Open Access
ARTICLE
Tissue Culture of Calla Lily (Zantedeschia spreng.): An Updated Review on the Present Scenario and Future Prospects
1
Institute of Grassland, Flowers and Ecology, Beijing Academy of Agriculture and Forestry Sciences, Beijing, 100097, China
2
College of Horticultural Science & Technology, Hebei Key Laboratory of Horticultural Germplasm Excavation and Innovative
Utilization, Hebei Normal University of Science & Technology, Qinhuangdao, 066004, China
3
Department of Agroecology and Plant Health, The Robert H. Smith Faculty of Agriculture, Food and Environment, The Hebrew
University of Jerusalem, Rehovot, 7610001, Israel
4
Zhejiang Institute of Landscape Plants and Flowers, Zhejiang Academy of Agricultural Sciences, Hangzhou, 311251, China
* Corresponding Authors: Wenting Xu. Email: ; Zunzheng Wei. Email:
(This article belongs to the Special Issue: Tree Somatic Embryogenesis and Application)
Phyton-International Journal of Experimental Botany 2023, 92(8), 2413-2428. https://doi.org/10.32604/phyton.2023.029667
Received 02 March 2023; Accepted 28 April 2023; Issue published 25 June 2023
Abstract
The calla lily (Zantedeschia spreng.) is a bulbous flower native to the tropical regions of Africa. Calla lily has gained significant popularity in the international market owing to its intricate morphology and prolonged flowering duration. Despite such advantages, for two sub-groups of calla lily, known as group Zantedeschia and group Aestivae, there are challenges in terms of hybrid production due to the ‘plastome-genome incompatibility’ therebetween. Tissue culture is a fundamental biotechnological tool used in gene editing research, with a focus on disease resistance and flower color in calla lily breeding programs. The present review provides a brief background on the history and development of the calla lily, as well as a comprehensive and critical summary of calla lily tissue culture research. The regeneration pathways for both group Zantedeschia and group Aestivae can be divided into de novo organogenesis and somatic embryogenesis. Both groups are capable of obtaining replants through such means. However, only some species in group Aestivae have been reported to be successful in the somatic embryogenesis pathway. In the present review, special attention was paid to the influence of explant types, plant growth regulators, and culture conditions on both de novo organogenesis and somatic embryogenesis in calla lily tissue culture. Ultimately, future research prospects were determined based on integrated analysis of recent progress in calla lily tissue culture research.Keywords
Nomenclature
6-BA | N-(Phenylmethyl)-9H-purin-6-amine |
NAA | 1-Naphthylacetic acid |
KT | Kinetin |
IAA | Auxin |
TDZ | Thidiazuron |
2,4-D | 2,4-Dichlorophenoxyacetic acid |
IBA | 1H-indole-3-butyric acid |
PEM | Pre-embryogenic Mass |
SE | Somatic Embryo |
PGRs | Plant Growth Regulator |
The calla lily (Zantedeschia spreng.), a member of the Araceae family, is an herbaceous, bulbous, perennial ornamental flowering plant with a horseshoe-shaped spathe [1]. Calla lily is native to the tropical regions of the African continent, from the marshy plains of the coastal area of South Africa to the mountainous regions of Southeastern Africa. Zantedeschia, scientifically known as calla lily, consists of eight species classified into two sub-groups. The first subgroup, Zantedeschia, consists of two evergreen species, Z. aethiopica and Z. odorata, with white flowers that blossom from late winter to late spring in their natural habitat. The second subgroup, Aestivae, includes six species with colorful flowers that bloom in summer. Thousands of hybrids of the Aestivae group have been introduced worldwide [2]. While the former (hereafter referred to as white calla lily) is accessible on the market and is dominated by intraspecific hybrids of Z. aethiopica, the latter (hereafter referred to colored calla lily) is derived from interspecific or intraspecific hybrids of other species, including Z. elliottianna, Z. rehmannii, Z. albomaculata, Z. jucunda, Z. pentlandii, and others. Owing to their intricate morphology and prolonged flowering duration, both varieties of calla lily have witnessed a substantial surge in market turnover in recent years, thereby making them the most in-demand bulbous flowers in the global market.
As the need for calla lily in the market has increased, the issues associated with its commercial exploitation have become more noticeable. Consequently, breeders have started to prioritize the production of novel varieties with disease resistance and color-rich spathe. It has been widely reported that white calla lily can adapt to the humid environment during cultivation in greenhouses, and is more resistant to bacterial soft rot [3] despite the homogeneous flower color. In contrast, colored calla lily, despite the diverse flower colors, is highly susceptible to soft rot due to the poor tolerance to moisture [4]. Recently, Guttman et al. [3] characterized the morphological and biochemical differences between white and colored calla lilies. The findings suggest that such differences may account for the heightened susceptibility of colored calla lilies to soft rot following Pectobacterium spp. bacterial infection compared to their white counterparts. The study further revealed that in contrast to white calla lily, colored calla lily had rougher abaxial leaf surfaces, as well as more compact aerenchyma and fewer total air spaces in leaf tissue. Such factors contribute to the increased growth of soft rot bacteria. Moreover, the colored calla lily’s immune response and activation of defense-related genes against such bacteria were found to be hindered. Overall, the research facilitates a comprehensive understanding of the distinct characteristics of white and colored calla lilies that contribute to their divergent susceptibility to soft rot. Researchers have made significant attempts to cross-breed the white and colored varieties of calla lily, so as to merge the desirable qualities of both [5]. As an example, the disease resistance of white calla lily was introduced into the colored variant and the spathe color of colored calla lily was added to the white calla lily. However, the findings of the experiment demonstrated that there was ‘plastome-genome incompatibility’ between the two, producing albino hybrid seedlings [6], which failed to produce regular plants. As a result, the focus of calla lily breeding has shifted towards the already existing white and colored calla lily germplasm independently.
Molecular breeding techniques [7], including gene editing, are now available for early utilization in some of the most extensively studied ornamental plants. Such techniques have the ability to manipulate intrinsic genes, leading to the development of modified plants with new and improved agronomic traits [8]. In Ipomoea nil, for instance, the Dihydroflavonol-4-reductase-B (DFR-B) gene, which encodes anthocyanin biosynthetic enzymes, was targeted with CRISPR/Cas9 via Agrobacterium-mediated transformation to produce 24 (75% positive rate) transgenic plants with anthocyanin-free white flowers [9]. Additionally, attempts were made to induce mutations in the flavonoid 3-hydroxylase (F3H) gene, which encodes a critical enzyme in flavonoid synthesis, in Torenia fournieri [10]. The use of the CRISPR/Cas9 system to modify T0 plants resulted in a high frequency of light blue (almost white) flowers (about 80% of regenerated lines) [11]. Such findings present a potential opportunity to develop novel calla lily germplasm with exceptional targeted traits without using hybridization between white and colored calla lilies [9]. Research on the molecular biology and genetic transformation of calla lilies is still in its initial phases, and there are numerous obstacles to overcome. Nonetheless, with the availability of the calla lily genome (which has been sequenced and assembled at the chromosome level by the present group but has not yet been released) and the identification of candidate genes for critical phenotypic traits, transgenic gene editing has emerged as a vital avenue for significantly modifying genetic traits.
