Open Access
ARTICLE
The Effect of Soil Enzymes and Polysaccharides Secreted by the Roots of Salvia miltiorrhiza Bunge under Drought, High Temperature, and Nitrogen and Phosphorus Deficits
1 State Key Laboratory of Characteristic Chinese Medicine Resources in Southwest China, Chengdu University of Traditional Chinese Medicine, Chengdu, China
2 School of Pharmacy, Chengdu University of Traditional Chinese Medicine, Chengdu, China
3 School of Medical Technology, Chengdu University of Chinese Medicine, Chengdu, China
* Corresponding Author: Zhuyun Yan. Email:
(This article belongs to the Special Issue: Abiotic and Biotic Stress Tolerance in Crop)
Phyton-International Journal of Experimental Botany 2024, 93(1), 119-135. https://doi.org/10.32604/phyton.2023.046075
Received 18 September 2023; Accepted 22 November 2023; Issue published 26 January 2024
Abstract
Root exudates serve as crucial mediators for information exchange between plants and soil, and are an important evolutionary mechanism for plants’ adaptation to environmental changes. In this study, 15 different abiotic stress models were established using various stress factors, including drought (D), high temperature (T), nitrogen deficiency (N), phosphorus deficiency (P), and their combinations. We investigated their effects on the seedling growth of Salvia miltiorrhiza Bunge and the activities of Solid-Urease (S-UE), Solid-Nitrite Reductase (S-NiR), Solid-Nitrate Reductase (S-NR), Solid-Phosphotransferase (S-PT), and Solid-Catalase (S-CAT), as well as the contents of polysaccharides in the culture medium. The results showed that the growth of S. miltiorrhiza was inhibited under 15 stress conditions. Among them, 13 stress conditions increased the root-shoot ratio. These 15 stress conditions significantly reduced the activity of S-NR, two combinations significantly improved the activity of S-NIR, they were synergistic stresses of high temperature and nitrogen deficiency (TN), and synergistic stresses of drought and nitrogen deficiency (DN) (p < 0.05). The activity of S-UE was significantly improved under N, D, T, synergistic stresses of drought and high temperature (DT), DN, synergistic stresses of drought and phosphorus deficiency (DP), and synergistic stresses of high temperature, nitrogen, and phosphorus deficiency (TNP) stress conditions (p < 0.05). Most stress combinations reduced the activity of S-PT, but D and T significantly improved it. (p < 0.05). The N, DN, and TN stress conditions significantly reduced S-CAT activity. The P, DT, and synergistic stresses of drought, high temperature, and phosphorus deficiency (DTP) significantly decreased the total polysaccharide content of the soil (p < 0.05). The research suggested that abiotic stress hindered the growth of S. miltiorrhiza and altered the behavior of root secretion. Roots regulated the secretion of several substances in response to various abiotic stresses, including soil nitrogen cycle enzymes, phosphorus transport-related enzymes, and antioxidant enzymes. In conclusion, plants regulate the utilization of rhizosphere substances in response to abiotic stresses by modulating the exudation of soil enzymes and polysaccharides by the root system. At the same time, soil carbon sequestration was affected by the adverse environment, which restricted the input of organic matter into the soil.Keywords
Supplementary Material
Supplementary Material FileRoot exudates are a complex mixture of biochemical compounds that are actively or passively secreted by plant roots. These compounds comprise diverse small molecules, such as amino acids and organic acids, and macromolecules, including polysaccharides, proteins, and biologically active enzymes [1]. Biotic and abiotic stress conditions alter the composition and quantity of plant root exudates, thereby altering the rhizosphere ecosystem and assisting plants in adapting to different stresses [1,2]. During the process of plant growth, development, and reproduction, approximately 21% of the net photosynthetic products of plants enter the soil as root exudates to adapt to different stress environments [3–5]. Moreover, root exudates serve as a source of nutrients and energy to the rhizosphere microbiome, regulating the structure of soil microbial communities, affecting enzyme activities produced by microorganisms, and ultimately influencing the decomposition, mineralization, and availability of organic compounds and soil nutrients [6]. Root secretory behavior is highly sensitive to abiotic stress and has a significant impact on plant yield and quality. Previous research has focused on maize [7] and other plants [8]. Consequently, root exudates influence soil enzymes and are essential in the soil material cycle, energy conversion, and plant carbon storage [4]. Especially, it plays an important role in the soil nitrogen and phosphorus cycle [9]. Meanwhile, soil enzymes are important components of root exudates.
The enzymes secreted by the roots are a mixture of plants and microorganisms. Root secretion is not only an important source of soil enzymes but also the main factor driving microorganisms to secrete soil enzymes. Solid-Nitrate Reductase (S-NR), Solid-Nitrite Reductase (S-NiR), and Solid-Urease (S-UE) are important enzymes in the nitrogen cycle of soil. Specifically, S-NR catalyzes the reduction of nitrate to nitrite, S-NiR catalyzes the reduction of nitrite to nitric oxide [10], and S-UE hydrolyzes urea to produce ammonia and carbonic acid [11]. The Solid-Phosphotransferase (S-PT) plays a crucial role in phosphorus uptake and utilization by catalyzing the transfer of phosphate groups from donors to acceptors [12]. Solid-Catalase (S-CAT) prevents the accumulation of toxic substances by promoting the degradation of hydrogen peroxide [13]. Additionally, root-secreted polysaccharides can enhance the growth of polysaccharide-utilizing microbial communities and stimulate the production of extracellular enzymes, thereby facilitating the decomposition of soil organic matter and nutrient cycling [14]. Furthermore, these polysaccharides also serve as a significant source of organic matter in the soil [15]. Abiotic stresses such as drought [16] and nitrogen deficiency [17] can change soil enzyme activities, thereby affecting soil-plant interactions. Nevertheless, root secretion plays an important role in the plant response to environmental stress, especially soil enzymes secreted by roots and soil enzymes secreted by microorganisms driven by root exudates. However, reports on soil enzymes and polysaccharides in root exudates under sterile conditions are limited.
