Open Access
ARTICLE
Inoculation of Chlorella and Food Waste Improves the Physio-Morphological Features of Red Pepper by Regulating Activating Antioxidant Defense System
1 Department of Applied Biosciences, Kyungpook National University, Daegu, 41566, Korea
2 Biosafety Division, National Institute of Agriculture Science, Rural Development Administration, Jeonju, 54874, Korea
3 Department of Technical Support, Daegu City Agricultural Technology Center, Daegu, 41159, Korea
4 Department of Chemical and Life Sciences, Qurtuba University of Science and Information Technology, Peshawar, Pakistan
5 Mountain Research for Field Crops Khudwani, Shere-e-Kashmir University of Agriculture Sciences and Technology Srinagar, Jamu and Kashmir, India
6 Corporate Affiliated Research Institute, Seyen Co., Ltd., Kyungsan, 38561, Korea
* Corresponding Authors: Won-Chan Kim. Email: ; In-Jung Lee. Email:
# These authors equally contribute to this work
(This article belongs to the Special Issue: Comprehensive Effects of Biochar or other Additives after Applied to Agricultural and Forest Soils)
Phyton-International Journal of Experimental Botany 2023, 92(9), 2699-2711. https://doi.org/10.32604/phyton.2023.028224
Received 08 December 2022; Accepted 28 February 2023; Issue published 28 July 2023
Abstract
Food waste is recognized as a valuable source for potential agricultural applications to supply organic matter and nutrients to arable soil. However, the information on the combined application of food waste and the plant growth-promoting bacterial strain, Chlorella, related to plant metabolic features and sodium chloride content in arable soil is limited. The present study was conducted to investigate the exogenous application of food waste along with Chlorella, which improved the physio-morphological features of red pepper. Our results revealed that this combination enhanced the organic matter in the soil, ultimately improving the fertility rate of the soil, and the physio-morphological features, such as chlorophyll a content (24.5 ± 0.7), root (7.8 ± 0.7) cm and shoot length (12.1 ± 0.7) cm, fresh weight (2.1 ± 0.05) g, dry weight (0.19 ± 0.05) g, mineral contents, and hormonal concentration (ABA by up to 2 folds). The combined treatment also minimized free radicals via the activation of the intrinsic antioxidant series cascade and electrolyte leakage. Our findings showed that adding Chlorella and food wastes improved growth characteristics and can be used as a green bio-fertilizer for sustainable agriculture.Keywords
Food waste is defined as the unconsumed or leftover portion of food [1,2]. Globally, one-third of food is wasted at various stages of production and marketing [3]. Gustavsson et al. [4] reported that over 1 billion tons of food waste are produced annually. Households produce 42% of food waste, manufacturing industries make up 39%, food service sectors are responsible for 14%, and the distribution process is responsible for 5% of food west [5]. Global food waste is expected to rise to 416 million tons by 2025, owing to rapid population growth in Asian countries [6]. According to reports, Japan generates almost 19 million tons, Taiwan produces 16.5 million tons, and Korea produces over 22% (4.3 million tons) annually of food waste [7–9]. Food waste has been identified as a valuable source of organic matter and nutrients for agricultural applications in arable soils. Composting is a modest, inexpensive, and regular degradation biological process that can transform food waste into nutrient-rich food for plants to improve and stabilize soil properties. Food waste contains nutrients that agriculture can reuse to enhance soil properties, growth, and productivity. Furthermore, the constituents of food waste include biological organic and inorganic compounds that oblige food for microbes; therefore, combining bio-fertilizer with organic fertilizers can be beneficial, and plants grow faster owing to plant root–microbe interactions [10,11]. Additionally, using food waste with Chlorella amendments as organic fertilizers can help improve soil chemical properties by bio-remediating pesticide and hydrocarbon-contaminated soils and alleviating salinity problems [12,13].
Many bio-stimulants, including purified seaweed extract, have been used in agriculture in recent years. However, microalgae have received insufficient attention as potential sources of plant bio-stimulants. Chlorella has also been investigated as a bio-fertilizer because it is high in carbohydrates, proteins, and growth hormones and is a bio-stimulant in conventional agriculture [14]. Plants have exhibited numerous reactions to microalgae inoculation, including increased growth and yield, improved nutrient acquisition, and tolerance to abiotic stresses, including salinity stress [15,16]. Microalgal treatment reduces the salinity levels in tomatoes by increasing the enzymatic activity of antioxidant enzymes [15]. Synthetic chemical fertilizers are a vital source of nourishment for crops; however, their scarcity, low plant uptake, and overuse have resulted in severe ecological imbalances. The magnitude of their effect on environmental stress has surpassed its limit [17]. Various agrochemicals are reported as carcinogenic and lethal for the environment and human health as well [18–20]. Our research team previously used commercial food waste with rhizobacteria to enhance the salinity tolerance in Chinese cabbage [5,21]. In light of these findings, we designed an experimental study to investigate the potential of food waste powder and plant growth-promoting microorganism (PGPM) Chlorella as a biologically safe fertilizer technique for improving crop growth and development. In the current study, we treated red pepper seedlings with Chlorella and food waste dry powder separately and together and examined their morphological and physiological parameters. Its impact on endogenous hormonal regulation and mineral contents was also investigated.