Plant in vitro regeneration via tissue culture is a critical component in genetic transformations mediated by A. tumefaciens, electroporation, and particle bombardment [12]. Such regeneration procedures usually include both de novo organogenesis (formation of shoots and roots in vitro from cultivated explants) [13] and somatic embryogenesis (generation of embryos from plant somatic cells without gamete fusion) [14]. The use of both regeneration procedures has been reported in the development of transgenic ornamentals [15]. For example, in Ornithogalum, somatic embryogenesis was used as a platform for transformation, the target plasmid was introduced into the embryonic callus derived from leaf base induction via particle bombardment, and disease-resistant transgenic plants were obtained after in vitro regeneration [16,17]. In two Lilium species (Lilium pumilum and L. longiflorum), two stable and effective genetic transformation protocols based on somatic embryogenesis and adventitious bud regeneration were established [18]. The hypothesis was that the likelihood of chimera formation in the genetic transformation was reduced and the transformation process was made more efficient [18]. Such findings could be attributed to the embryogenic callus being made up of a large number of embryogenic cells, each of which can develop into a somatic embryo on its own [19]. However, the genetic transformation by de novo organogenesis, such as adventitious bud regeneration, is faster as compared to the regeneration pathway via somatic embryogenesis [17].
With regard to addressing the prevailing breeding constraints in calla lily, the development of a highly efficient in vitro plant regeneration system is essential for the future implementation of gene editing-mediated molecular breeding. There have been many reports of calla lily regeneration in vitro, but the methods used have been found to be ineffective and inefficient. The present review covers the current efforts in calla lily in vitro regeneration, including the effects of various explants, phytohormones, and treatment conditions on the effectiveness of the process. The review can serve as a guide and a reference for the future application of molecular breeding programs for the improvement of calla lily varieties.
2 Summary of Tissue Culture Studies of Calla Lily
In the early 1990s, Wu et al. [20] conducted the first study on the in vitro regeneration of calla lily. The leaf of white calla lily was inoculated with additional auxin and cytokinin (NAA and KT) on MS medium and developed a callus after 10 days. Afterward, buds emerged and formed clusters of shoots within approximately three weeks. The shoots, which grew to a length of approximately 3 cm, were then transferred to a rooting medium to facilitate the development of complete plants. The most recent developments in in vitro tissue culture of calla lily are shown in Fig. 1A, while a summary of the research on calla lily de novo organogenesis and somatic embryogenesis since 1985 is shown in Fig. 1B. Notably, the research on somatic embryogenesis in calla lily was initiated later and has experienced less progress than de novo organogenesis. Detailed notes of the in vitro regeneration routes of the calla lily are shown in Fig. 1C; however, somatic embryogenesis has yet to be achieved.
Figure 1: Research on tissue culture of calla lily. (A) Historical progress of tissue culture research in calla lily; (B) Summary of study quantity; (C) Research results of tissue culture of calla lily
3 Advances in Organogenesis of Calla Lily
As has been repeatedly demonstrated, plants have extensive regeneration potential, with both inter- and intra-specific differences in their ability to divide and regenerate cells [21]. According to the existing research, de novo organogenesis in calla lily involves both direct organogenesis from thin-walled tuber cells and indirect organogenesis through the production of callus induced from tubers and leaves. There are several factors, including plant genotype, explant type, plant growth regulators (PGRs) and culture conditions that contribute to successful organogenesis [22]. The present review provides a comprehensive overview of the direct and indirect pathways involved in calla lily organogenesis.
3.1 Effects of Explants on Organogenesis
Various calla lily explants, including leaf, petiole, spathe, tuber, and anther, have been included in numerous experiments for the organogenesis process. Such studies suggest that although all vegetative cells possess the capacity to regenerate complete plants, the ease or difficulty of inducing such capacity may differ considerably [23].
In a case in which three distinct explants (petiole, leaf, and spathe) were employed to induce the callus, the petiole was revealed to possess the greatest dedifferentiation capacity following callus induction without the usage of PGRs (Table 1). The highest petiole callus induction rate [24] was maintained when all three explants were cultured on a medium supplemented with N-(Phenylmethyl)-9H-purin-6-amine (6-BA) and auxin (IAA). In previous studies, it was found that a significant proportion of leaves in the culture exhibited withering, while only a small number formed callus [25]. Additionally, it was observed that anthers used as explants were particularly susceptible to browning [26,27]. The utilization of seed as explants in the white calla lily variety ‘Green Goddess’ was found to require high concentrations of 6-BA stimulation to generate multiple young shoots [28]. However, due to a lack of repeatability with successful protocols in seed-as-explant experiments, the stability of such systems is uncertain. Contrastingly, tuber as explants has a high regenerative capacity in the organogenesis pathway [26]. In 2006, the anthers of four species of calla lily were used for the first time on a medium supplemented with 6-BA and 1-Naphthylacetic acid (NAA); all 1,380 anthers expanded but did not form callus, while callus was induced but eventually turned brown on a medium supplied with thidiazuron (TDZ) and NAA [27]. Further observations have shown that the combination of Kinetin (KT) and 2,4-D stimulated anthers to form callus more effectively [26]. One potential explanation for the varying responses of explants to different hormones is the differences in their endogenous hormone levels, which can be influenced by various exogenous hormones [28–32]. Based on the aforementioned findings, it can be concluded that 6-BA and NAA activate the indirect organogenesis pathway in all explants, except for anthers. Further, the system for inducing callus via tubers is the most established among the indirect organogenesis pathways and has exhibited the greatest stability across multiple studies.
In addition, the physiological state of the plant, as well as the type, size, source, sampling period, and inoculation orientation of the explants, are all significant factors that can influence in vitro tissue culture [35,36]. Findings were made that the induction efficacy of clumped shoots could be determined by calla lily tuber explants at different periods. For instance, the induction of dormant tubers was four to five times more effective than that of growing tubers, and the sterilizing duration may be decreased by 50% [37]. At the same time, the orientation of the explant on the medium may influence the initiation position, polarity, and regeneration efficiency of callus or shoots [38], with horizontal induction of explants on the medium being more efficient than vertical induction [39].
3.2 Effects of PGRs on Organogenesis
Plant organogenesis depends on the addition of PGRs and the response of plants to such hormones during tissue culture [40–43]. There are certain essential steps that must be completed in order to achieve the desired organogenesis and regeneration of plants, including the initiation of shoots, elongation of shoots, and initiation of roots. In contrast to direct organogenesis, indirect organogenesis entails the formation of a callus, which is subsequently differentiated into shoots, roots, or somatic embryos, depending on the type of PGR utilized [44]. Therefore, the selection of a particular PGR is closely associated with the fate of the explants [45].
The direct organogenesis pathway of calla lily induces clumps of buds primarily using 6-BA, TDZ, and KT. An experiment using three different types of PGRs (6-BA, TDZ, and KT) on tuber of colored calla lily revealed that a medium supplemented with 6-BA induced the highest adventitious bud proliferation rate [27]. In a separate investigation, the efficacies of four types of cytokinins (TDZ, 6-BA, KT, and 2iP) in inducing cluster buds were evaluated using stem tips of calla lily. The study revealed that 6-BA and TDZ were more effective in inducing cluster buds [34], and that increasing the concentration of 6-BA led to a greater number of cluster buds [46]. However, additional research has demonstrated that the growth-promoting effect of cytokinin tends to be restricted when cytokinin concentrations exceed the optimum level, leading to low shoot proliferation and stunted growth [47]. As an example, adding 6-BA to the tuber induction medium of colored calla lily at 0.5 mg/L resulted in the highest induction efficiency, but increasing to 3 mg/L resulted in a reduced induction rate [48]. Such trend is supported by other research results [49,50]. In a subsequent study, findings were made that low concentrations of 6-BA induced adventitious buds in explants, while high concentrations induced callus [51,52]. For instance, using colored calla lily stem tips cut from axillary buds, a medium supplemented with 1 mg/L 6-BA was used to form multiple buds, while the medium supplemented with 3 mg/L 6-BA effectively supported the growth of the callus [53].