Drought and high temperatures resulting from climate change [18], combined with the scarcity of nitrogen and phosphorus in ecologically cultivated areas for Chinese traditional medicine, pose significant constraints on plant growth and development [19]. Salvia miltiorrhiza, a commonly employed model medicinal plant, holds significant importance in the treatment of cardiovascular-related diseases [20]. It grows in a complex environment and is exposed to a wide range of abiotic stresses. These stresses have a substantial influence on the yield and quality of the plant [21,22]. Most recent studies have concentrated on how plants respond to individual stresses. However, nature is primarily exposed to multiple stresses, and it is not known whether this is more severe than experiencing a single stress. Meanwhile, there is no direct evidence that the root system secretes soil enzymes and polysaccharides into the soil during plant responses to adverse conditions. Based on this understanding, a total of 15 stress models were designed under sterile conditions. These models included high temperature (T), drought (D), nitrogen deficiency (N), phosphorus deficiency (P), and their combinations. After the stress culture of S. miltiorrhiza test-tube seedlings, the secretion of S-UE, S-NIR, S-NR, S-PT, and S-CAT activities and total polysaccharide content changes were measured. Understanding how S. miltiorrhiza alters its root secretions can provide insights into its adaptive mechanisms and its influence on soil nutrient dynamics. Further research in this area can shed light on the intricate interactions between S. miltiorrhiza, abiotic stress, and the soil ecosystem.
2.1 Study Site and Test Materials
The plant materials were obtained from the Medicinal Botanical Garden of Chengdu University of Traditional Chinese Medicine (E: 103.81°, N: 30.68°), and the plant was identified as S. miltiorrhiza Bunge. cv. Sativa by Professor Zhu-Yun Yan (Chengdu University of Traditional Chinese Medicine, College of Pharmacy). These leaves of S. miltiorrhiza were then cultivated into tissue culture seedlings after undergoing surface sterilization, tissue healing, bud clustering, and root induction in the laboratory. The medium for test-tube seedlings was prepared as follows: callus induction medium was MS + 2.0 mg∙L−1 6-BA + 1.0 mg∙L−1 NAA + 30 g sucrose; differentiation medium was MS + 1.0 mg∙L−1 6-BA + 0.1 mg∙L−1 NAA + 30 g sucrose; rooting medium was 1/2 MS + 0.2 mg∙L−1 NAA + 0.5 mg∙L−1 IBA + 15 g sucrose. A rooting rate of 94% was achieved.
The culture medium utilized was shell shale sourced from Ximeishan Village (E: 104.54°, N: 30.94°), Shiquan Township, Zhongjiang County, Sichuan Province. The soil was air-dried, ground in a mortar, and sieved to remove impurities (1–5 mm). Dry heat sterilization was performed at 180°C for 4 h, and the cycle was repeated twice to remove microorganisms and their secretions. After cooling, it was packed in kraft paper and then sterilized again through dry heat at 120°C for 3–4 h before use. Sterilization of soil samples is a common practice in scientific research to prevent the introduction of microorganisms into the experimental setup. The pH value of the shell shale was 7.78, with an organic matter content of 0.69 g/kg, alkaline nitrogen of 4.2 mg/kg, potassium of 3.79 mg/kg, and phosphorus of 2.23 mg/kg.
The culture device used was a circular polypropylene box with a top diameter of 17.2 cm, a bottom diameter of 11.8 cm, and a height of 9.7 cm. Each box was wrapped in kraft paper and sterilized at high-pressure steam at 0.1 MPa and 121°C for 30 min, and after cooling, it was filled with 600 g of culture medium. We made sure that the experimental materials and the experimental environment were in aseptic conditions, as the growth of S. miltiorrhiza was only affected by stress.
Four stress factors, including T, D, N, and P, were measured at 2 levels per factor for 16 treatments, of which CK was the control group. The treatment conditions for each group are shown in Table 1. As a full nutrient solution, Hoagland’s solution was used (Supplementary Table S1). In the reference method [23,24], the phosphorus content was set to 0 mM for the phosphorus stress treatment, and the nitrogen content was set to 0 mM for the nitrogen deficiency stress treatment. The corresponding concentrations of KH2PO4, Ca(NO3)2, KNO3, and NH4NO3 were adjusted to 0%, respectively. The lack of Ca and K in the deficit solutions were supplemented by CaCl2 and KCl, respectively. At the same time, the remaining components of Hoagland’s solution remained unchanged. Based on ground temperature data from the S. miltiorrhiza cultivation base in Zhongjiang, Sichuan, the temperature was chosen with 25°C/20°C (day/night) for the normal conditions and 42°C/35°C (day/night) for the high temperature stress condition. The water holding capacity (WHC) was 75% for normal moisture conditions and 30% for drought stress conditions [25].
Under aseptic conditions, test tube seedlings with 3–5 uniform long roots weighing approximately 0.5 g were carefully extracted from the culture flasks, and any adhering agar was eliminated using sterile filter paper. The seedlings were weighed and transferred to culture boxes containing 600 g of shale pellets, 2 plants per box. A total of 96 boxes were planted. Each box was filled with 100 mL of sterile water and 5 mL of sterilized Hoagland’s total nutrient solution at the seedlings’ roots. Each box’s total weight was recorded as a reference value for irrigation. Place on the culture rack in the tissue culture room for cultivation at 25°C/20°C (day/night) and a light intensity of 3000 lx. The water was weighed and replaced every 3 days to maintain 75% WHC of the culture medium and the roots of the seedlings were watered with 5 mL of Hoagland’s full nutrient solution each week. After 4 weeks of incubation, the plants were randomly divided into 16 groups of 6 boxes each and watered with the nutrient solution needed for the different stress treatment groups. After 3 weeks of culture, the morphological characteristics of the plant’s nitrogen and phosphorus deficiency stress and then other synergistic stress treatments were observed. The natural precipitation method was used for drought treatment in the drought synergistic stress treatment group. After the WHC dropped to 30%, the 30% WHC was maintained until the end of the treatment. The plants were transferred to an incubator at 42°C/35°C (day/night) under the same light conditions for the temperature stress treatment. All experiments were completed after 5 days of heat stress.
2.4 Sample Collection and Determination
At the end of the culture experiments, the roots were washed with sterile water and blotted dry with absorbent paper, and the weights of the plants’ above- and below-ground parts were measured separately. Two boxes of culture substrates in each group were randomly combined into one sample, mixed, and sampled by quartering. The samples were passed through a 40-mesh sieve after grinding to determine soil enzymes and polysaccharides. Enzymatic activities of S-UE, S-NiR, S-NR, and S-CAT were evaluated using cominbio kits (Suzhou Keming Biotechnology Co., Ltd., China) following the manufacturer’s guidelines, while mlbio kits (Shanghai Enzyme Biotechnology Co., Ltd., China) were employed to measure the activity of S-PT enzymes. Pentoses were determined using the lichenol-hydrochloric acid method [26], and hexoses were determined using the anthrone-sulphuric acid method [27]. The sum of the two was then calculated as the total polysaccharide content.
