Open Access
REVIEW
Microglial TRPV1 in epilepsy: Is it druggable for new antiepileptic treatment?
The First Affiliated Hospital of Yangtze University, Jingzhou, 434000, China
* Corresponding Author: XIANGLIN CHENG. Email:
(This article belongs to the Special Issue: Neuroimmune Interactions at the Crossroads of Health and Disease)
BIOCELL 2023, 47(8), 1689-1701. https://doi.org/10.32604/biocell.2023.029409
Received 16 February 2023; Accepted 08 May 2023; Issue published 28 August 2023
Abstract
Epilepsy is one of the most common neurological diseases worldwide with a high prevalence and unknown pathogenesis. Further, its control is challenging. It is generally accepted that an imbalance between the excitatory and inhibitory properties of the central nervous system (CNS) leads to a large number of abnormally synchronized neuronal discharges in the brain. Transient receptor potential vanilloid protein type 1 (TRPV1) is a non-selective cation channel that contributes to the regulation of the nervous system and influences the excitability of the nervous system. This includes the release of neurotransmitters, action potential generation due to alterations in ion channels, synaptic transmission, and the changes in glial cells. There is abundant evidence that TRPV1 is widely expressed in the central nervous system (including microglia) and is involved in the development of epilepsy through neuroinflammation. In conclusion, microglial TRPV1 participates in neuroinflammatory reactions and functions as a potential proinflammatory mediator. This presents a novel treatment approach to regulate seizures brought on by neuroinflammation.Keywords
Epilepsy is a chronic and recurrent seizure disorder caused by abnormal neuronal discharges that result in brain dysfunction. The etiology of epilepsy falls into the following categories: structural, genetic, infectious, metabolic, immunological, and unknown causes (Scheffer et al., 2017). Currently, the targets of clinical antiepileptic drugs (AEDs) include ion channels, neurotransmitter transporters, and neurotransmitter metabolizing enzymes. Recently, TRPV1 channels are emerging as a new potential antiepileptic target, according to many studies. TRPV1 is widely expressed in the CNS such as in the hippocampus, cortex, cerebellum, olfactory bulb, midbrain, hindbrain, caudate nucleus, thalamus, hypothalamus, and basal ganglia regions (Toth et al., 2005). Further, it is highly expressed in the hippocampus, the cytoplasm of cortical neurons and dendrites (Hurtado-Zavala et al., 2017). In addition, TRPV1 is also expressed in microglia (Kong et al., 2019). Hence, the central nervous system shows high levels of TRPV1 expression, particularly in microglia. Microglia are the resident immune cells of the central nervous system, accounting for about 5%–12% of brain cells (Lawson et al., 1990). The morphological changes of microglia reflect the degree of their activation. There are generally two types of morphologies: the resting state, which is characterized by a small branching cell body, and the active state, which is characterized by a large cell body and shortened protrusions like the shape of amoebas. In the development of epilepsy, microglia were shown to actively monitor their surroundings and regulate neurogenesis (Cunningham et al., 2013), and also promoted neurons survival (Ueno et al., 2013). Reports also showed they phagocytose neurons (Svahn et al., 2013), regulate axonal connections (Squarzoni et al., 2014), induce synapse formation (Miyamoto et al., 2016), and phagocytosis synapses. Microglia also affect how the brain functions by interacting with synapses. They also support the preservation of synaptic function and the development of learner-dependent synapses (Tremblay et al., 2010; Wang et al., 2016) (Fig. 1). Different studies have shown that inflammatory processes driven by glial cell activation and proinflammatory cytokine release cause neuronal damage that leads to epileptogenesis, a potential epilepsy-related process (Devinsky et al., 2013; Vezzani, 2014). However, due to the complex mechanism of microglial TRPV1 in epilepsy, the development of related drugs still faces many challenges. In this article, we will mainly review the role and molecular mechanism of TRPV1 channels in microglia in epilepsy to provide theoretical support for the treatment of epilepsy and the development of drugs related to TRPV1 channels in microglia.
Figure 1: The role of microglia in the central nervous system.