Despite such findings, organogenesis in most plants is controlled by more than one type of hormone [54]. To illustrate, applying a certain concentration of 1H-indole-3-butyric acid (IBA) can increase the average number of axillary buds and significantly increase the bud length and fresh weight when 6-BA is the primary exogenous hormone in the induction stage of calla lily [55]. Under the combined treatments of 6-BA 1 mg/L and NAA 0.1 mg/L, the bush buds produced in the primary culture achieved simultaneous root and bud differentiation [56], which significantly decreased the culture period of in vitro seedlings and reduced the culture cost. Although there is evidence that 6-BA can stimulate callus production in calla lily, bud growth is not promoted. Notably, the addition of NAA can address such issue and induce bud differentiation [57]. In general, a culture containing 6-BA at a concentration of 2 mg/L and NAA at 0.5 mg/L for a duration of 60 days can lead to the development of a callus exhibiting a dense green bud point [58]. Such findings were confirmed in different varieties [59]. Auxin and cytokinin are central endogenous signaling molecules that regulate plant callus formation [60]. Generally, high auxin and low cytokinin concentrations are used to induce tissue mass dedifferentiation and maintain cells in a proliferative state [61,62]. RNA-Seq analysis of Anthurium andraeanum cv. ‘Alabama’ tissue revealed that, following a 2-day culture period, there was up-regulation of the auxin biosynthesis gene expression, while the expression of the cytokinin biosynthesis gene remained unchanged. Such findings suggest that the explants were unable to produce sufficient endogenous cytokinin required for the induction of callus [63]. Therefore, the addition of high concentration of cytokinin and low concentration of auxin to the medium is conducive to callus formation. For callus-inducing explants of calla lily, such method can be used to adjust the PGR ratio.
Currently, there is no consensus regarding the optimal exogenous hormones for inducing rooting, although most commonly used hormones belong to the auxin class, such as indole-3-acetic acid (IAA) and naphthaleneacetic acid (NAA) [64,65]. Studies have found that rooting can also be promoted without adding hormones in the culture environment of 1/2 MS medium and 200 mg/L activated carbon [55]. Many researchers have reported NAA to be the strongest auxin compared with others [58]. A number of existing studies on calla lily have shown that NAA exhibits a noticeable promoting effect on the rooting stage, as root initiation can only be stimulated by adding NAA to the rooting medium. However, an increase in the concentration of NAA results in a lengthening of the rooting induction period [64]. Therefore, the primary goal of the research on the initiation of root is to determine the proper concentration of NAA.
3.3 Effects of Genotype on Organogenesis
Regeneration in tissue culture is highly dependent on genotype, and tissue regenerative potential is determined empirically and varies from species to species [66]. Different genotypes of calla lily have different responses to PGRs. Changes in the concentration of PGRs and genotype of species can affect the number of buds produced by explants [67], and the use of PGRs in aseptic culture has a long-term effect on ontogeny [68]. Calla lily hybrids introduced into New Zealand (‘Pink Petticoat’, ‘Golden Sun’, ‘Galaxy’, ‘Red Beauty’, ‘Red Gold’, ‘Pink Persuasion’, ‘Golden Affair’ and ‘Pink Satin’) were found to be able to induce clumping shoots on differentiation medium. However, the quantity of clumping shoots varied depending on the variety, with ‘Galaxy’ being the highest producer of clumping shoots. Further findings were made that ‘Galaxy’ also demonstrated strong rooting ability throughout the rooting stage [33]. In subsequent studies that used tubers generated by tissue culture seedlings, the proliferation coefficients of tubers from 10 calla lily varieties showed significant differences, with yellow flower varieties having higher proliferation coefficients than safflower and purple flower [36]. To screen the optimal conditions for adventitious bud proliferation and plant regeneration, adventitious buds of three varieties of calla lily; ‘Purple’, ‘Flame’ and ‘Parfum’, were used as test materials. The stages of proliferation and rooting of the three varieties were found to be significantly different [64].
Colored calla lily is the subject of most studies on genotype differences, while white calla lily has fewer varieties and related studies. The genotype effect in calla lily tissue culture has been found to be largely influenced by the concentration of endogenous plant hormones in different varieties [64]. Several studies have shown that the gene expression pattern of endogenous PGRs and the balance between endogenous and exogenous PGRs are significant factors in in vitro culture [69]. A detailed study of the metabolism of endogenous PGRs in different varieties can reveal the dynamic inter-relationships therebetween and provide a new research direction for the establishment of regeneration systems in different varieties. At present, there is a scarcity of research on the endogenous hormone metabolism of different varieties of calla lily. As such, exploring the endogenous hormone metabolism of different genotypes of calla lily may become another direction of future research.
3.4 Other Important Influencing Factors
The majority of research has focused on the effects of PGRs and the type of explants and genotypes on calla lily organogenesis, which has resulted in a lack of information on many other factors. Therefore, analyzing other factors may lead to the invention of high-frequency regenerative systems or a reduction in production costs. In this section, a discussion is provided on several promising strategies for improving regeneration protocols. Carbohydrates serve as a source of energy for cell cultures and are the primary regulators of the osmotic environment. As a critical energy-supplying substance, both the source and concentration of carbohydrates are crucial factors that influence cell culture. Sucrose, glucose, and fructose have been extensively studied as the most important carbohydrates in Agave angustifolia [70], Pinus koraiensis [71], Vitis vinifera [72], Brassica napus [73], A. angustifolia [74] and P. koraiensis [75] exhibited the most favorable response to sucrose, while Brassica napus and V. vinifera exhibited a positive reaction to both glucose and fructose. Sucrose has mainly been discussed in terms of its effects on organogenesis in the calla lily. Findings were made that root length and number were significantly increased when the sucrose concentration ranged from 51.15–56.5 g/L [55]. The culture medium with sucrose concentrations ranging from 0–60 g/L, containing 30 and 60 g/L of sucrose, produced the highest number of leaves (4.13) and the greatest fresh weight (0.9 g) of the aboveground portion [56]. To summarize, sucrose can affect not only the rooting of adventitious buds, but also affect the growth of the aboveground parts in tissue culture of calla lily.
The source, intensity, and quality of light are pivotal in in vitro organogenesis [76,77]. A variety of physiological processes can be regulated through the use of light emitting diodes, which provide spectra with the capability of regulating plant morphogenesis [78,79]. Red light and blue light had a significant influence on plant phenotypes [63]. The regeneration process of the calla lily was affected differently by various light treatments. Under the same culture conditions, the number of calla lily leaves under blue light was higher than that under white light. Branch elongation and the fresh and dry weights of tufted shoots increased significantly under blue or red light [80].
In addition, the physical forms of medium and sterilization methods have also been partially studied in terms of the organogenesis process of calla lily. Such factors affect regeneration efficiency to varying degrees. Due to the difference in osmotic pressure between solid and liquid conditions, the physical state of the medium also affects the differentiation and rooting of explants. Several studies have shown that a solid medium is better than a liquid medium in the bud induction stage of calla lily, while there is no significant difference between the two conditions in the rooting stage [81]. In liquid culture conditions, tuft shoots of white calla lily exhibited the lowest water content. Sterilizing calla lily tubers for tissue culture has long been a challenging issue. Recent disinfection methods involved a hot water bath at different temperatures (30°C, 35°C, 40°C, 45°C, 50°C) and for different durations (30, 35 min). Among such methods, treatment at 45°C for 35 min was found to be the most effective, achieving a pollution rate reduction of less than 10% [82].