S-NiR activity was assessed using the cominbio kit’s method. The amount of reducing 1 μmol NO2− per g soil sample per day was regarded as an enzyme activity unit. 0.02 g of air-dried soil sample was mixed with 40 µL each of sodium nitrite and glucose solutions. The mixture was thoroughly combined and reacted for one hour at 25°C. Then, 40 μL of aluminum potassium alum solution was added and centrifuged with sufficient shaking. Take 70 μL of supernatant, along with 1:1 p-amino benzene sulfonic acid-phosphoric acid solution and 140 µL of N-1-naphthalene ethylenediamine hydrochloride solution. The components were mixed well and evaluated for absorbance at 540 nm. Control tubes were supplied with distilled water instead of sodium nitrite solution, while blank tubes had no samples.
S-NR activity was assessed using the cominbio kit’s method. The amount of 1 μmol NO2− produced per gram of soil sample per day was regarded as one S-NR activity unit. 0.02 g of air-dried soil sample was mixed with 225 µL of KNO3 solution and 75 µL of Reduced Coenzyme I (NADH) solution. The samples were well mixed in a 37°C water bath for 24 h. After centrifugation, 130 μL of the supernatant was taken and added to 85 µL each of p-amino benzene sulfonic acid solution and α-naphthylamine solution. Mix thoroughly to develop the color for 20 min, then spin 200 μL of the supernatant and read the absorbance at 540 nm. Distilled water replaced the KNO3 solution for the control tube, while the blank tubes omitted soil samples. The standard tube replaced the soil sample with 0.1 μmol/mL NaNO2 solution.
S-UE activity was assessed using the cominbio kit’s method. 1 μg of NH3-N produced per gram of soil sample per day was defined as an enzyme activity unit. 0.02 g of air-dried soil sample was collected after adding 20 µL of toluene and shaking. Afterward, 90 µL of urea and 190 µL of citric acid-potassium hydroxide solution were blended before a 24 h water bath at 37°C. Centrifuge 80 μL of the supernatant after 10-fold dilution, and the mixture was coupled with 15 µL of phenol, methanol, acetone, absolute ethanol, and NaOH solutions. Next, 15 µL of sodium hypochlorite solution was added and mixed well before being placed at room temperature for 20 min. The absorbance of the resultant mixture was monitored at 578 nm after the inclusion of 90 µL of distilled water. Distilled water substituted urea in the control tube.
S-CAT activity was assessed using the cominbio kit’s method. The degradation of 1 μmol H2O2 catalyzed per g of air-dried soil sample per day was defined as an enzyme activity unit. Air-dried soil samples (0.03 g) were mixed with 260 μL of hydrogen peroxide. After 20 min, 10 μL of aluminium-potassium alum was added and mixed before spinning. 180 µL of the supernatant was collected and mixed with 20 µL of sulfuric acid solution before monitoring absorptivity at 240 nm. The control tube contained distilled water instead of hydrogen peroxide, while the blank tubes contained no soil samples.
S-PT activity was assessed using the mlbio kit’s method. 0.1 g of soil was mixed with 0.9 mL of Phosphate buffer saline (PBS) before centrifugation at 4000 r for 15 min. The resultant supernatant became the sample for examination. Next, 50 µL of the diluted standard and 40 µL of the sample diluent were added to the sample, which was then sealed with a sealing film and incubated at 37°C for 30 min. Afterward, the liquid was discarded, followed by the introduction of wash solution, left for 30 s, and discarded five times. Subsequently, 50 µL of HRP enzyme-labeled reagent was included, and incubation and washing processes resumed before adding 50 µL of carbamide peroxide color reagent and 50 µL of color reagent. At this stage, color development was initiated, with 10 min allotted for completion in the dark at 37°C. Additionally, 50 µL of termination solution was infused before absorbance levels were measured at 450 nm. Blank wells that omitted samples and enzyme labeling reagents were also featured. Phosphotransferase markers (Supplementary Fig. S1a).
The content determination method of the total polysaccharide in the soil followed the method of Pansu et al. [28]. Preparation of the test solution: 2.5 g of air-dried soil, add 20 mL of 2.5 M H2SO4, heat, and reflux in a water bath at 100°C for 20 min. Cool, filter, and collect the filtrate. Put the residue, add 1 mL 13 M H2SO4, and place it at room temperature for 16 h. Slowly add 25 mL of distilled water, let cool, heat at 100°C for 5 h after airtight. Cool, filter, and combine the filtrate with the above filtrate, and dilute to 50 mL to obtain the test solution. The total polysaccharide content was determined using the lichenol-hydrochloric acid method for pentose, while hexose was assessed using the anthrone-sulfuric acid technique. Pentose and hexose markers, respectively (Supplementary Figs. S1b, S1c). Finally, the sum of the two was calculated as the polysaccharide content.
The data were analyzed using IBM SPSS 21.0 software for one-way analysis of variance, and the least significant difference method and Duncan’s were used for multiple comparisons. Data presented here as a fully randomized design with three replicates expressed as the mean ± standard deviation. Different lowercase letters in the table indicate a significant difference from the blank. They were graphed with GraphPad Prism 9 and Spearman correlation analysis with R (version 4.1.2).
3.1 Changes in Growing S. miltiorrhiza Seedlings
Abiotic stress negatively impacts the growth of S. miltiorrhiza seedlings, among which P, synergistic stresses of drought and nitrogen deficiency (DN), synergistic stresses of drought, nitrogen deficiency and phosphorus deficiency (DNP) and synergistic stresses of drought, high temperature, nitrogen deficiency, and phosphorus deficiency (DTNP) stresses reached a significant level (p < 0.05). P, DN, synergistic stresses of drought, high temperature and nitrogen deficiency (DTN), synergistic stresses of drought high temperature and phosphorus deficiency (DTP), DNP, synergistic stresses of high temperature, nitrogen deficiency and phosphorus deficiency (TNP), and DTNP stresses significantly inhibited the fresh weight of aboveground parts of S. miltiorrhiza (p < 0.05) (Fig. 1, Supplementary Table S2). Drought or synergistic stresses of drought and high temperature increased the plant growth inhibition of nitrogen deficiency, but not significantly (e.g., DN and DTN stresses) (Fig. 1a). Drought or high temperature or synergistic stresses of drought and high temperatures alleviated the plant growth inhibition of phosphorus deficiency, but not significantly (e.g., DP, TP, and DTP stresses) (Fig. 1b). Synergistic stresses of drought and high temperatures aggravated the plant growth inhibition of nitrogen and phosphorus deficiency (e.g., DTNP stresses) (p < 0.05) (Fig. 1c). The synergistic stresses of drought and high temperatures exacerbated the effects of single-factor inhibition, but not to a significant extent (e.g., DT stress) (Fig. 1d).