Transient Receptor Potential Vanilloid Protein Type 1 Channel
An overview of the transient receptor potential vanilloid protein type 1
TRPV1 is a nonselective cation channel that was first reported in 1997 in sensory neurons including dorsal root ganglion (DRG) and trigeminal ganglion neurons (Caterina et al., 1997). Using cryo-electron microscopy, it was found that there is a pore between the fifth and sixth transmembrane structural domains of the channel, which has six transmembrane structural domains. The channel also has cytosolic N- and C-terminal binding sites for substances that control TRPV1 activity such as calmodulin, or CaM, and ATP binding sites at the N- and C-terminals. TRPV1 exhibits high divalent selectivity, and activation produces a significant flux of Ca2+ into cells (Liao et al., 2013). A study used a pharmacological probe (peptide toxin and small vanilloid-like agonist) to confirm that the capsaicin receptor TRPV1 has a unique double-gating mechanism (Cao et al., 2013).
Further, physical, chemical, endogenous, and external stimuli can all activate TRPV1 channels. For instance, TRPV1 could be activated by hazardous heat (>42°C) (Tominaga et al., 1998), an acid solution (pH < 6.5) (Tominaga et al., 1998), oxidative stress (NO, hydrogen peroxide), endogenous lipid-derived molecules (unsaturated N-acyldopamine, lipoxygenase products of arachidonic acid, and endocannabinoids and some of their homologs) (van der Stelt and di Marzo, 2004), food components(capsaicin) and toxins (resinous toxins and vanilloid toxins) (Cao et al., 2013). Additionally, exogenous substances such as evodiamine, rutacine alkaloids, and M-channel blocker linobidine were also shown to activate TRPV1 (Neacsu and Babes, 2010; Ivanova and Spiteller, 2014).
TRPV1 channels were initially found to be expressed mainly in neurons associated with injury perception. Hence, the majority of the early research on TRPV1 focused on its function in damage perception, and the intervention studies on TRPV1 drugs were mainly aimed at the treatment of pain. However, a growing number of studies have found that TRPV1 is also expressed in neurons that are not related to nociceptive sensation and in many different non-neuronal tissues (Szallasi et al., 2007). Further, the brain was discovered to have high levels of TRPV1 channel expression, particularly in synapses (mainly in the postsynaptic zone but also in presynaptic neurons), and the astrocyte endfeet and pericytes by using immunohistochemistry, RT-PCR, and in situ hybridization. The use of double-labeled immunofluorescence colocalization with glutamate and γ-aminobutyric acid (GABA) indicated that TRPV1 is distributed on both glutamatergic and GABAergic neurons (Sun et al., 2013). Given that the level and localization of TRPV1 show a wide variation among studies, despite the abundance of research studies, the presence of TRPV1 in the brain is still a contentious topic (Menigoz and Boudes, 2011). Interestingly, the use of TRPV1 reporter mice revealed robust expression of TRPV1 in primary afferent neurons, with very low-level expression in several discrete brain regions, most notably within and near the caudal hypothalamus (Cavanaugh et al., 2011). This difference may be caused by the transient expression of TRPV1 during development. Other investigators have used reporter mice to show that the specific medulla regions are innervated by TRPV1 vagal afferent subsets (Kim et al., 2020). Understanding the location of TRPV1 helps to clarify its potential significance in epilepsy and is consistent with its several roles.
The transient receptor potential vanilloid protein type 1 channel and epilepsy
Recently, several studies attempted to answer the question regarding the physiological and behavioral relevance of TRPV1 in epilepsy (Fig. 2). Experimental studies have shown that in pilocarpine-treated mice, TRPV1 protein levels in the dentate gyrus were increased after undergoing status epilepticus (Bhaskaran and Smith, 2010). It was also shown that TRPV1 is expressed not only in the plasma membranes but also in the endoplasmic reticulum and calcium storage vesicles (Marshall et al., 2003). Another report revealed that TRPV1 influences the pathological course of epilepsy through the regulation of synaptic plasticity (Saffarzadeh et al., 2015). Synaptic plasticity is mainly characterized by long-temporal inhibition of long-term potentiation (LTP) and long-term depression (LTD) (Bear and Malenka, 1994). Further, some researchers found that TRPV1 was associated with LTP changes in pilocarpine-induced status epilepticus (Saffarzadeh et al., 2015). In one study, the in vivo induction of mossy cells (MCs) and granule cells (GC) by LTP exacerbated kainic acid (KA)-induced seizures (Nasrallah et al., 2022). In animal models of epilepsy studies, it was found that the synaptic connections in the granule cells of the dentate gyrus of the epileptic hippocampus are similar to those of LTP. Further, alterations in these synaptic connections in LTP increase the efficacy of synaptic transmission and cause increased excitability of the corresponding neurons, which may be associated with epileptogenesis (Leite et al., 2005). TRPV1-mediated LTD may play a role in physiological and pathological changes during epileptic activity (Gibson et al., 2008). In one report, the activation of TRPV1 facilitated LTP and suppressed LTD (Li et al., 2008). Additionally, nerve growth factor (NGF) has been proven to be a regulator of TRPV1 expression (Chuang et al., 2001). NGF enhances TRPV1 function and total phosphorylation. NGF acts on TrkA receptors and increases TRPV1 expression levels by phosphorylating TRPV1 at a single tyrosine residue (Zhang et al., 2005). However, the phosphorylation of individual tyrosines enhances mossy fiber sprouting, exacerbates neuronal damage, and accelerates seizure development (Adams et al., 1997).