Research on somatic embryogenesis in calla lily is still in its early stages, with few related reports available. Most studies have focused on the indirect somatic embryogenesis pathway of calla lily, examining the effects of factors such as explants, plant growth regulators (PGRs), and other related factors on induction efficiency. In the study of the embryogenic callus of calla lily, three types of explants were used: leaf, tuber, and anther (Table 2). In 2006, the tuber of ‘Captain Tendens’ was first used to induce embryogenic callus and regeneration into a complete plant [29]. Subsequently, the tuber of ‘Gagsi’ was utilized as explants to produce calla lily embryogenic callus, and regenerated complete plants were obtained from such callus [26]. Although callus induction was observed in the leaves of ‘Pink Gian’, complete plant regeneration was not achieved. Additionally, an attempt was made to induce embryogenic callus using anthers in calla lily. However, only one explant out of 900 anthers inoculated developed a somatic embryo on medium containing 0.5 mg/L TDZ and 2 mg/L NAA, and all the callus that was obtained eventually turned brown [83].
The combination of auxin and cytokinin is required for the induction of embryogenic callus in the calla lily. The main PGRs affecting embryogenic callus development were 2,4-Dichlorophenoxyacetic acid (2,4-D), NAA, and 6-BA [64]. By applying 6-BA 1.5 mg/L and NAA 0.5 mg/L to the tuber of ‘Gagsi’ as explants in MS media, the embryogenic callus induction rate was 25% [26]. Leaves of ‘Pink Gian’ were induced by MS supplemented with 2,4-D 4.0 mg/L, 6-BA 0.5 mg/L, and NAA 0.2 mg/L, and the callus induced in the first generation was subjected to two rounds of subculture [27]. The results showed that MS containing 2,4-D 2 mg/L and 6-BA 2 mg/L was the optimal medium for callus subculture and promotion of their transformation into somatic embryos. The media containing 2 mg/L 6-BA and 2 mg/L NAA were found to be most effective in promoting embryogenic callus formation and regenerating normal plants. However, different combinations of concentrations eventually resulted in browning and death, without any somatic embryogenesis. The browning of explants is a natural phenomenon that occurs due to enzymatic oxidation of polyphenolic compounds. Notably, the oxidation process may potentially hinder the proliferation of callus in plant tissue culture [84,85]. Certain kinds of antioxidants such as Vitamin C or AgNO3 can be added to the culture medium to inhibit oxidative browning [86,87].
Although there is a scarcity of research on the somatic embryogenesis of calla lily, there have been many reports on somatic embryogenesis in other Araceae plants. The leaves of Anthurium andraeanum Lind, another member of the Araceae family, have been used to establish a stable and efficient system for somatic embryogenesis [88]. Such system utilizes leaves to induce embryogenic callus, which differentiates to form pre-embryogenic mass (PEM), and then develops into a somatic embryo. Maintaining the pre-embryonic mass in a homogenous developmental state makes the transformation process to somatic embryo smoother [89,90]. Moreover, a liquid culture can be used for propagation, in which PEM is used instead of embryonic callus or mature somatic embryo for proliferation [66]. In the study of the main factors affecting PEM proliferation, somatic embryo (SE) development and somatic embryo germination, findings have been made that in a liquid culture system, lower concentrations of plant growth regulators, mineral nutrients and sucrose promoted the proliferation of PEM, formation and germination of SE. A liquid medium containing 1/2 MS (half strength MS medium) + 2,4-D 2 mg/L + 0.5 mg/L KT + 3% sucrose produced the highest proliferation coefficient of leaves [58]. The proliferation curve of callus in liquid suspension culture typically exhibits an S-shape [83], which is more conducive to the proliferation of embryonic callus. By utilizing the inflorescence of the ‘fine wine’ variety as explants, it was observed that the optimal hormone combination for inducing embryogenesis of primary somatic cells in inflorescence was 5 mg/L 6-BA + 0.5 mg/L TDZ + 0.1 mg/L NAA [64,65].
The majority of studies have concentrated on the induction of callus and the development of embryogenic callus in colored calla lily, but there have been no relevant reports in white calla lily. To date, the research on somatic embryogenesis of calla lily is still in its initial phase, and extensive research is necessary to produce high-quality somatic embryos that can be directly utilized or transformed into artificial seeds for the reproduction of high-value hybrids.
5 Prospects of Future Research
Nanotechnology is a highly active area of research in modern materials science. Recent studies have demonstrated the effectiveness of NMs in the removal of microbial contaminants from explants. Further, nanomaterials have also been found to have beneficial effects on various processes in plant tissue culture, including somatic embryogenesis, callus induction, plant cell dedifferentiation and redifferentiation, somaclonal variation, genetic modification, and secondary metabolite production [86]. In the future, further investigations can be conducted into the improvement effect of nanomaterials on the tissue culture of calla lily. Additionally, activated charcoal is a critical factor in tissue cultures, promoting micro-propagule growth and development. Research has shown that activated charcoal supplementation in the culture medium can darken the medium to simulate the soil conditions and absorb toxic metabolites, thereby increasing shoot and root development in ornamental plants [87–89]. As such, the efficiency of tissue culture of calla lily can be improved by using activated carbon.
In white calla lily, regenerated plants using organogenesis were observed to be more reproducible and effective, and the regeneration duration was shorter than that of inducing embryogenic callus. As such, the organogenetic pathway can be used as the basis of a genetic transformation platform to introduce color genes and create new varieties of white calla lily with rich colors. However, a high reproduction coefficient of embryonic callus and fewer chimeras have natural advantages for genetic transformation in plants [91,92]. Further investigation into the somatic embryogenesis pathway is necessary to optimize the system and increase the efficiency of regeneration and subsequent genetic transformation in the white calla lily. Although there are few studies on embryogenic callus induction in calla lily, existing reports suggest that there is significant application potential in colored calla lily callus, and thus, further research is needed. In order to improve the disease resistance of calla lily, the indirect somatic embryogenesis pathway can be used as the basis of the genetic transformation system, and the susceptible genes can be knocked out by gene editing to obtain new varieties of calla lily that are resistant to soft rot. Fig. 2 depicts an upcoming genetic engineering breeding blueprint for two groups of calla lily species.
Figure 2: Schematic diagram of Agrobacterium-mediated gene transformation
The focus of the present study was on the main influencing factors of calla lily tissue culture, such as genotype, explant type, and PGRs, as well as other factors. Further, relevant studies of other Araceae species were reviewed to establish a foundation for calla lily tissue culture research, the development of a genetic transformation platform, and the generation of novel ideas for improving desirable traits.
Acknowledgement: The authors would like to extend their sincere appreciation to the National Natural Science Foundation of China, Beijing Academy of Agriculture and Forestry Sciences Specific Projects for Building Technology Innovation Capacity.
Funding Statement: This work was supported by the National Natural Science Foundation of China (32071812), Beijing Academy of Agriculture and Forestry Sciences Specific Projects for Building Technology Innovation Capacity (KJCX202000111/20230108).
Author Contributions: The authors confirm contribution to the paper as follows: constructed the manuscript outline: Zunzheng Wei and Wenting Xu; wrote the manuscript: Xuan Sun, Xue Wang, Bijay Sharma Subedi, Yin Jiang, Di Wang, Ronxin Gou, Guojun Zhang. All authors reviewed the results and approved the final version of the manuscript.
Conflicts of Interest: The authors declare that they have no conflicts of interest to report regarding the present study.