Figure 1: The growth changes of S. miltiorrhiza seedlings under abiotic stress. (a), (b), and (c) respectively represent the changes in the growth effects of the superposition of various environmental factors under the stress of nitrogen, phosphorus, and nitrogen and phosphorus deficiency, and (d) represents the changes in the growth effects of the superposition of drought and high temperature. * indicates significant difference from CK treatments or significant difference between the two groups, p < 0.05
3.2 The Activity of Enzymes Related to Nitrogen Metabolism in the Medium
Abiotic stress significantly reduced S-NR activity in the culture medium, with single factors N and T stresses having the most potent inhibitory effect. In contrast, the combined effect was most severe in synergistic stresses of high temperature and nitrogen deficiency (TN), synergistic stresses of nitrogen and phosphorus deficiency (NP), and DTN stresses (p < 0.05). DN and TN stress improved S-NiR activity (p < 0.05), but the other factors did not show statistical significance. Moreover, N, T, D, synergistic stresses of high temperature and drought (DT), DN, synergistic stresses of drought and phosphorus deficiency (DP), and TNP stresses all elevated S-UE activity in the culture medium, while synergistic stresses of high temperature and phosphorus deficiency (TP), TN, NP, and DTN stresses significantly reduced S-UE activity in the culture medium (p < 0.05) (Fig. 2, Supplementary Table S3). These findings indicate that under abiotic stress, plants reduce the secretion of the S-NR enzyme from their root system, which curtails denitrification and prevents nitrate-nitrogen loss. In response to N, T, D, DT, DN, DP, and TNP stresses, plants secrete S-UE to enhance organic nitrogen utilization.
Figure 2: Roots in the culture medium secrete the activity of enzymes related to nitrogen metabolism. (a), (b) and (c) respectively represent the changes in nitrogen metabolism-related enzymes under the superimposed environmental factors of nitrogen, phosphorus, and nitrogen-phosphorus deficit stresses. (d) Indicates the changes in nitrogen metabolism-related enzymes under the superimposed drought and high temperature. * indicates significant difference from CK treatments or significant difference between the two groups, p < 0.05
Drought or high temperature stress significantly improved the effect of nitrogen deficiency on the release of S-NiR from roots (e.g., DN and TN stresses) (p < 0.05). Drought stress significantly decreases the effect of nitrogen deficiency on the release of S-NR from roots and simultaneously significantly promotes the release of S-UE from roots (e.g., DN stress) (p < 0.05). High temperature or synergistic stresses of drought and high temperature inhibit the effect of nitrogen deficit on the induction of S-UE release from roots (e.g., TN and DTN stresses) (p < 0.05) (Fig. 2a). Moreover, drought stress significantly enhances the effect of phosphorus deficiency on the release of S-UE from roots, while high temperature decreases the effect of phosphorus deficiency on the release of S-NR from roots and inhibits the release of S-UE from roots (e.g., DP and TP stresses) (p < 0.05) (Fig. 2b). Furthermore, drought or high temperature or the synergistic stresses of both decreases the effect of nitrogen and phosphorus deficiency on the inhibition of root secretion of S-UE, and the synergistic stresses of high temperature and drought significantly promote the release of S-NR from roots (e.g., DNP, TNP, and DTNP stresses) (p < 0.05) (Fig. 2c). Finally, the synergistic stresses of drought and high temperature significantly reduce the effect of drought on the release of S-UE from roots (e.g., DT stress) (p < 0.05) (Fig. 2d).
3.3 Phosphotransferase Activity in the Medium
The activity of S-PT in the culture medium was improved by D and T stress, whereas all stresses except N stress reduced the activity of S-PT in the culture medium (p < 0.05) (Fig. 3, Supplementary Table S3). Drought or high temperature or the synergistic stresses of drought and high temperature significantly reduce the effect of nitrogen deficiency on up-regulating S-PT activity (e.g., DN, TN, and DTN stresses) (p < 0.05) (Fig. 3a). Moreover, drought alleviates the effect of phosphorus deficiency on down-regulating S-PT activity, while the synergistic stresses of drought and high temperature aggravate the effect of phosphorus deficiency on down-regulating S-PT activity (e.g., DP and DTP stresses) (p < 0.05) (Fig. 3b). Furthermore, drought or high temperature or synergistic stresses of drought and high temperature alleviate the effect of nitrogen and phosphorus deficiency on the inhibition of S-PT activity (e.g., DNP, TNP, and DTNP stresses) (p < 0.05) (Fig. 3c). Finally, synergistic stresses of drought and high temperature inhibit the induction effect of a single factor on S-PT activity (e.g., DT stress) (p < 0.05) (Fig. 3d). This shows that under D and T, plants secrete S-PT through the root system to improve the transport and utilization of phosphorus, but most stresses are not conducive to the transport and utilization of phosphorus.
Figure 3: The root system secretes phosphotransferase activity in the culture medium. (a), (b), and (c) respectively represent the changes in phosphotransferases under the superimposed environmental factors of nitrogen, phosphorus, and nitrogen-phosphorus deficit stress. (d) Indicates the changes in phosphotransferase under the superposition of drought and high temperature. * indicates significant difference from CK treatments or significant difference between the two groups, p < 0.05
3.4 Catalase Activity in the Medium
N, DN, and TN stress significantly reduced the activity of S-CAT in the culture medium (p < 0.05), while the other groups did not show statistical significance (Fig. 4, Supplementary Table S3). In addition, synergistic stresses of drought and high temperature significantly decrease the effect of nitrogen deficiency in down-regulating S-CAT activity (e.g., DTN stress) (p < 0.05) (Fig. 4a), but the other groups were not statistically different. This shows that N, DN, and TN stresses are not conducive to removing root peroxide.
