye, Y. X., Ren, X. Q., Qi, M. Y., Zhao, H. Y. et al. (2020). The rice Aux/IAA transcription factor gene OsIAA18 enhances salt and osmotic tolerance in Arabidopsis. Biologia Plantarum, 64, 454–464.
64. Szabados, L., Savouré, A. (2010). Proline: A multifunctional amino acid. Trends in Plant Science, 15(2), 89–97.
65. Al-Khayri, J., Al-Bahrany, A. (2004). Growth, water content, and proline accumulation in drought-stressed callus of date palm. Biologia Plantarum, 48, 105–108.
66. Liu, M., Liu, R., Chen, W. (2013). Graphene wrapped Cu2O nanocubes: Non-enzymatic electrochemical sensors for the detection of glucose and hydrogen peroxide with enhanced stability. Biosensors and Bioelectronics, 45, 206–212.
67. Tripathi, A., Vázquez-Baeza, Y., Gauglitz, J. M., Wang, M., Dührkop, K. et al. (2021). Chemically informed analyses of metabolomics mass spectrometry data with Qemistree. Nature Chemical Biology, 17(2), 146–151.
68. Patade, V. Y., Bhargava, S., Suprasanna, P. (2012). Effects of NaCl and iso-osmotic PEG stress on growth, osmolytes accumulation and antioxidant defense in cultured sugarcane cells. Plant Cell, Tissue and Organ Culture (PCTOC), 108(2), 279–286.
69. Davies, K. J. (1987). Protein damage and degradation by oxygen radicals. I. General aspects. Journal of Biological Chemistry, 262(20), 9895–9901.
70. Fridovich, I. (1986). Biological effects of the superoxide radical. Archives of Biochemistry and Biophysics, 247(1), 1–11.
71. Larson, R. A. (1988). The antioxidants of higher plants. Phytochemistry, 27(4), 969–978.
72. Cao, B. L., Ma, Q., Xu, K. (2020). Silicon restrains drought-induced ROS accumulation by promoting energy dissipation in leaves of tomato. Protoplasma, 257(2), 537–547.
73. Sivanesan, I., Jeong, B. R. (2014). Silicon promotes adventitious shoot regeneration and enhances salinity tolerance of Ajuga multiflora bunge by altering activity of antioxidant enzyme. The Scientific World Journal, 2014, 521703.
74. Habibi, G., Hajiboland, R. (2013). Alleviation of drought stress by silicon supplementation in pistachio (Pistacia vera L.) plants. Folia Horticulturae, 25(1), 21–29.
75. Yadollahi, A., Arzani, K., Ebadi, A., Wirthensohn, M., Karimi, S. (2011). The response of different almond genotypes to moderate and severe water stress in order to screen for drought tolerance. Scientia Horticulturae, 129(3), 403–413.
76. Serra, I., Strever, A., Myburgh, P. A., Deloire, A. (2014). The interaction between rootstocks and cultivars (Vitis vinifera L.) to enhance drought tolerance in grapevine. Australian Journal of Grape and Wine Research, 20(1), 1–14.
77. Dayer, S., Herrera, J. C., Dai, Z., Burlett, R., Lamarque, L. J. et al. (2020). The sequence and thresholds of leaf hydraulic traits underlying grapevine varietal differences in drought tolerance. Journal of Experimental Botany, 71(14), 4333–4344.
78. Duursma, R. A., Blackman, C. J., Lopéz, R., Martin-StPaul, N. K., Cochard, H. et al. (2019). On the minimum leaf conductance: Its role in models of plant water use, and ecological and environmental controls. New Phytologist, 221(2), 693–705.
79. Soundararajan, P., Manivannan, A., Cho, Y. S., Jeong, B. R. (2017). Exogenous supplementation of silicon improved the recovery of hyperhydric shoots in Dianthus caryophyllus L. by stabilizing the physiology and protein expression. Frontiers in Plant Science, 8, 738.
Cite This Article
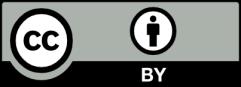