Red peppers were purchased from Syngenta Inc. (Switzerland) (commercial cultivar ‘Dokyacheongcheong’) and were then sown in a germination tray containing horticultural soil for 21 days. For the current study, the organic fertilizer was mixed with food waste in dry powder form, using two different concentrations (2g/pot FW2) (4g/pot FW4) and was then incorporated into the upper 10 cm of the soil and supplemented with various amounts of food waste according to the experimental design. Chlorella was distributed from the Agricultural Technology Center. The pots were appropriately labeled and divided into six groups; after three weeks, growth parameters such as seedling length and biomass were investigated.
2.1 Physiochemical Properties of Soil
To determine the physiochemical properties of soil, the detailed method of [22] was followed. In brief, 15–20 g of soil samples were collected for the field, and impurities were removed. The obtained soil sample was subject to air-dried and sieved through a 4-mm mesh to obtain clean and fine soil for further investigation. 5 g of the obtained soil sample was diluted with 25 ml of deionized water, followed by vortex for 5 min, and incubated at room temperature at continuous shaking for 1 h. After that, the pH and electrical conductivity were measured using pH and EC meters, respectively.
2.2 Endogenous Abscisic Acid Quantification
The endogenous ABA was extracted and quantified through gas chromatography-mass spectrophotometry (GC-MS) following the detailed methodology as previously described by [23] with slight modifications. Briefly, 0.5 g of sample was diluted with 10 mL of extraction buffer and was then subjected to shaking (130–140 rpm) at room temperature for 2 h. After shaking, the sample was then subjected to vacuum filtration and was diluted four times with a 5 mL extraction buffer. The obtained supernatant was treated with 50 ng of ABA standard before being evaporated in a rotary evaporator to a minimum of 2 mL. A 2 mL of 1 N (sodium hydroxide) NaOH was added to RBF and sonicated before being transferred to the long test tube. The pH was adjusted to 12.5, and 4 mL of dichloromethane was added to an empty RBF and sonicated before being transferred to respective test tubes, followed by vertex, and allowing to form layers.
The obtained upper layers were acidified by lowering the pH to 2.5–3.5, followed by adding 4 mL acetyl acetate three times for solvent-solvent partition through vertexing. The upper layer was then subjected to vacuum dry, and a 1 g of PVPP was added to the new flask while the RBF was washed three times with 4 mL of phosphate buffer pH 8.0, sonicated, and then transferred to the respective flask, followed by shaking for 1,30 Hr and they were the cleaned twice with 5 mL of P buffer. The filtrate pH was adjusted to a range of 2.5 to 3.5, and added ethyl acetate was three times, and vortexed again three times; the top layer was removed and added DW pH with 2.5 to the ethyl acetate vortex, and thrower away the bottom part. The solution was dried to 1 mL before transferring it to RBF. One ml was transferred to small vials and dried under an N-dryer. After that, 1 mL of 100% ethyl acetate was added twice and sonicated. Sixty uL of diazomethane was added, vortexed, and subjected to incubation in the dark at room temperature for 30 min. After incubation for 30 min, the vials were dried using N-dry gas; this incubation with diazomethane was repeated two times. Finally, the vials were diluted with 20 µL of dichloromethane and injected into GC-MS (6890N network gas chromatograph, Agilent Technologies), software to monitor signal ions (m/z 1162 and 190 for Me-ABA and m/z 166 and 194 for Me-[2H6]-ABA).
2.3 Measurement of Different Antioxidants
The antioxidant activity was estimated using the method described by Adhikari et al. 2020 [24]. Briefly, to quantify SOD, a 0.2 g of the plant samples were added to 5 mL of extraction buffer (5 mM Tris HCl (60 mL) + 40 mL EDTA (10 mL) and were then centrifuged at 10,000 xg for 10 min. A 50 uL of the obtained supernatant was diluted by the addition of 150 µL of extraction buffer + 50 µL of pyrogallol to record A values, and 50 uL of supernatant was added 200 µL of extraction buffer to record B values, while a 50 uL of supernatant was added 150 uL of extraction buffer + 100 µL of pyrogallol to record C values at an absorbance wavelength of 420 nm.
To measure flavonoid content, 0.2 g of plant samples were extracted with NaNO2, followed by centrifuge for 10 mint at 10000 rpm. The obtained supernatant was then diluted with 60 µL of 10% AlCl3, and 1 M sodium hydroxide was added. The absorbance was measured at 510 nm, as described by Adhikari et al. [25].