Figure 4: The root system secretes catalase activity in the culture medium. (a), (b), and (c) respectively represent the changes in catalase under the superimposed environmental factors of nitrogen, phosphorus, and nitrogen-phosphorus deficit stress. (d) Indicates the changes in catalase under the superimposed drought and high temperature. * indicates significant difference from CK treatments or significant difference between the two groups, p < 0.05
3.5 Polysaccharide Content in the Medium
P, DT, and DTP stress significantly reduced the polysaccharide content in the culture medium (p < 0.05), and all groups except DP, TP, and DN stresses tend to reduce the content of polysaccharides in the culture medium (Fig. 5, Supplementary Table S3). Additionally, drought or high temperature significantly alleviate the effects of the down-regulation of root polysaccharides secreted by phosphorus deficiency (e.g., DP and TP stresses) (p < 0.05) (Fig. 5b). However, the synergistic stresses of drought and high temperatures exacerbate the suppressive effects of drought (e.g., DT stress) (p < 0.05) (Fig. 5d). These results suggest that under abiotic stress, the root system of plants reduces the secretion of polysaccharides, thereby reducing the consumption of carbon and energy, but the effects of DP, TP, and DN stresses are not obvious.
Figure 5: Content of total polysaccharides secreted by roots in culture media. (a), (b), and (c) respectively represent the changes in polysaccharide content under the superimposed environmental factors of nitrogen, phosphorus, and nitrogen-phosphorus deficit stress. (d) Indicates the changes in polysaccharide content under superimposed drought and high temperature. * indicates significant difference from CK treatments or significant difference between the two groups, p < 0.05
3.6 Correlation of Soil Enzyme Activity and Polysaccharide Content with the Seedling Weight
The polysaccharide content in the culture medium showed a significant positive correlation with the fresh weight of S. miltiorrhiza seedlings and the fresh weight of their aboveground or underground parts (p < 0.05). Additionally, S-NR and S-PT were found to have an extremely significant negative correlation with the root-shoot ratio (p < 0.001), while S-NiR had a significant positive correlation with the root-shoot ratio (p < 0.05). Furthermore, S-UE had a significant positive correlation with S-NR and S-PT, whereas S-NiR had a significant negative correlation with S-NR (p < 0.05) (Fig. 6, Supplementary Table S3). These findings suggest that the release of polysaccharides from the root system into the environment is closely related to the growth and developmental status of the plant.
Figure 6: Correlation of soil enzyme activity and polysaccharide content with the seedling weight. The top right corner of the graph indicates correlation coefficients and the bottom left corner indicates significant differences in correlation. * indicates a significant correlation p < 0.05, ** indicates a strong significant correlation p < 0.01
Plants require nitrogen for the formation of new cells [29], and nitrogen deficiency is known to limit crop productivity [24]. Soil nitrogen exists in organic and inorganic forms, with nitrate and ammonium being the plant-available types [30]. Root secretion of nitrogen cycle enzymes in the soil has an impact on nitrogen utilization [31]. This experiment revealed that S-NR activity secreted by S. miltiorrhiza roots was significantly reduced under 15 different abiotic stress conditions. This suggests that under stress, S. miltiorrhiza maintains the supply of nitrate-nitrogen in the root environment by reducing the activity of root-secreted S-NR and reducing its nitrate-nitrogen reduction ability [32]. Similar studies have been performed in maize [33] and cowpea [34]. Reducing nitrogen loss is a strategy for plants to cope with abiotic adversity. It also helps to reduce the harmful impact of nitrite on the plant. However, the results of the present study differ from those of previous studies on the effects of drought [35] and nutrient stress [36] on root NR activity. This phenomenon may be closely related to the intricate interactions between microorganisms and root exudates. Root exudates attract a large number of microorganisms, including denitrifying bacteria. The dynamic action of denitrifying bacteria enhances the activity of nitrate reductase in the soil, leading to a cascade of transformations.
S. miltiorrhiza increases the root secretion of S-NIR activity under most stress conditions. This finding is consistent with other similar studies [37]. However, it is not consistent with the findings of tobacco [38]. Notably, S. miltiorrhiza significantly increased the activity of S-NiR in both DN and TN stresses, indicating improved denitrification, which may be related to the high temperature causing soil oxygen overflow [39]. The results of this study indicate that under stress, nitrogen utilization is initially controlled by the reduction of S-NR secreted by the root system. However, the resulting low concentrations of nitrite still pose a threat to the plant inter-root environment. To counteract the harmful effects of nitrite, plants must increase denitrification. Nitrite is converted to nitric oxide or other alternative forms by increasing the activity of the root-secreted S-NIR. This achieves a delicate balance between maximizing nitrogen use and avoiding nitrite damage in the root zone.
S-UE plays a critical role in converting organic nitrogen in soil into ammonium nitrogen available for plants. This study shows that S. miltiorrhiza significantly improves the activity of S-UE secreted by roots under N, D, T, DT, DN, DP, and TNP stresses. This finding is consistent with the observation that increasing temperature enhances the activity of S-UE in the soil [40]. Furthermore, nitrogen deficiency and its synergistic stress with drought significantly improve the activity of root-secreting S-UE. However, the synergistic stress of high temperature or synergistic stresses of drought and high temperature significantly reduces the activity of root-secreting S-UE (Fig. 2a) (e.g., DN, TN, and DTN stresses). Additionally, nitrogen deficiency, phosphorus deficiency, and their synergistic stress with drought or high temperature drought and high temperature significantly improve the activity of root-secreting S-UE (Fig. 2c) (e.g., DNP, TNP, and DTNP stresses). This study shows that plants respond uniquely to different stress combinations. The impact of each stressor on the plant is not simply additive. Under abiotic environmental stress, plants decrease nitrate nitrogen reduction and expedite organic nitrogen conversion to maintain plant-available nitrogen. This is achieved by modifying the types and activities of enzymes secreted by the root system, which are associated with the soil nitrogen cycle. Simultaneously, there is an increase in the reduction of nitrite to mitigate its damage, subsequently enhancing the efficiency of nitrogen conversion and utilization.