Figure 2: Transient receptor potential vanilloid protein type 1 (TRPV1) channel activation leads to a large increase in calcium (Ca2+). It has been reported that mitochondria accumulate Ca2+, in which case there is a large amount of Ca2+ in the cell. In excessive amounts, Ca2+ can result in significant mitochondrial permeability shift or even mitochondrial membrane rupture, and outer membrane rupture causes huge swelling of the mitochondria and the release of molecules that trigger apoptosis, including caspase3 and caspase9 and ROS in high levels. Heat treatment (over 42°C), acidic solutions (pH < 6.5), oxidative stress (NO, hydrogen peroxide), endogenous lipid derivative compounds (such as unsaturated N-acyl dopamine, arachidonic acid lipoxygenase product, and some endocannabinoids and their homologs), food ingredients (for instance, capsaicin), toxins (examples include resin toxins and vanilla toxins), and exogenous substances all induce Ca2+ accumulation through desensitization of TRPV1 channels. Moreover, by activating TRPV1 channels, it increases the rate of glutamate release spontaneously from presynaptic terminals to neurons.
Additionally, TRPV1 activation could affect excitatory or inhibitory neurotransmitter release (Shoudai et al., 2010; Chavez et al., 2014). Studies have shown that TRPV1 is present in synapses, presynaptic neurons, interneurons, and postsynaptic neurons (Zhang et al., 2020). Reports on the function of TRPV1 in synaptic plasticity have found that TRPV1 can not only regulate excitatory synaptic input but also target inhibitory synapses. For example, cells in glutamatergic synaptic circuits in the hippocampus and endocannabinoids regulate inhibitory synaptic transmission by targeting TRPV1 channels (Chavez et al., 2014; Lee et al., 2015). Looking at the control control of synaptic transmission by TRPV1 in the CNS, it was widely believed that TRPV1 activation not only regulates synaptic transmission through presynaptic mechanisms but also participates in synaptic transmission through postsynaptic mechanisms (Doyle et al., 2002; Marinelli et al., 2003). TRPV1 can be expressed in both pre-synaptic and post-synaptic conditions and can increase or decrease the release of neurotransmission depending on the specific synapse. For instance, presynaptic TRPV1 usually increases glutamate neurotransmitter release by increasing presynaptic calcium concentration. Similarly, activation of this receptor in the striatum enhances glutamate and epinephrine/norepinephrine release in this brain region (Marinelli et al., 2002). TRPV1 was also implicated in the modulation of GABAergic transmission and glutamatergic transmission. For example, using a combination of pharmacology, electrophysiology, and an in vivo knockdown strategy, it was demonstrated that activation of TRPV1 by capsaicin or an endocannabinoid inhibited somatic but not dendritic inhibitory GABAergic transmission in the dentate gyrus of rats and mice (Chavez et al., 2014). The postsynaptic mechanisms of TRPV1 including calcineurin and chained lipoprotein-dependent AMPA receptor internalization prevented excitatory transmission in the dentate gyrus of rats and mice. Additionally, TRPV1-dependent regulation of excitatory propagation in the nucleus accumbens is also mediated via postsynaptic processes, like endocytosis of α-amino-3-hydroxy-5-methyl-4-isoxazolepropionic acid (AMPA) receptors. (Chavez et al., 2010; Grueter et al., 2010). Although the precise localization of TRPV1 remains controversial, it has been demonstrated to control synaptic efficacy in the postsynaptic compartments and neurotransmitter release from presynaptic terminals. Further, TRPV1 can alter the induction of additional types of plasticity in addition to taking part in these types of synaptic plasticity mechanisms. Recently, it was found that TRPV1 can affect transmission between synapses, and TRPV1 influences synaptic transmission through the calcium ion concentration. Synaptic transmission in the prefrontal cortex and the effects of capsaicin