References
1. Earl, G. (1957). Chromosome number and speciation in the genus Zantedeschia. Plant Life, 655, 140–146. [Google Scholar]
2. Kubo, T., Inaba, K., Mori, G. (2006). Compatibility of interspecific hybridization in Zantedeschia. Journal of the Japanese Society for Horticultural Science, 75(3), 273–275. https://doi.org/10.2503/jjshs.75.273 [Google Scholar] [CrossRef]
3. Guttman, Y., Joshi, J. R., Chriker, N., Khadka, N., Kleiman, M. et al. (2021). Ecological adaptations influence the susceptibility of plants in the genus Zantedeschia to soft rot Pectobacterium spp. Horticulture Research, 8, 13. https://doi.org/10.1038/s41438-020-00446-2 [Google Scholar] [PubMed] [CrossRef]
4. Li, L., Yuan, L. F., Zhao, Y. R., Shi, Y. X., Chai, A. et al. (2022). Emergence of bacterial soft rot in calla lily caused by Pectobacterium aroidearum in China. Crop Protection, 152, 105854. https://doi.org/10.1016/j.cropro.2021.105854 [Google Scholar] [CrossRef]
5. Waleron, M., Misztak, A., Waleron, M., Franczuk, M., Jonca, J. et al. (2019). Pectobacterium zantedeschiae sp. nov. a new species of a soft rot pathogen isolated from Calla lily (Zantedeschia spp.). Systematic and Applied Microbiology, 42(3), 275–283. https://doi.org/10.1016/j.syapm.2018.08.004 [Google Scholar] [PubMed] [CrossRef]
6. Yao, J. L., Cohen, D., Rowland, R. E. (1995). Interspecific albino and variegated hybrids in the genus Zantedeschia. Plant Science, 109(2), 199–206. https://doi.org/10.1016/0168-9452(95)04163-O [Google Scholar] [CrossRef]
7. Zhu, H., Li, C., Gao, C. (2020). Applications of CRISPR-Cas in agriculture and plant biotechnology. Nature Reviews Molecular Cell Biology, 21(11), 661–677. https://doi.org/10.1038/s41580-020-00288-9 [Google Scholar] [PubMed] [CrossRef]
8. Quétier, F. (2016). The CRISPR-Cas9 technology: Closer to the ultimate toolkit for targeted genome editing. Plant Science, 242, 65–76. https://doi.org/10.1016/j.plantsci.2015.09.003 [Google Scholar] [PubMed] [CrossRef]
9. Watanabe, K., Kobayashi, A., Endo, M., Sage-Ono, K., Toki, S. et al. (2017). CRISPR/Cas9-mediated mutagenesis of the dihydroflavonol-4-reductase-B (DFR-B) locus in the Japanese morning glory Ipomoea (Pharbitis) nil. Scientific Reports, 7(1), 1–9. https://doi.org/10.1038/s41598-017-10715-1 [Google Scholar] [PubMed] [CrossRef]
10. Nitarska, D., Boehm, R., Debener, T., Lucaciu, R. C., Halbwirth, H. (2021). First genome edited poinsettias: Targeted mutagenesis of flavonoid 3’-hydroxylase using CRISPR/Cas9 results in a colour shift. Plant Cell, Tissue and Organ Culture, 147(1), 49–60. https://doi.org/10.1007/s11240-021-02103-5 [Google Scholar] [PubMed] [CrossRef]
11. Sedeek, K. E., Mahas, A., Mahfouz, M. (2019). Plant genome engineering for targeted improvement of crop traits. Frontiers in Plant Science, 10, 114. https://doi.org/10.3389/fpls.2019.00114 [Google Scholar] [PubMed] [CrossRef]
12. Nishihara, M., Higuchi, A., Watanabe, A., Tasaki, K. (2018). Application of the CRISPR/Cas9 system for modification of flower color in Torenia fournieri. BMC Plant Biology, 18(1), 331. https://doi.org/10.1186/s12870-018-1539-3 [Google Scholar] [PubMed] [CrossRef]
13. Regni, L., Facchin, S. L., da Silva, D. F., Proietti, P., Silvestri, C. et al. (2023). Optimization of the in vitro proliferation of an ancient pear tree cultivar (‘Decana d’inverno’) through the use of neem oil. Plants, 12(8), 1593. https://doi.org/10.3390/plants12081593 [Google Scholar] [PubMed] [CrossRef]
14. Bhatia, P., Ashwath, N., Senaratna, T., Midmore, D. (2004). Tissue culture studies of tomato (Lycopersicon esculentum). Plant Cell Tissue and Organ Culture, 78, 1–21. https://doi.org/10.1023/BTICU.0000020430.08558.6e [Google Scholar] [CrossRef]
15. Duclercq, J., Sangwan-Norreel, B., Catterou, M., Sangwan, R. S. (2011). De novo shoot organogenesis: From art to science. Trends in Plant Science, 16(11), 597–606. https://doi.org/10.1016/j.tplants.2011.08.004 [Google Scholar] [PubMed] [CrossRef]
16. Bassuner, B. M., Lam, R., Lukowitz, W., Yeung, E. C. (2007). Auxin and root initiation in somatic embryos of Arabidopsis. Plant Cell Reports, 26, 1–11. https://doi.org/10.1007/s00299-006-0207-5 [Google Scholar] [PubMed] [CrossRef]
17. Hesami, M., Baiton, A., Alizadeh, M., Pepe, M., Torkamaneh, D. et al. (2021). Advances and perspectives in tissue culture and genetic engineering of cannabis. International Journal of Molecular Sciences, 22(11), 5671. https://doi.org/10.3390/ijms22115671 [Google Scholar] [PubMed] [CrossRef]
18. Lipsky, A., Cohen, A., Ion, A., Yedidia, I. (2014). Genetic transformation of Ornithogalum via particle bombardment and generation of Pectobacterium carotovorum-resistant plants. Plant Science, 228, 150–158. https://doi.org/10.1016/j.plantsci.2014.02.002 [Google Scholar] [PubMed] [CrossRef]
19. Yan, R., Wang, Z., Ren, Y., Li, H., Liu, N. et al. (2019). Establishment of efficient genetic transformation systems and application of CRISPR/Cas9 genome editing technology in Lilium pumilum DC. Fisch. and Lilium longiflorum White Heaven. International Journal of Molecular Sciences, 20, 2920. https://doi.org/10.3390/ijms20122920 [Google Scholar] [PubMed] [CrossRef]
20. Wu, N., Chen, W. M. (1985). Regenerated plants by tissue culture of calla lily. Journal of Plants, 4, 34. [Google Scholar]
21. Niazian, M. (2019). Application of genetics and biotechnology for improving medicinal plants. Planta, 249(4), 953–973. https://doi.org/10.1007/s00425-019-03099-1 [Google Scholar] [PubMed] [CrossRef]
22. Sabbadini, S., Capriotti, L., Molesini, B., Pandolfini, T., Navacchi, O. et al. (2019). Comparison of regeneration capacity and Agrobacterium-mediated cell transformation efficiency of different cultivars and rootstocks of Vitis spp. via organogenesis. Scientific Reports, 9, 1–10. https://doi.org/10.1038/s41598-018-37335-7 [Google Scholar] [PubMed] [CrossRef]
23. Long, Y., Yang, Y., Pan, G., Shen, Y. (2022). New insights into tissue culture plant-regeneration mechanisms. Frontiers in Plant Science, 13, 926752. https://doi.org/10.3389/fpls.2022.926752 [Google Scholar] [PubMed] [CrossRef]
24. Jonytienė, V., Masienė, R., Burbulis, N., Blinstrubienė, A. (2017). Factors affecting Zantedeschia Spreng. dedifferentiation in vitro. Biologija, 63(4), 334–340. https://doi.org/10.6001/biologijav63i4.3608 [Google Scholar] [CrossRef]