Phosphorus is one of the essential elements for plants [41], and the distribution and transport of soil phosphorus affect plant growth and productivity [42,43]. S-PT is an enzyme that catalyzes the transfer of phosphate groups from donors to acceptors and is closely related to phosphorus uptake and transport. This study shows that under D, T, and N stresses, S. miltiorrhiza improves the activity of root-secreted S-PT, while other stresses reduce this activity significantly. In the context of nitrogen stress, the synergistic stress of drought, high temperature, or synergistic stresses of drought and high temperature significantly reduce the activity of root-secreted S-PT (Fig. 3a) (e.g., DN, TN, and DTN stresses). However, under nitrogen and phosphorus stress, the synergistic stress of drought, high temperature or synergistic stresses of drought and high temperature synergistic stress significantly improve the activity of root-secreted S-PT (Fig. 3c) (e.g., DNP, TNP, and DTNP stresses). This finding further supports the notion that plants exhibit unique responses to different synergistic stresses. It is also suggested that in the absence of microbial participation, most stress environments are not conducive to phosphorus uptake and transport. Most stressful environments that promote phosphorus uptake and transport in plant roots may be directly related to rhizosphere microorganisms [44]. Exploring microbes that facilitate phosphorus absorption and movement in plants is another strategy to address these challenges.
The accumulation of reactive oxygen species (ROS) in the rhizosphere of plants causes oxidative damage to the cell membrane system of the root tip and leads to a series of physiological and biochemical changes in the root’s absorptive function [45]. Exposure to stressful environments can potentially contribute to the generation of ROS in plant organisms. The study shows that S. miltiorrhiza significantly reduced the activity of S-CAT secreted by the root under N, DN, and TN stresses. Under the synergistic stresses of three and four factors, S. miltiorrhiza tended to up-regulate the activity of root-secreted S-CAT. This is similar to the effect of nitrogen inhibiting the secretion of enzymes in maize roots [46] but different from the effect of drought stress stimulating the secretion of antioxidant substances in maize roots [47]. Overall, N, DN, and TN stresses can cause oxidative stress in the rhizosphere, which impacts the uptake and transport of rhizosphere nutrients. Additionally, this indicates that the accumulation of ROS is not simply additive to the stress factors, and plants do not respond to each stress factor in the same manner.
The saccharides secreted by plant roots are an important source of soil organic matter, microbial carbon, and energy, mainly hexoses and a small number of pentoses. Saccharides are also believed to be plant growth promoters. This study shows that the pentose content tends to increase under most stress conditions, but it is not synchronized with the change in hexose. Under N stress, the secretion of polysaccharides from S. miltiorrhiza roots was down-regulated, confirming that nitrogen stress significantly reduced the secretion of saccharides from maize roots [48,49]. The secretion of polysaccharides by the root system of S. miltiorrhiza was significantly down-regulated under P, DTP, and DT stress conditions. This differs from phosphorus deficiency, which stimulates sugar secretion [48], and drought stress stimulates polysaccharide secretion [50]. The release of root exudates positively correlates with microbial growth [51], suggesting that this result and the literature results may be related to the lack of microbial involvement in rhizosphere activities. Further analysis shows that the content of polysaccharides secreted by roots is positively correlated with plant yield. Abiotic stresses are unfavorable for plant biomass accumulation and may also impact the distribution of plant polysaccharides. Most energy substances, such as carbon source synthetic sugars, are supplied to plants, reducing the root secretion of polysaccharides that are not conducive to carbon sequestration in the soil. Thus, an unfavorable environment affects plant growth and also affects the carbon stored in the soil by the plants.
In conclusion, individual stressors have independent effects on the root secretion behavior of S. miltiorrhiza, and the combined effect of multiple stressors is not simply the sum of their individual effects. A balance between maximizing nitrogen utilization and preventing inter-root damage from nitrite salts was achieved by decreasing root-secreted S-NR activity and increasing S-NIR activity. However, sterile conditions are not favorable for soil phosphorus utilization and translocation or for reducing oxidative damage among roots by adjusting root S-CAT activity. Decreased plant polysaccharides secreted by the root system are unfavorable for soil carbon sequestration.
In this experiment, We revealed the effect of eliminating microbial interference on root secretion of various enzymes responsible for regulating the morphology and transport of soil elements. This study provides valuable insights into the adaptive mechanisms of plants under stress and their implications for soil nutrient cycling, plant growth, and soil carbon storage. The findings highlight the ability of these enzymes to cope with environmental stress. The tests showed that the roots of S. miltiorrhiza respond differently to each stress factor, demonstrating that the effects of multiple stressors cannot be merely added up. Plants obtain nutrients by altering the level of soil enzyme activity secreted by the root system, reducing oxidative damage to the root system. The exchange of root-produced sugars with root endophytes serves as a source of carbon and energy, while microbes mineralize soil organic nitrogen to release inorganic nutrients. Therefore, unfavorable environments directly impact plant growth, organic matter input, and soil carbon storage. Plants have limited resistance on their own, so enhancing the transportation and utilization of soil components is the key to improving plant resistance. In addition, fungi play a pivotal role in soil decomposition, especially in promoting nitrogen and phosphorus assimilation, and will undoubtedly be the focus of our subsequent research.
Acknowledgement: We would like to thank Dr. Dongmei He and his team for providing the sterile room.
Funding Statement: This research was funded by the National Natural Science Foundation of China (Grant Number 81973416), and this research was funded by the Science and Technology Department of Sichuan Province (2021YFS0045).
Author Contributions: Yong Qin: Conducting experiments, Formal analysis, Writing-original draft. Xiao-Yu Li: Data collection. Yan-Hong Wu: Conducting experiments, Paper check. Hai Wang: Content is critically revised, Paper check. Gui-Qi Han: Content is critically revised, Paper check. Zhu-Yun Yan: Experimental design, Methodology, Writing-review & editing, Supervision.
Availability of Data and Materials: All data is available in the main text and the supplementary materials.
Ethics Approval: Not applicable.
Conflicts of Interest: The authors declare that they have no known competing financial interests or personal relationships that could have appeared to influence the work reported in this paper.
Supplementary Materials: The supplementary material is available online at https://doi.org/10.32604/phyton.2023.046075.