The 1,1-diphenyl-2-picrylhydrazyl (DPPH) scavenging activity was determined as described by Blois [26] with modifications. Briefly, 0.2 g of sample was extracted using 90% ethanol followed by centrifuge for 10 mint at 10000 rpm. The obtained supernatant was then diluted with 0.5 mM DPPH and 100 mM acetate buffer pH 5.5. The absorption was measured using a spectrophotometer at 517 nm and deliberated using the following equation:
To measure LPO lipid peroxidation levels, a 0.2 g of freeze-dried samples were diluted with 10 mM phosphate buffer pH 7.8% and 5% TCA and followed by centrifuge at 15,000 rpm for 12 min at 4°C. After centrifugation, 1 ml of supernatant and 2 ml of 0.5% TBA was added and incubated at room temperature for one hour, and then absorbance was measured at 532 nm.
For MDA quantification, a 0.2 g of grounded fresh leaves were combined with 5 mL of 5% TCA solution, followed by centrifuging at 10,000 xg for 10 min; the obtained 100 uL of supernatant was added to 1 mL TBA reagent and heated in a water bath at 45°C or 10 mins, and cooled immediately in a refrigerator, later centrifuged again at 10,000 xg for 10 mins and 250 uL of supernatant was taken, the absorbance was read as follows.
R1 at 530 nm, R2 at 600 nm, and the final value were obtained using MDA equation R3 (R1–R2) as the absolute value.
For Chlorophyll a, 0.2 g of freeze-dried grounded shoot sample was extracted by adding 150 uL of 80% methanol and centrifuged at 10,000 xg for 10 min, 200 uL of supernatant was removed and read at 470 nm.
2.4 ICP Analysis of Ion Uptake
The ion uptake was determined using a previously described method [27]. In a microwave, freeze-dried powdered plant samples of 0.1 g were mixed with 3 ml of nitric acid and 2 ml of H2O2. The samples were injected using inductively coupled plasma mass spectrometry (ICP-MS).
The experiment was repeated thrice, and each replicate contained 20 plants. GraphPad Prism was used for graphical representations. One-way ANOVA in MS Excel determined the statistical difference between treatment means. Duncan’s multiple range test (DMRT) was used to determine critical values for comparison between the mean of treatments at a significance level of p < 0.05. Statistical Analysis System (SAS 9.1) was used for the DMRT Analysis. For the graphical representation, we used GraphPad Prism software (version 6.0, San Diego, CA, USA).
3.1 Physiochemical Properties of the Soil
The results of the current study indicate that the application of food waste significantly improved soil electrolytic conductivity. As shown in Fig. 1a significant increase in EC was found in FW4 (1.4 mS/m) and decreased in FW2 (0.8 mS/m: No, FW2 was lower than the control) treated plants when compared to control plants. Moreover, further addition of Chlorella slightly increases the EC level by (0.7 mS/m) when compared to the control. However, the combined application of FW and CR significantly increases the EC level by 133.3%, compared to the control plant followed by the sole application of FW and chlorella (Fig. 1A). Similarly, the application of FW lowers the soil pH to 3.0–3.5, when compared to control plants, while the combined application of FW + CR significantly balances soil pH to optimum ranges between 3.8–4.0, which is the optimum level for the availability of most nutrients. These parameters further improve the soil’s physiochemical properties and nutrient availability (Fig. 1B).
Figure 1: Effect of FW + CR on physiochemical properties of soil. NT as control, FW2 and FW4 as food waste, CR as Chlorella, and FW2 + CR, FW4 + CR as combinations. (A) Effect of food waste and Chlorella on soil electrolytic conductivity (B) Effect of food waste and Chlorella on soil pH. Each bar represents the mean (±SE) with two replicates. Means with different letters are significantly different at p ≤ 0.05 between two replicates
3.2 Inoculation of Chlorella Regulates Pepper Physiological Growth under Food Waste Treatment
The current results showed that food waste application (FW2 and FW4) reduced pepper plants’ growth characteristics (Table 1). As plants treated with FW4 significantly decreased the shoot length (15.9 ± 2.50 cm), root length (21.9 ± 2.65 cm) shoot fresh weight (11.6 ± 3.13 g), dry weight (1.8 ± 0.68 g), and stem thickness (4.4 ± 0.43 mm), when compared to control plants (Fig. 2). While, combine inoculation of Chlorella along with food waste enhances the morphological features in red pepper plant such as increases in shoot length (28 ± 0.91 cm), root length (30 ± 3.37 cm), fresh shoot weight (15.6 ± 1.60 g), shoot dry weight (2.7 ± 0.44 g) and stem thickness (5.7 ± 0.31 mm).
Figure 2: Effect of FW + CR on Chlorophyll a content. NT as control, FW2 and FW4 as food waste, CR as Chlorella, and FW2 + CR, FW4 + CR as combinations. Each bar represents the mean (±SE) with two replicates. Means with different letters are significantly different at p ≤ 0.05 between two replicates
3.3 Effect of Food Waste on Endogenous ABA
Red pepper plants treated with food waste alone and in combination with Chlorella were further investigated to quantify endogenous ABA. The current results show that sole FW2 and FW4 cause a significant increase in the ABA content by 41.8% and 67.5%, when compared to control plants. However, the sole CR and FW2 + CR application shows a significant reduction in ABA content by 39.7% compared FW4 treated plant, followed by the combined application of FW4 + CR (Fig. 3).