25. Zhang, Z., Deng, B., Wu, Z, S., Hu, Y, S., Zhao, J. et al. (2012). Studies on tissue culture of Zantedeschia aethiopica. Journal of Anhui Agricultural Sciences, 30, 14645–14647+14699. https://doi.org/10.13989/j.cnki.0517-6611.2012.30.237 [Google Scholar] [CrossRef]
26. Gong, X. Q., Qu, F, N., You, C, R., Sun, W, T., Wang, M. (2008). Establishment of callus induction system from leaves in Zantedeschia. Journal of Yantai University (Natural Science and Engineering Edition), 74(3), 221–225. https://doi.org/10.13951/j.cnki.37-1213/n.2008.03.010 [Google Scholar] [CrossRef]
27. Wang, J. Z., Gao, W., Gao, X. H., Feng, Q., Sun, S. L. et al. (2005). Tissue culture of coloured common calla lily (Zantedeschia). Journal of Beijing University of Agriculture, 20(2), 10–13. https://doi.org/10.3969/j.issn.1002-3186.2005.02.003 [Google Scholar] [CrossRef]
28. Zhang, X. Y., Wu, Q. Q., Li, X. L., Zheng, S. X., Wang, S. M. et al. (2011). Haploid plant production in Zantedeschia aethiopica ‘Hong Gan’ using anther culture. Scientia Horticulturae, 129(2), 335–342. https://doi.org/10.1016/j.scienta.2011.03.045 [Google Scholar] [CrossRef]
29. Duquenne, B., Eeckhaut, T., Werbrouck, S., van Huylenbroeck, J. (2006). In vitro somatic embryogenesis and plant regeneration in Zantedeschia hybrids. Plant Cell Tissue and Organ Culture, 87(3), 329–331. https://doi.org/10.1007/s11240-006-9161-8 [Google Scholar] [CrossRef]
30. Ngamau, K. (2001). Development of an in vitro culture procedure using seeds from Zantedeschia aethiopica ‘Green Goddess’ as explants. Gartenbauwissenschaft, 66(3), 133–139. [Google Scholar]
31. Kumari, A., Baskaran, P., Plackova, L., Omamikova, H., Nisler, J. et al. (2018). Plant growth regulator interactions in physiological processes for controlling plant regeneration and in vitro development of Tulbaghia simmleri. Journal Plant Physiology, 223, 65–71. https://doi.org/10.1016/j.jplph.2018.01.005 [Google Scholar] [PubMed] [CrossRef]
32. Kosakivska, I. V., Vasyuk, V. A., Voytenko, L. V., Shchebatiuk, M. M., Romanenko, K. O. et al. (2020). Endogenous phytohormones of fern Polystichum aculeatum (L.) roth gametophytes at different stages of morphogenesis in vitro culture. Cytology and Genetics, 54, 23–30. https://doi.org/10.3103/S0095452720010089 [Google Scholar] [CrossRef]
33. Shi, S., Xue, J., Zhou, Z. P., Zhang, L. (2019). Optimization and adventitious bud induction of sterilization conditions of Zabtadeschia hybrida in different varieties. Molecular Plant Breeding, 17(3), 4398–4403. https://doi.org/10.13271/j.mpb.017.004398 [Google Scholar] [CrossRef]
34. Peng, F., Chen, Y. Y., Hao, R. M., Xia, B. (2006). Optimum on induction and proliferation of adventitious buds from Zantedeschia hybrida ‘Parfait’ in vitro. Journal of Plant Resources and Environment, 2, 47–49. https://doi.org/10.3969/j.issn.1674-7895.2006.02.011 [Google Scholar] [CrossRef]
35. Bidabadi, S. S., Jain, S. M. (2020). Cellular, molecular, and physiological aspects of in vitro plant regeneration. Plants, 9(6), 702. https://doi.org/10.3390/plants9060702 [Google Scholar] [PubMed] [CrossRef]
36. Lardon, R., Geelen, D. (2020). Natural variation in plant pluripotency and regeneration. Plants, 9(10), 1261. https://doi.org/10.3390/plants9101261 [Google Scholar] [PubMed] [CrossRef]
37. Fan, J. Q., Zhang, W. W., Zhang, N., Guo, W. M. (2005). In vitro culture and rapid propagation of several varieties of Zantedeschia spp. Journal of Nanjing Agricultural University, 28, 28–31. https://doi.org/10.7685/j.issn.1000-2030.2005.02.006 [Google Scholar] [CrossRef]
38. Li, F., Liu, S., Zeng, M. (2020). An efficient micropropagation protocol for Monochasma savatieri Franch. ex maxim through seed germination and direct shoot regeneration. In Vitro Cellular & Developmental Biology–Plant, 57, 30–38. https://doi.org/10.1007/s11627-020-10103-x [Google Scholar] [CrossRef]
39. Liu, Y., Lu, J. N., Zhu, H. B., Li, L. F., Shi, Y. Z. et al. (2016). Efficient culture protocol for plant regeneration from cotyledonary petiole explants of Jatropha curcas L. Biotechnology & Biotechnological Equipment, 30(5), 907–914. https://doi.org/10.1080/13102818.2016.1199971 [Google Scholar] [CrossRef]
40. Zhang, J. J., Yang, Y. S., Lin, M. F., Li, S. Q., Tang, Y. et al. (2017). An efficient micropropagation protocol for direct organogenesis from leaf explants of an economically valuable plant, drumstick (Moringa oleifera Lam.). Industrial Crops and Products, 103, 59–63. https://doi.org/10.1016/j.indcrop.2017.03.028 [Google Scholar] [CrossRef]
41. Kumar, N., Vijay Anand, K., Reddy, M. P. (2010). In vitro plant regeneration of non-toxic Jatropha curcas L.: Direct shoot organogenesis from cotyledonary petiole explants. Journal of Crop Science and Biotechnology, 13(3), 189–194. https://doi.org/10.1016/j.jplph.2018.01.005 [Google Scholar] [PubMed] [CrossRef]
42. Jiménez, V. M. (2005). Involvement of plant hormones and plant growth regulators on in vitro somatic embryogenesis. Plant Growth Regulation, 47, 91–110. https://doi.org/10.1007/s10725-005-3478-x [Google Scholar] [CrossRef]
43. Zhang, S. F., Yan, S. S., An, P. Q., Cao, Q., Wang, C. et al. (2021). Embryogenic callus induction from immature zygotic embryos and genetic transformation of Larix kaempferi 3× Larix gmelinii. PLoS One, 16(10), e0258654. https://doi.org/10.1371/journal.pone.0258654 [Google Scholar] [PubMed] [CrossRef]
44. Yang, E. D., Zheng, M. Y., Zou, X., Huang, X. L., Yang, H. Y. et al. (2021). Global transcriptomic analysis reveals differentially expressed genes involved in embryogenic callus induction in Drumstick (Moringa oleifera Lam.). International Journal of Molecular Sciences, 22(22), 12130. https://doi.org/10.3390/ijms222212130 [Google Scholar] [PubMed] [CrossRef]
45. Xiong, Y. P., Wei, Z. P., Yu, X. C., Pang, J. H., Zhang, T. et al. (2021). Shoot proliferation, embryogenic callus induction, and plant regeneration in Lepturus repens (G. Forst.) R. Br. In Vitro Cellular & Developmental Biology-Plant, 57, 1031–1039. https://doi.org/10.1007/s11627-021-10183-3 [Google Scholar] [CrossRef]
46. Chang, H. S., Chakrabarty, D., Hahn, E. J., Paek, K. Y. (2003). Micropropagation of calla lily (Zantedeschia albomaculata) via in vitro shoot tip proliferation. In Vitro Cellular Developmental Biology-Plant, 39, 129–134. https://doi.org/10.1079/Ivp2002362 [Google Scholar] [PubMed] [CrossRef]