References
1. Chai YN, Schachtman DP. Root exudates impact plant performance under abiotic stress. Trends Plant Sci. 2022;27(1):80–91. [Google Scholar] [PubMed]
2. Calvo OC, Franzaring J, Schmid I, Muller M, Brohon N, Fangmeier A. Atmospheric CO2 enrichment and drought stress modify root exudation of barley. Glob Chang Biol. 2017;23(3):1292–304. [Google Scholar] [PubMed]
3. Wang NQ, Kong CH, Wang P, Meiners SJ. Root exudate signals in plant-plant interactions. Plant Cell Environ. 2021;44(4):1044–58. [Google Scholar] [PubMed]
4. Panchal P, Preece C, Penuelas J, Giri J. Soil carbon sequestration by root exudates. Trends Plant Sci. 2022;27(8):749–57. [Google Scholar] [PubMed]
5. Pramanik P, Phukan M. Potential of tea plants in carbon sequestration in North-East India. Environ Monit Assess. 2020;192(4):211. [Google Scholar] [PubMed]
6. Lu T, Zhang Z, Li Y, Zhang Q, Cui H, Sun L, et al. Does biological rhythm transmit from plants to rhizosphere microbes? Environ Microbiol. 2021;23(11):6895–6906. [Google Scholar] [PubMed]
7. Tiziani R, Miras-Moreno B, Malacrinò A, Vescio R, Lucini L, Mimmo T, et al. Drought, heat, and their combination impact the root exudation patterns and rhizosphere microbiome in maize roots. Environ Exp Bot. 2022;203:105071. [Google Scholar]
8. Vives-Peris V, Molina L, Segura A, Gomez-Cadenas A, Perez-Clemente RM. Root exudates from citrus plants subjected to abiotic stress conditions have a positive effect on rhizobacteria. J Plant Physiol. 2018;228:208–17. [Google Scholar] [PubMed]
9. Malek S, Wazny R, Blonska E, Jasik M, Lasota J. Soil fungal diversity and biological activity as indicators of fertilization strategies in a forest ecosystem after spruce disintegration in the Karpaty Mountains. Sci Total Environ. 2021;751:142335. [Google Scholar] [PubMed]
10. Liu JG, Liu WG. Advances in microbial-mediated nitrogen cycling. Acta Agrestia Sinica. 2018;26(2):277–83. [Google Scholar]
11. Fisher KA, Yarwood SA, James BR. Soil urease activity and bacterial ureC gene copy numbers: effect of pH. Geoderma. 2017;285(4):1–8. [Google Scholar]
12. Wohlgemuth R, Liese A, Streit W. Biocatalytic phosphorylations of metabolites: past, present, and future. Trend Biotechnol. 2017;35(5):452–65. [Google Scholar]
13. Cusack DF, Silver WL, Torn MS, Burton SD, Firestone MK. Changes in microbial community characteristics and soil organic matter with nitrogen additions in two tropical forests. Ecology. 2011;92(3):621–32. [Google Scholar] [PubMed]
14. Morcillo RJL, Manzanera M. The effects of plant-associated bacterial exopolysaccharides on plant abiotic stress tolerance. Metabolite. 2021;11(6):337. [Google Scholar]
15. Leifeld J, Siebert S, Kögel-Knabner I. Changes in the chemical composition of soil organic matter after application of compost. Eur J Soil Sci. 2002;53(2):299–309. [Google Scholar]
16. Staszel K, Lasota J, Blonska E. Effect of drought on root exudates from Quercus petraea and enzymatic activity of soil. Sci Rep. 2022;12(1):7635. [Google Scholar] [PubMed]
17. Jia XY, Zhong YQW, Liu J, Zhu GY, Shangguan ZP, Yan WM. Effects of nitrogen enrichment on soil microbial characteristics: from biomass to enzyme activities. Geoderma. 2020;366:114256. [Google Scholar]
18. Yuan JS, Himanen SJ, Holopainen JK, Chen F, Stewart Jr CN. Smelling global climate change: mitigation of function for plant volatile organic compounds. Trends Ecol Evol. 2009;24(6):323–31. [Google Scholar] [PubMed]
19. Zhang YQ, Deng QL, Wen QS, Xiao JY, Yu ZH, Li SJ, et al. Brief discussion about role and measures of scientific fertilization in ecological cultivation of Chinese medicinal materials. Zhongguo Zhong Yao Za Zhi. 2020;45(20):4846–52 (In Chinese). [Google Scholar]
20. Ren J, Fu L, Nile SH, Zhang J, Kai G. Salvia miltiorrhiza in treating cardiovascular diseases: a review on its pharmacological and clinical applications. Front Pharmacol. 2019;10:753. [Google Scholar] [PubMed]
21. Caser M, Chitarra W, D’Angiolillo F, Perrone I, Demasi S, Lovisolo C, et al. Drought stress adaptation modulates plant secondary metabolite production in Salvia dolomitica Codd. Ind Crops Prod. 2019;129:85–96. [Google Scholar]
22. Lu LL, Zhang YX, Yang YF. Integrative transcriptomic and metabolomic analyses unveil tanshinone biosynthesis in Salvia miltiorrhiza root under N starvation stress. PLoS One. 2022;17(8):e0273495. doi:https://doi.org/10.1371/journal.pone.0273495. [Google Scholar] [PubMed] [CrossRef]
23. Torres-Rodriguez JV, Salazar-Vidal MN, Chavez Montes RA, Massange-Sanchez JA, Gillmor CS, Sawers RJH. Low nitrogen availability inhibits the phosphorus starvation response in maize (Zea mays ssp. mays L.). BMC Plant Biol. 2021;21(1):259. [Google Scholar] [PubMed]
24. Zhao D, Reddy KR, Kakani VG, Reddy VR. Nitrogen deficiency effects on plant growth, leaf photosynthesis, and hyperspectral reflectance properties of sorghum. Eur J Agron. 2005;22(4):391–403. [Google Scholar]
25. Liu HY, Wang XD, Wang DH, Zou ZR, Liang ZS. Effect of drought stress on growth and accumulation of active constituents in Salvia miltiorrhiza Bunge. Ind Crops Prod. 2011;33(1):84–8. [Google Scholar]
26. Li HL, Li JL, Zhang XF. Orcinol hydrochloric acid method for determination of pentosans in oat β-glucan. Guangdong Chem Ind. 2014;41(17):179–80 (In Chinese). [Google Scholar]