Figure 3: Effect of FW + CR on ABA content. NT as control, FW2 and FW4 as food waste, CR as Chlorella and FW2 + CR, FW4 + CR as combinations. Each bar represents the mean (±SE) with two replicates. Means with different letters are significantly different at p ≤ 0.05 between two replicates
3.4 Antioxidant Quantification in Red Pepper Plants Treated with Food Waste
Major ROS-scavenging enzymes, such as SOD, PPO, MDA, and DPPH, and phytochemicals, such as flavonoids and phenolics, were investigated to assess their influence on food waste and on the regulation of the endogenous antioxidant defense system of red pepper. The current results indicate that a slight increase in the SOD, MDA, El, and DPPH content was found in the plant treated with sole CR application, whereas a significant decrease was found in the total flavonoids and phenolic content by sole CR application when compared to control plants (Figs. 4–7). On the other hand, FW2 and FW4 application cause significant increases in the SOD (21.5%), MDA (8.6% and 17.3%), EL (9.8% and 14.1%), DPPH (24.1%), total flavonoids (15.5%), and phenolic content (19.6%) compared to control plants. Were the combined application FW2 + CR and FW4 + CR significantly reduced this increase in the SOD (9.1% and 13%), MDA (15.4% and 7.3%), EL (13.2% and 7.1%), total flavonoids (11.2% and 16.1%), and phenolic content (8.6% and 12.4%) when compared to sole FW application. However, in the case of DPPH, the FW2 + CR significantly increased its content by 12.1% and 22.4% compared to FW-treated and control plants, while the FW4 + CR shows a significant reduction in the DPPH content by 7.5% and 16.7% when compared to control and FW treated plants (Fig. 6B).
Figure 4: Effect of FW + CR on antioxidant activity. NT as control, FW2 and FW4 as food waste, CR as Chlorella, and FW2 + CR, FW4 + CR as combinations. (A) Effect of food waste and Chlorella on SOD content. Each bar represents the mean (±SE) with two replicates. Means with different letters are significantly different at p ≤ 0.05 between two replicates
Figure 5: Effect of FW + CR on MDA content. NT as control, FW2 and FW4 as food waste, CR as Chlorella, and FW2 + CR, FW4 + CR as combinations. Each bar represents the mean (±SE) with two replicates. Means with different letters are significantly different at p ≤ 0.05 between two replicates
Figure 6: Effect of FW + CR on Electrolytic leakage NT as control, FW2 and FW4 as food waste, CR as Chlorella and FW2 + CR, and FW4 + CR as combinations. (A) Effect of food waste and Chlorella on soil electrolytic leakage (B) Effect of food waste and Chlorella on free radical scavenging. Each bar represents the mean (±SE) with two replicates. Means with different letters are significantly different at p ≤ 0.05 between two replicates
Figure 7: Effect of FW + CR on phytochemical contents. NT as control, FW2 and FW4 as food waste, CR as Chlorella, and FW2 + CR, FW4 + CR as combinations. (A) Effect of food waste and Chlorella on total flavonoid contents (B) Effect of food waste and Chlorella on total phenolic contents. Each bar represents the mean (±SE) with two replicates. Means with different letters are significantly different at p ≤ 0.05 between two replicates
3.5 ICP Analysis of Na, K, P, and Ca Content
The accumulation of different elements such as Ca, Na, and K was investigated through ICP analysis (Fig. 8). The current results show a significant increase in the Na content by FW2 (21.5%) and FW4 (48.6%) compared to control plants, where the sole CR and combine FW2 + CR application shows a slight decrease in Na content compared to control plant (Fig. 8A). in the case of K content, its level was significantly reduced in the FW4 (19.3%), CR (11.2%), and FW4 + CR (11.6%) treated plant compared to control plants, where the FW2 + CR application cause a significant increase in the K content by 13.8% compared to control plants (Fig. 8B). Moreover, the Ca content shows a significant increase by the FW2 (10%) and FW4 (8.6%) treated plants compared to control plants, where the sole CR and combine application of FW and CR cause significant reduction in the Ca content by 8% and 12% when compared to a sole FW treated plants (Fig. 8C).