47. Liu, Y., Liu, Y., He, Y., Yan, Y., Yu, X. et al. (2023). Cytokinin-inducible response regulator SlRR6 regulates plant height through gibberellin and auxin pathways in tomato. Joural of Experimental Botany, erad159. https://doi.org/10.1093/jxb/erad159 [Google Scholar] [PubMed] [CrossRef]
48. Kubo, T., Mori, G., Oda, M., Konagai, M. (2002). Multiple proliferation of Zantedeschia hybrida ‘Hazel Mary’ by shoot tip culture of axillary buds. Journal of the Japanese Society for Horticultural Science, 71(1), 123–126. https://doi.org/10.2503/jjshs.71.123 [Google Scholar] [CrossRef]
49. He, J, R., Wu, J., Yuan, N., Yan, W, S., Pu, Z. et al. (2008). Tissue culture and rapid propagation of Zantedeschia antedschia. Southwest China Journal of Agricultural Sciences, 21(3), 775–778. https://doi.org/10.16213/j.cnki.scjas.2008.03.056 [Google Scholar] [CrossRef]
50. Zhang, T. Q., Li, R. Q., Wang, Y. Z., Wang, F. H. (2005). Effects of cytokinin on micro-tuber production of color calla lily in vitro. Journal of Beijing Forestry University, 27(3), 108–111. https://doi.org/10.13332/j.1000-1522.2005.03.024 [Google Scholar] [CrossRef]
51. Hashemidehkordi, E., Mortazavi, S. N., Azadi, P. (2021). An efficient in vitro propagation protocol of pot calla lily (Zantedeschia spp cv. Orania and Sunclub) via tuber production. International Journal of Horticultural Science and Technology, 8, 343–351. https://doi.org/10.22059/ijhst.2021.317458.436 [Google Scholar] [CrossRef]
52. Ruiz-Sifre, G., Rosa-Márquez, E., Flores-Ortega, C. (1996). Zantedeschia aethiopica propagation by tissue culture. Journal of Agriculture-University of Puerto Rico, 80, 193–194. [Google Scholar]
53. Hlophe, N. P., Moyo, M., Van Staden, J., Finnie, J. F. (2015). Micropropagation of Zantedeschia Aethiopica (L.) Spreng.: Towards its commercial use in the cut flower industry. Propagation of Ornamental Plants, 15(2), 73–78. [Google Scholar]
54. Schwarz, O. J., Beaty, R. M. (2018). “Organogenesis” in plant tissue culture concepts and laboratory exercises, pp. 125–138. Oxford, UK: Routledge. [Google Scholar]
55. Kulpa, D. (2016). Micropropagation of calla lily (Zantedeschia rehmannii). Folia Horticulturae, 28(2), 181–186. https://doi.org/10.1515/fhort-2016-0021 [Google Scholar] [CrossRef]
56. Singh, M., Rathore, M. S., Choudhary, K., Shekhawat, N. S. (2009). Direct shoot bud formation and tuberization from aseptically cultured root tubers of calla lily (Zantedeschia aethiopica L). Journal of Plant Biochemistry and Biotechnology, 18(2), 203–207. https://doi.org/10.1007/Bf03263320 [Google Scholar] [CrossRef]
57. Wang, Y., Zou, H., Lin, J. B., Dai, Y. M. (2012). Study on tissue culture and rapid propagation of Zantedeschia hybida ‘Gaoyuan’. Journal of Agriculture of the University of Puerto Rico, 2(2), 42–45+78. [Google Scholar]
58. Lin, R., Wang, X. Q., Wang, R. Z., Yao, J. (1989). Tissue culture and rapid propagation of Zantedeschia hybrid. Guihaia, 9(2), 97–102. [Google Scholar]
59. Bernula, D., Benkő, P., Kaszler, N., Domonkos, I., Valkai, I. et al. (2020). Timely removal of exogenous cytokinin and the prevention of auxin transport from the shoot to the root affect the regeneration potential of Arabidopsis roots. Plant Cell, Tissue and Organ Culture, 140, 327–339. https://doi.org/10.1007/s11240-019-01730-3 [Google Scholar] [CrossRef]
60. Liu, Y., Tong, X., Hui, W. K., Liu, T., Chen, X. et al. (2015). Efficient culture protocol for plant regeneration from petiole explants of physiologically mature trees of Jatropha curcas L. Biotechnology Biotechnological Equipment, 29(3), 479–488. https://doi.org/10.1080/13102818.2015.1013308 [Google Scholar] [CrossRef]
61. Wang, L. X., Xu, Y. P., Liu, J. Q., Luo, X., Zhang, S. S. et al. (2021). Cytokinins affect shoot regeneration and modulate the expression of IPT and CKX genes of in vitro cultured Eleocharis dulcis (Brum. f.) Trin. The Journal of Horticultural Science and Biotechnology, 96(3), 330–337. https://doi.org/10.1080/14620316.2020.1829503 [Google Scholar] [CrossRef]
62. Ebrahim, M. K. H. (2004). Comparison, determination and optimizing the conditions required for rhizome and shoot formation, and flowering of in vitro cultured calla explants. Scientia Horticulturae, 101(3), 305–313. https://doi.org/10.1016/j.scienta.2003.11.002 [Google Scholar] [CrossRef]
63. Gliožeris, S., Tamošiūnas, A. (2008). Rooting of micropropagated calla lily. Propagation of Ornamental Plants, 8(3), 164–166. [Google Scholar]
64. Luo, Z. Z., You, C. R., Zhang, H., Liu, D., Kong, Y. H. et al. (2017). Optimization of adventitious bud proliferation and regeneration system of different color calla lily. Jiangsu Agricultural Sciences, 45(11), 38–41. https://doi.org/10.15889/j.issn.1002-1302.2017.11.010 [Google Scholar] [CrossRef]
65. Zhang, J. Y., Yang, X. H., Li, H., Wen, Z. W. (2010). Application in tissue culture of the colored common calla of orthogonal design. Chinese Agricultural Science Bulletin, 26(15), 71–74. https://doi.org/10.11924/j.issn.1000-6850.2008-0486 [Google Scholar] [CrossRef]
66. Abdalla, N., El-Ramady, H., Seliem, M. K., El-Mahrouk, M. E., Taha, N. et al. (2022). An academic and technical overview on plant micropropagation challenges. Horticulturae, 8(8), 677. https://doi.org/10.3390/horticulturae8080677 [Google Scholar] [CrossRef]
67. Su, Y. H., Liu, Y. B., Bai, B., Zhang, X. S. (2015). Establishment of embryonic shoot-root axis is involved in auxin and cytokinin response during Arabidopsis somatic embryogenesis. Frontiers in Plant Science, 5, 792. https://doi.org/10.3389/fpls.2014.00792 [Google Scholar] [PubMed] [CrossRef]
68. Centeno, M. L., Rodriguez, R., Berros, B., Rodriguez, A. (1997). Endogenous hormonal content and somatic embryogenic capacity of Corylus avellana L. cotyledons. Plant Cell Reports, 17(2), 139–144. https://doi.org/10.1007/s002990050367 [Google Scholar] [PubMed] [CrossRef]
69. Didi, D. A., Su, S., Sam, F. E., Tiika, R. J., Zhang, X. (2022). Effect of plant growth regulators on osmotic regulatory substances and antioxidant enzyme activity of Nitraria tangutorum. Plants, 11(19), 2559. https://doi.org/10.3390/plants11192559 [Google Scholar] [PubMed] [CrossRef]
70. Litz, R. E. (1993). Organogenesis and somatic embryogenesis. II International Symposium on In vitro Culture and Horticultural Breeding, 336, 199–206. https://doi.org/10.17660/ActaHortic.1993.336.25 [Google Scholar] [CrossRef]