27. Sun XY, Cai CL, Xu LL, Wang Q. Comparison of methods for determination of polysaccharide content. Res Prac Chinese Med. 2015;29(3):58–62 (In Chinese). [Google Scholar]
28. Pansu M, Gautheyrou J. Handbook of soil analysis. Springer; 2006. p. 629–43. [Google Scholar]
29. Li K, Zhou XH, Li FJ, Hu DN, Guo XM, Yu SQ, et al. Response of growth and nitrogen balance of Camellia oleifera seedlings under different nitrogen concentrations. South China Forest Sci. 2020;48(5):1–6+48 (In Chinese). [Google Scholar]
30. Xu G, Fan X, Miller AJ. Plant nitrogen assimilation and use efficiency. Annu Rev Plant Biol. 2012;63(1):153–82. [Google Scholar] [PubMed]
31. Dong HY, Sun HY, Fan SX, Jiang LL, Ma DR. Rhizobacterial communities, enzyme activity, and soil properties affect rice seedling’s nitrogen use. Agr J. 2021;113(1):633–44. [Google Scholar]
32. Coskun D, Britto DT, Shi W, Kronzucker HJ. How plant root exudates shape the nitrogen cycle. Trends Plant Sci. 2017;22(8):661–73. [Google Scholar] [PubMed]
33. Qiang L, Yun R, Yong Z, Jing L, Yuan JC. Differences in nitrogen metabolism and dry matter production between maize cultivars and different nitrogen efficiencies under low nitroger stress. Acta Agriculturae Boreali-occidentalis Sinica. 2021;30(5):672–80 (In Chinese). [Google Scholar]
34. Qi BL, Cao CL, Wang F, Lei ZP, Zhao QR, Li J. Influence of low phosphorus on nitrate reductive activity and NO3-N content in cowpea seedling. Agric Res Arid Areas. 2010;28(1):147–51. [Google Scholar]
35. Hosseini SS, Lakzian A, Razavi BS. Reduction in root active zones under drought stress controls spatial distribution and catalytic efficiency of enzyme activities in rhizosphere of wheat. Rhizosphere. 2022;23:100561. [Google Scholar]
36. Meng H, Li SC, Chen YM, Jiao X, Sui YY. Effects of irrigation and fertilizers management on nitrate reductase activities in Mollisols under facility eggplant field. Soil Crop. 2021;10(2):187–93. [Google Scholar]
37. Zhu L, Li Y, Wang Y, Wang YY, Zhang XY, Chen AJ, et al. Effects of nitrogen application on maize nitrogen uptake and soil biological and chemical properties under drought stresses at seedling stage. J Soil Water Conserv. 2021;35(4):267–74. [Google Scholar]
38. Liang XH, Gong SY, Ni JY, Yang SQ, Wang ZX, Wang J, et al. Response of key enzymes activity of nitrogen metabolism to low nitrogen stress in different genotypes tobacco. Mol Breeding. 2020;182(2):7554–61. [Google Scholar]
39. Guan HL, Yang JZ, Chen YJ, Cui XM, Wang Y, Zhang YF. Change of rhizospheric microbe colony in cultivated soil and its correlation to root rot disease in Panax Notoginseng. Soil. 2010;42(3):378–84. [Google Scholar]
40. Bai Y, Li F, Yang G, Shi S, Dong F, Liu M, et al. Meta-analysis of experimental warming on soil invertase and urease activities. Acta Agriculturae Scandinavica, Section B—Soi Plant Sci. 2017;68(2):104–9. [Google Scholar]
41. Arwenyo B, Varco JJ, Dygert A, Mlsna T. Phosphorus availability from magnesium-modified P-enriched Douglas fir biochar as a controlled release fertilizer. Soil Use Manage. 2022;38(1):691–702. [Google Scholar]
42. Xia Z, He Y, Yu L, Lv R, Korpelainen H, Li C. Sex-specific strategies of phosphorus (P) acquisition in Populus cathayana as affected by soil P availability and distribution. New Phytol. 2020;225(2):782–92. [Google Scholar] [PubMed]
43. Yadav S, Kanwar RS, Patil JA, Tomar D. Effects of Heterodera avenae on the absorption and translocation of N, P, K, and Zn from the soil in wheat. J Plant Nutr. 2020;43(17):2549–56. [Google Scholar]
44. Glaesner N, Baelum J, Jacobsen CS, Ritz C, Rubaek GH, Kjaergaard C, et al. Bacteria as transporters of phosphorus through soil. Eur J Soil Sci. 2016;67(1):99–108. [Google Scholar]
45. Mittler R, Zandalinas SI, Fichman Y, Van Breusegem F. Reactive oxygen species signalling in plant stress responses. Nat Rev Mol Cell Biol. 2022;23(10):663–79. [Google Scholar] [PubMed]
46. Liang GP, Houssou AA, Wu HJ, Wu XP, Cai DX, Gao LL, et al. Soil nitrogen content and enzyme activities in rhizosphere and non-rhizosphere of summer maize under different nitrogen application rates. Ying Yong Sheng Tai Xue Bao. 2016;27(6):1917–24 (In Chinese). [Google Scholar] [PubMed]
47. Song FB, Han XY, Zhu XC, Herbert SJ. Response to water stress of soil enzymes and root exudates from drought and non-drought tolerant corn hybrids at different growth stages. Can J Soil Sci. 2012;92(3):501–7. [Google Scholar]
48. Carvalhais LC, Dennis PG, Fedoseyenko D, Hajirezaei MR, Borriss R, von Wirén N. Root exudation of sugars, amino acids, and organic acids by maize as affected by nitrogen, phosphorus, potassium, and iron deficiency. J Plant Nutr Soil Sci. 2010;74(1):3–11. [Google Scholar]
49. Zhu S, Vivanco JM, Manter DK. Nitrogen fertilizer rate affects root exudation, the rhizosphere microbiome and nitrogen-use-efficiency of maize. Appl Soil Eco. 2016;107:324–33. [Google Scholar]
50. Ulrich DEM, Clendinen CS, Alongi F, Mueller RC, Chu RK, Toyoda J, et al. Root exudate composition reflects drought severity gradient in blue grama (Bouteloua gracilis). Sci Rep. 2022;12(1):12581. [Google Scholar] [PubMed]
51. Bengtson P, Barker J, Grayston SJ. Evidence of a strong coupling between root exudation, C and N availability, and stimulated SOM decomposition caused by rhizosphere priming effects. Ecol Evol. 2012;28:1843–52. [Google Scholar]
Cite This Article
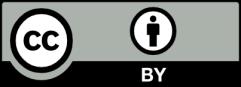