Figure 8: Effect of FW + CR on Na, Ca, and K content. NT as control, FW2 and FW4 as food waste, CR as Chlorella, and FW2 + CR, FW4 + CR as combinations. (A) Effect of food waste and Chlorella on sodium content (B) Effect of food waste and Chlorella on potassium content (C) Effect of food waste and Chlorella on calcium content. Each bar represents the mean (±SE) with two replicates. Means with different letters are significantly different at p ≤ 0.05 between two replicates
Although cities and households generate food waste in significant quantities, proper management remains a challenge [28–30]. Hence, turning food waste into resources and energy may help achieve economic and environmental sustainability [31]. Organic waste has been reported to facilitate Chlorella growth, which is consequently used for various bio-resource products and aids with plant growth [32–34]. Our experimental results suggested that the combination treatment of Chlorella and food waste dry powder considerably improved the morphological and physiological features of crops. Our findings align with those of Chew et al. [35], who reported that food waste compost could be used as an organic medium for microalgae cultivation, which could aid organic matter in the soil. These findings demonstrated the feasibility of using food waste as a fertilizer source. However, there are several disadvantages, such as the high salt content in food, which can cause salinity stress and soil sodification [36,37]. As a result, we used Chlorella, a microbe that we believe could protect the crop against salt stress and soil sodification. Counterintuitively, when monitoring the mineral content of pepper plants, we discovered that Chlorella treatment with food waste powder reduced the Na content in the plants while improving other metabolic features, including endogenous phytohormones, antioxidants, and critical nutrient elements such as Ca++ and K+. Composting produces stable organic matter rich in humic compounds and essential plant nutrients that can be utilized as organic fertilizers [38]. Food waste can be a vital source of composting materials and organic fertilizers to aid organic components in the soil [21]. In the current study, we observed that the combined application returned the pH level of the soil to a normal state. Furthermore, the application of Chlorella marginally reduced the soil EC content. These findings highlight the importance of food waste and Chlorella treatment in soil health management, which might be owing to enhanced organic matter and humic substances.
Long-term management solutions require a comprehensive understanding of the physiological context of crops [39]. Our research revealed that the combined application increased the chlorophyll a content of crops, possibly improving photosynthetic activity and, thus, crop growth. Moreover, the ABA content was substantially reduced by combination therapy. In general, ABA elevation above a specific threshold level can cause severe plant stress, which can be harmful [40]. The ABA level was significantly increased by using only food waste. This could be attributed to an increase in Na inflow. However, the ABA concentration was reduced considerably after inoculation with Chlorella. Excessive salt prevents critical nutrients from being absorbed, and PGPM improves the compartmentalization and exclusion of harmful ions by absorbing and transferring soil nutrients. Therefore, understanding the interaction of Na+/K+ and Ca++ ions is crucial for understanding plant physiology [41,42]. Similarly, ABA has been linked to the regulation of stomatal conductance in plant cells for water balance, signaling, and osmotic adjustment [43]. Our results are supported by numerous studies [44–46] reporting that the accumulation of Na+ ions would elevate ABA levels. Similarly, our findings corroborate those of Arkhipova et al. [47] and Mohamed et al. [48], who reported a high efflux rate of Na+ ions and low ABA levels upon inoculation with plant growth-promoting microorganisms, consequently improving plant growth.
The interactions among antioxidant activity, ionic balance, and phytohormones are critical to improving plant metabolomics. Under diverse environmental conditions, plants activate their intrinsic antioxidant defense system to help with ROS detoxification [49,50]. DPPH, SOD, flavonoid, and phenolic content are considered vital components that regulate the antioxidant activities of crops. Typically, superoxide dismutase converts O2 to H2O2, which catalyzes the conversion of 2H2O2 to O2 + 2H2O [51]. DPPH scavenges toxic radicals, flavonoids, and phenolic compounds known as metal chelators and scavenges H2O2. In addition, flavonoids also act as signaling molecules in plant-microbe interactions [52]. The present experimental study suggested that the exogenous application of food waste and Chlorella mostly significantly enhance the phenolic content and DPPH, SOD, and flavonoid levels. These antioxidants are thought to neutralize vulnerable oxygen radicals, known as ROS. Moreover, our results also suggested that the combined application of FW2/CR plants exhibited lower electrolytic leakage compared to sole FW2 and FW4, which validates the possible low levels of ROS in these plants. Collectively, the lower content of these scavenging radicals and electrolytic leakage indicates the potential growth and development of red pepper seedlings. Overall, the co-inoculation of CR and FW enhanced the morphological features of the plant by maintaining the soil pH and EC, improving the chlorophyll a content, and maintaining the ABA regulation at a steady level, along with stabilizing the antioxidant potential and nutrient uptake. Through extensive research, this combination can be used to combat diverse types of environmental stress in the future.
Previously, our team also determined the effect of food waste on the growth of Chinese cabbage [5] and the mitigation of commercial food waste-related salinity stress with the help of halotolerant isolate Bacillus pumilus MAK9 [21]. The present study validated the effect of food waste on the growth of red pepper via the activation of the antioxidant defense system and regulation of endogenous abscisic acid.
In this study, red pepper was treated with different concentrations of food waste along with the plant growth-promoting agent Chlorella. An increase in the morphological growth parameters was observed. The study demonstrated that physio-morphological features improved owing to the activation of endogenous ABA and the antioxidant defense system.
Funding Statement: This work was supported by the National Research Foundation of Korea (NRF) Grant Funded by the Korean Government (MSIT) (No. 2022R1A2C1008993).