71. Hesami, M., Naderi, R., Tohidfar, M., Yoosefzadeh-Najafabadi, M. (2019). Application of adaptive neuro-fuzzy inference system-non-dominated sorting genetic Algorithm-II (ANFIS-NSGAII) for modeling and optimizing somatic embryogenesis of Chrysanthemum. Frontiers in Plant Science, 10, 869. https://doi.org/10.3389/fpls.2019.00869 [Google Scholar] [PubMed] [CrossRef]
72. Zhang, G. F., Liu, J., Liu, C., Zhang, L. (2017). Study on induction of somatic embryogenesis in inflorescence of Spathiphyllum kochii. Jiangsu Agricultural Sciences, 45(23), 38–41. https://doi.org/10.15889/j.issn.1002-1302.2017.23.011 [Google Scholar] [CrossRef]
73. Ye, X., She, M., Wang, K., Xu, H. (2012). Identification, cloning, and potential application of genes related to somatic embryogenesis in plant tissue culture. Acta Agronomica Sinica, 38(2), 191–201. https://doi.org/10.3724/SP.J.1006.2012.00191 [Google Scholar] [CrossRef]
74. Hou, J. Y., Mao, Y. J., Su, P. F., Wang, D. C., Chen, X. et al. (2020). A high throughput plant regeneration system from shoot stems of Sapium sebiferum Roxb., a potential multipurpose bioenergy tree. Industrial Crops and Products, 154, 112653. https://doi.org/10.1016/j.indcrop.2020.112653 [Google Scholar] [CrossRef]
75. Chen, J. R., Wu, L., Hu, B. W., Yi, X., Liu, R. et al. (2014). The influence of plant growth regulators and light quality on somatic embryogenesis in China rose (Rosa chinensis Jacq.). Journal of Plant Growth Regulation, 33, 295–304. https://doi.org/10.1007/s00344-013-9371-3 [Google Scholar] [CrossRef]
76. Li, H., Xu, Z., Tang, C. (2010). Effect of light-emitting diodes on growth and morphogenesis of upland cotton (Gossypium hirsutum L.) plantlets in vitro. Plant Cell, Tissue and Organ Culture, 103, 155–163. https://doi.org/10.1007/s11240-010-9763-z [Google Scholar] [CrossRef]
77. Miler, N., Kulus, D., Woźny, A., Rymarz, D., Hajzer, M. et al. (2019). Application of wide-spectrum light-emitting diodes in micropropagation of popular ornamental plant species: A study on plant quality and cost reduction. In vitro Cellular & Developmental Biology-Plant, 55, 99–108. https://doi.org/10.1007/s11627-018-9939-5 [Google Scholar] [CrossRef]
78. Batista, D. S., Felipe, S. H. S., Silva, T. D., de Castro, K. M., Mamedes-Rodrigues, T. C. et al. (2018). Light quality in plant tissue culture: Does it matter? In Vitro Cellular & Developmental Biology-Plant, 54, 195–215. https://doi.org/10.1007/s11627-018-9902-5 [Google Scholar] [CrossRef]
79. Naor, V., Kigel, J., Ziv, M. (2004). Hormonal control of inflorescence development in plantlets of calla lily (Zantedeschia spp.) grown in vitro. Plant Growth Regulation, 42, 7–14. [Google Scholar]
80. Ribeiro, M. D. O., Pasqual, M., Silva, A., Rodrigues, V. A. (2008). Different salt concentrations of MS environment and sucrose on the in vitro multiplication of Zantedeschia aethiopica L. spreng. Revista Ciencia Agronomica, 39, 101–106. [Google Scholar]
81. Yip, M. K., Huang, H. E., Ger, M. J., Chiu, S. H., Tsai, Y. C. et al. (2007). Production of soft rot resistant calla lily by expressing a ferredoxin-like protein gene (pflp) in transgenic plants. Plant Cell Reports, 26(4), 449–457. https://doi.org/10.1007/s00299-006-0246-y [Google Scholar] [PubMed] [CrossRef]
82. Han, I. S., Kim, J. B. (2019). Establishment of a regeneration system for the production of calla plants (Zantedeschia spp.) via embryogenic callus culture. Journal of Plant Biotechnology, 46(1), 32–36. https://doi.org/10.5010/JPB.2019.46.1.032 [Google Scholar] [CrossRef]
83. Zhao, S., Wang, H., Liu, K., Li, L., Yang, J. et al. (2021). The role of JrPPOs in the browning of walnut explants. BMC Plant Biology, 21(1), 9. https://doi.org/10.1186/s12870-020-02768-8 [Google Scholar] [PubMed] [CrossRef]
84. Alagarsamy, K., Shamala, L. F., Wei, S. (2018). Influence of media supplements on inhibition of oxidative browning and bacterial endophytes of Camellia sinensis var. sinensis. 3 Biotech, 8(8), 356. https://doi.org/10.1007/s13205-018-1378-9 [Google Scholar] [PubMed] [CrossRef]
85. Raghavan, V. (2004). Role of 2,4-dichlorophenoxyacetic acid (2,4-D) in somatic embryogenesis on cultured zygotic embryos of Arabidopsis: Cell expansion, cell cycling, and morphogenesis during continuous exposure of embryos to 2,4-D. American Journal of Botany, 91(11), 1743–1756. https://doi.org/10.3732/ajb.91.11.1743 [Google Scholar] [PubMed] [CrossRef]
86. Yadollahi, A., Abdollahi, M. R., Moieni, A., Danaee, M. (2011). Effects of carbon source, polyethylene glycol and abscisic acid on secondary embryo induction and maturation in rapeseed (Brassica napus L.) microspore-derived embryos. Acta Physiologiae Plantarum, 33, 1905–1912. https://doi.org/10.1007/s11738-011-0738-4 [Google Scholar] [CrossRef]
87. Wang, G. D., Xu, C. Y., Yan, S., Xu, B. (2019). An efficient somatic embryo liquid culture system for potential use in large-scale and synchronic production of Anthurium andraeanum seedlings. Frontiers in Plant Science, 10, 29. https://doi.org/10.3389/fpls.2019.00029 [Google Scholar] [PubMed] [CrossRef]
88. Su, Y. H., Zhao, X. Y., Liu, Y. B., Zhang, C. L., O’Neill, S. D. et al. (2009). Auxin-induced WUS expression is essential for embryonic stem cell renewal during somatic embryogenesis in Arabidopsis. Plant Journal, 59(3), 448–460. https://doi.org/10.1111/j.1365-313X.2009.03880x [Google Scholar] [CrossRef]
89. Fki, L., Masmoudi, R., Drira, N., Rival, A. (2003). An optimised protocol for plant regeneration from embryogenic suspension cultures of date palm, Phoenix dactylifera L., cv. Deglet Nour. Plant Cell Reports, 21(6), 517–524. https://doi.org/10.1007/s00299-002-0558-5 [Google Scholar] [PubMed] [CrossRef]
90. Silva, C. M. D., Dias, R. D. S., de Melo, N. F. (2021). The effect of temperature and growth regulators on callus induction in watermelon anthers. Brazilian Archives of Biology and Technology, 64, e21180505. https://doi.org/10.1590/1678-4324-2021180505 [Google Scholar] [CrossRef]
91. Sun, X., Wang, Y., Yang, T., Wang, X., Wang, H. et al. (2022). Establishment of an efficient regeneration and Agrobacterium transformation system in mature embryos of calla lily (Zantedeschia spp.). Front in Genetics, 13, 1085694. https://doi.org/10.3389/fgene.2022.1085694 [Google Scholar] [PubMed] [CrossRef]
92. Tamimi, S. M., Othman, H. (2021). Callus induction and regeneration from germinating mature embryos of wheat (Triticum aestivum L.). Sains Malaysiana, 50(4), 889–896. https://doi.org/10.17576/jsm-2021-5004-01 [Google Scholar] [CrossRef]
Cite This Article
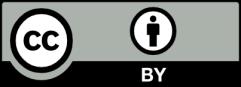