Conflicts of Interest: The authors declare that they have no conflicts of interest to report regarding the present study.
References
1. Khan, M. A., Kalsoom, Imran, M., Lubna, Shaffique, S. et al. (2022). Mitigation of commercial food waste-related salinity stress using halotolerant rhizobacteria in Chinese cabbage plants. Horticulturae, 8(49). https://doi.org/10.3390/horticulturae8010049 [Google Scholar] [CrossRef]
2. Melikoglu, M., Lin, C. S. K., Webb, C. (2013). Analysing global food waste problem: Pinpointing the facts and estimating the energy content. Central European Journal of Engineering, 3, 157–164. [Google Scholar]
3. Scherhaufer, S., Moates, G., Hartikainen, H., Waldron, K., Obersteiner, G. (2018). Environmental impacts of food waste in Europe. Waste Management, 77, 98–113. [Google Scholar] [PubMed]
4. Gustavsson, J., Cederberg, C., Sonesson, U., Van Otterdijk, R., Meybeck, A. (2011). Global food losses and food waste. pp. 1–24. Food and Agriculture Organization of the UN, Rome, Italy. http://www.fao.org/docrep/014/mb060e/mb060e00.pdf [Google Scholar]
5. Kang, S. M., Shaffique, S., Kim, L. R., Kwon, E. H., Kim, S. H. et al. (2021). Effects of organic fertilizer mixed with food waste dry powder on the growth of Chinese cabbage seedlings. Environments, 8(8), 86. https://doi.org/10.3390/environments8080086 [Google Scholar] [CrossRef]
6. Paritosh, K., Kushwaha, S. K., Yadav, M., Pareek, N., Chawade, A. et al. (2017). Food waste to energy: An overview of sustainable approaches for food waste management and nutrient recycling. BioMed Research International, 1–19. [Google Scholar]
7. Pergola, M., Persiani, A., Palese, A. M., di Meo, V., Pastore, V. et al. (2018). Composting: The way for a sustainable agriculture. Applied Soil Ecology, 123, 744–750. [Google Scholar]
8. Chatterjee, R., Gajjela, S., Thirumdasu, R. (2017). Recycling of organic wastes for sustainable soil health and crop growth. International Journal of Waste Resources, 7, 296–292. [Google Scholar]
9. Ju, M., Bae, S. J., Kim, J. Y., Lee, D. H. (2016). Solid recovery rate of food waste recycling in South Korea. Journal of Material Cycles and Waste Management, 18, 419–426. [Google Scholar]
10. Mukhopadhyay, R., Sarkar, B., Jat, H. S., Sharma, P. C., Bolan, N. S. (2021). Soil salinity under climate change: Challenges for sustainable agriculture and food security. Journal of Environmental Management, 280, 111736. [Google Scholar] [PubMed]
11. O'Connor, J., Hoang, S. A., Bradney, L., Dutta, S., Xiong, X. et al. (2021). A review on the valorisation of food waste as a nutrient source and soil amendment. Environmental Pollution, 272, 115985. [Google Scholar] [PubMed]
12. Chew, K. W., Chia, S. R., Yen, H. W., Nomanbhay, S., Ho, Y. C. et al. (2019). Transformation of biomass waste into sustainable organic fertilizers. Sustainability, 11, 2266. [Google Scholar]
13. Diacono, M., Montemurro, F. (2015). Effectiveness of organic wastes as fertilizers and amendments in salt-affected soils. Agriculture, 5, 221–230. [Google Scholar]
14. Kim, M. J., Shim, C. K., Ko, B. G., Kim, J. (2020). Effect of the microalga Chlorella fusca CHK0059 on strawberry PGPR and Biological control of fusarium wilt disease in non-pesticide hydroponic strawberry cultivation. Journal of Microbiology and Biotechnology, 30(5), 708–716. [Google Scholar] [PubMed]
15. Arroussi, H. E., Benhima, R., Elbaouchi, A., Sijilmassi, B., Mernissi, N. E. et al. (2018). Dunaliella salina exopolysaccharides: A promising biostimulant for salt stress tolerance in tomato (Solanum lycopersicum). Journal of Applied Phycology, 30, 2929–2941. [Google Scholar]
16. Chiaiese, P., Corrado, G., Colla, G., Kyriacou, M. C., Rouphael, Y. (2018). Renewable sources of plant biostimulation: Microalgae as a sustainable means to improve crop performance. Frontiers in Plant Science, 9, 1782. [Google Scholar] [PubMed]
17. Adhikari, A., Khan, M. A., Imran, M., Lee, K. E., Kang, S. M. et al. (2022). The combined inoculation of curvularia lunata AR11 and biochar stimulates synthetic silicon and potassium phosphate use efficiency, and mitigates salt and drought stresses in rice. Frontiers in Plant Science, 13. https://doi.org/10.3389/fpls.2022.816858 [Google Scholar] [PubMed] [CrossRef]
18. Devi, P. I., Manjula, M., Bhavani, R. (2022). Agrochemicals, environment, and human health. Annual Review of Environment and Resources, 47, 399–421. [Google Scholar]
19. Majeed, A. (2018). Application of agrochemicals in agriculture: Benefits, risks and responsibility of stakeholders. Journal of Food Science and Toxicology, 2(1:3). [Google Scholar]
20. Rahman, K., Debnath, S. C. (2015). Agrochemical use, environmental and health hazards in Bangladesh. International Research Journal of Interdisciplinary and Multidisciplinary Studies, 1, 75–79. [Google Scholar]
21. Khan, M. A., Imran, M., Shaffique, S., Kwon, E. H., Kang, S. M. et al. (2022). Mitigation of commercial food waste-related salinity stress using halotolerant rhizobacteria in Chinese cabbage plants. Horticulturae, 8, 49. [Google Scholar]
22. Muche, M., Kokeb, A., Molla, E. (2015). Assessing the physicochemical properties of soil under different land use types. Journal of Environmental & Analytical Toxicology, 5, 1. [Google Scholar]
23. Rabbani, M. A., Maruyama, K., Abe, H., Khan, M. A., Katsura, K. et al. (2003). Monitoring expression profiles of rice genes under cold, drought, and high-salinity stresses and abscisic acid application using cDNA microarray and RNA gel-blot analyses. Plant Physiology, 133, 1755–1767. [Google Scholar] [PubMed]
24. Adhikari, A., Khan, M. A., Lee, K. E., Kang, S. M. et al. (2020). The halotolerant rhizobacterium-pseudomonas koreensis MU2 enhances inorganic silicon and phosphorus use efficiency and augments salt stress tolerance in soybean (Glycine max L.). Microorganisms, 8. https://doi.org/10.3390/microorganisms8091256 [Google Scholar] [PubMed] [CrossRef]
25. Adhikari, B., Dhungana, S. K., Ali, M. W., Adhikari, A., Kim, I. D. et al. (2018). Resveratrol, total phenolic and flavonoid contents, and antioxidant potential of seeds and sprouts of Korean peanuts. Food Science and Biotechnology, 27, 1275–1284. https://doi.org/10.1007/s10068-018-0364-7 [Google Scholar] [PubMed] [CrossRef]
26. Blois, M. S. (1958). Antioxidant determinations by the use of a stable free radical. Nature, 181, 1199–1200. [Google Scholar]
27. Kang, S. M., Khan, A. L., Waqas, M., You, Y. H., Kim, J. H. et al. (2014). Plant growth-promoting rhizobacteria reduce adverse effects of salinity and osmotic stress by regulating phytohormones and antioxidants in Cucumis sativus. Journal of Plant Interactions, 9, 673–682. [Google Scholar]
28. Parizeau, K., von Massow, M., Martin, R. J. W. M. (2015). Household-level dynamics of food waste production and related beliefs, attitudes, and behaviours in Guelph, Ontario. Waste Management, 35, 207–217. [Google Scholar] [PubMed]
29. Bhatia, L., Harit, J., Tanushree, S., Prakash, K. S. (2023). Food waste utilization for reducing Carbon footprints towards sustainable and cleaner environment: A review. International Journal of Environmental Research and Public Health, 2(3), 2318. [Google Scholar]
30. Papargyropoulou, E., Lozano, R., Steinberger, J. K., Wright, N., bin Ujang, Z. (2014). The food waste hierarchy as a framework for the management of food surplus and food waste. Journal of Cleaner Production, 76, 106–115. [Google Scholar]
31. Ma, Y., Liu, Y. (2019). Turning food waste to energy and resources towards a great environmental and economic sustainability: An innovative integrated biological approach. Biotechnology Advances, 37(7), 107414. [Google Scholar] [PubMed]
32. Lam, M. K., Lee, K. T. J. A. e. (2012). Potential of using organic fertilizer to cultivate Chlorella vulgaris for biodiesel production. Applied Energy, 94, 303–308. [Google Scholar]
33. Hu, B., Min, M., Zhou, W., Du, Z., Mohr, M. et al. (2012). Enhanced mixotrophic growth of microalga Chlorella sp. on pretreated swine manure for simultaneous biofuel feedstock production and nutrient removal. Bioresource Technology, 126, 71–79. [Google Scholar] [PubMed]
34. Li, R., Duan, N., Zhang, Y., Liu, Z., Li, B. et al. (2017). Anaerobic co-digestion of chicken manure and microalgae Chlorella sp.: Methane potential, microbial diversity and synergistic impact evaluation. Waste Management, 68, 120–127. [Google Scholar] [PubMed]
35. Chew, K. W., Chia, S. R., Show, P. L., Ling, T. C., Arya, S. S. et al. (2018). Food waste compost as an organic nutrient source for the cultivation of Chlorella vulgaris. Bioresource Technology, 267, 356–362. https://doi.org/10.1016/j.biortech.2018.07.069 [Google Scholar] [PubMed] [CrossRef]
36. Westerman, P., Bicudo, J. (2005). Management considerations for organic waste use in agriculture. Bioresource Technology, 96, 215–221. [Google Scholar] [PubMed]
37. Lee, J. H., Kang, Y. G., Luyima, D., Park, S. J., Oh, T. K. et al. (2020). Characteristics of food waste: Water and salinity contents. Korean Journal of Agricultural Science, 47, 375–380. [Google Scholar]
38. Zhou, Y., Selvam, A., Wong, J. W. (2016). Effect of Chinese medicinal herbal residues on microbial community succession and anti-pathogenic properties during co-composting with food waste. Bioresource Technology, 217, 190–199. [Google Scholar] [PubMed]
39. Dawood, M. F. A., Zaid, A., Latef, A. A. H. A. (2021). Salicylic acid spraying-induced resilience strategies against the damaging impacts of drought and/or salinity stress in two varieties of Vicia faba L. seedlings. Journal of Plant Growth Regulation, 41(5), 1919–1942. https://doi.org/10.1007/s00344-021-10381-8 [Google Scholar] [CrossRef]
40. González-Guzmán, M., Gómez-Cadenas, A., Arbona, V. (2021). Abscisic acid as an emerging modulator of the responses of plants to low oxygen conditions. Frontiers in Plant Science, 12. https://doi.org/10.3389/fpls.2021.661789 [Google Scholar] [PubMed] [CrossRef]
41. Adhikari, A., Khan, M. A., Lee, K. E., Kang, S. M., Dhungana, S. K. et al. (2020). The halotolerant rhizobacterium—Pseudomonas koreensis MU2 enhances inorganic silicon and phosphorus use efficiency and augments salt stress tolerance in soybean (Glycine max L.). Microorganisms, 8(9), 1256. [Google Scholar] [PubMed]
42. Thor, K. J. F. I. P. S. (2019). Calcium—nutrient and messenger. Frontiers in Plant Science, 10, 440. [Google Scholar] [PubMed]
43. Harb, A., Krishnan, A., Ambavaram, M. M., Pereira, A. (2010). Molecular and physiological analysis of drought stress in Arabidopsis reveals early responses leading to acclimation in plant growth. Plant Physiol, 154, 1254–1271. https://doi.org/10.1104/pp.110.161752 [Google Scholar] [PubMed] [CrossRef]
44. Zhu, C., Schraut, D., Hartung, W., Schäffner, A. R. (2005). Differential responses of maize MIP genes to salt stress and ABA. Journal of Experimental Botany, 56(421), 2971–2981. [Google Scholar] [PubMed]
45. Chen, S., Li, J., Wang, S., Hüttermann, A., Altman, A. J. T. (2001). Salt, nutrient uptake and transport, and ABA of populus euphratica, a hybrid in response to increasing soil NaCl. Trees, 15, 186–194. [Google Scholar]
46. Janicka-Russak, M., Kłobus, G. J. J. O. P. P. (2007). Modification of plasma membrane and vacuolar H+-ATPases in response to NaCl and ABA. Journal of Plant Physiology, 164(3), 295–302. [Google Scholar] [PubMed]
47. Arkhipova, T., Martynenko, E., Sharipova, G., Kuzmina, L., Ivanov, I. et al. (2020). Effects of plant growth promoting rhizobacteria on the content of abscisic acid and salt resistance of wheat plants. Plants, 9(11), 1429. [Google Scholar] [PubMed]
48. Mohamed, H., Gomaa, E. J. P. (2012). Effect of plant growth promoting Bacillus subtilis and Pseudomonas fluorescens on growth and pigment composition of radish plants (Raphanus sativus) under NaCl stress. Photosynthetica, 50, 263–272. [Google Scholar]
49. Hasanuzzaman, M., Bhuyan, M. H. M. B., Zulfiqar, F., Raza, A., Mohsin, S. M. et al. (2020). Reactive oxygen species and antioxidant defense in plants under abiotic stress: Revisiting the crucial role of a universal defense regulator. Antioxidants, 9(8), 681. [Google Scholar] [PubMed]
50. Hasanuzzaman, M., Bhuyan, M. H. M. B., Parvin, K., Bhuiyan, T. F., Anee, T. I. et al. (2020). Regulation of ROS metabolism in plants under environmental stress: A review of recent experimental evidence. International Journal of Molecular Sciences, 21(22), 8695. https://doi.org/10.3390/ijms21228695 [Google Scholar] [PubMed] [CrossRef]
51. You, J., Chan, Z. (2015). ROS regulation during abiotic stress responses in crop plants. Frontiers in Plant Science, 6, 1092. [Google Scholar] [PubMed]
52. Bautista, I., Boscaiu, M., Lidón, A., Llinares, J. V., Lull, C. et al. (2016). Environmentally induced changes in antioxidant phenolic compounds levels in wild plants. Acta Physiologiae Plantarum, 38, 1–15. [Google Scholar]
Cite This Article
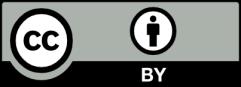