and endogenous N-arachidonoyl taurine on TRPV1 activation were also explored. It has been demonstrated in mice that the exogenous agonist capsaicin activates TRPV1, which controls synaptic activity in glutamatergic and GABAergic synaptic transmission. Furthermore, TRPV1 activation by the naturally occurring activator N-arachidonic acyl taurine (NAT) also resulted in outcomes comparable to those of capsaicin. Taurine, a NAT catabolic product, on the other hand, significantly reduced the transmission of such induced glutamatergic synapses. TRPV1 may also be found in inhibitory interneurons according to immunohistochemistry reports (Hurtado-Zavala et al., 2017). The findings consistently demonstrate that TRPV1 enhances excitatory innervation with a decreased frequency of miniature inhibitory postsynaptic currents (mIPSCs) in foramen ovale molecule (OLM) interneurons in the hippocampus. Endocannabinoids (eCB), which are generally released from postsynaptic compartments and work retrogradely to block synaptic transmission, are also produced at a higher rate as a result of TRPV1 activation (Chavez et al., 2014).
In the pentylenetetrazol-induced epilepsy rats, hippocampal neurons showed higher apoptosis, mitochondrial membrane depolarization, intracellular reactive oxygen species (ROS), and caspase-3 and caspase-9 values via TRPV1 channels than in normal rats (Naziroglu et al., 2015). Further, increased ROS generation is linked to TRPV1 activation given that ROS can also influence this signaling pathway. Nitric oxide (NO) and reactive oxygen species can cause amino acid residues to be phosphorylated to aggravate the toxic damage to neurons. There is proof that TRPV1 activation boosts ROS production, disturbs the mitochondrial membrane potential, and causes an imbalance in the level of Ca2+ in cells. Reactive substances (RSs) such as ROS and reactive nitrogen species (RNS) produced in excess were shown to gradually impair intracellular Ca2+ homeostasis. This made neurons more vulnerable to further stress and activated cell apoptotic pathways (Olowe et al., 2020). The findings on the expression and function of TRPV1 in epileptic animal models or epileptic patients are summarized in Table 1.
In contrast to the proconvulsive roles described above, TRPV1 activation has also been linked to anticonvulsant effects in a number of preclinical models (Jiang and Yu, 2023). Many animal studies show that capsaicin exerts both anti- and pro-epileptic effects. For instance, the activation of the TRPV1 receptors with capsaicin was also shown to induce neuronal death both in vitro and in vivo (Gonzalez-Reyes et al., 2013). The administration of the TRPV1 channel agonist capsaicin enhanced epileptic activity in rat hippocampal. Further, mice with TRPV1 receptor blockers have higher seizure thresholds (Socala et al., 2015). Additionally, capsaicin reduced the severity of brain oxidative stress, seizures, and neuronal damage and provided neuronal protection (Lee et al., 2011; Abdel-Salam et al., 2020; Pasierski and Szulczyk, 2022; Tyagi et al., 2022). Capsaicin may exert its antiepileptic effects by causing TRPV1 dephosphorylation through a calcium-dependent mechanism (Koplas et al., 1997). In line with these findings, a recent study suggests that cannabidiol (CBD), a TRPV1 agonist, also has anticonvulsant and neuroprotective effects (Gray and Whalley, 2020). In contrast, other studies indicated that the seizure threshold was reduced thus facilitating epileptogenesis (Wallace et al., 2002). Additonally, TRPV1 was strongly phosphorylated in epileptic hippocampal tissue with both capsaicin and CBD inducing TRPV1 dephosphorylation (Iannotti et al., 2014). Further, many studies now indicate that TRPV1 has physiological relevance for epilepsy, with reported functions including those in rodent models. For example, TRPV1 knockdown reduces epilepsy susceptibility (Barrett et al., 2016). A TRPV1 knock-out mouse model showed a prolonged onset latency and a shortened duration and seizure grade of febrile seizures when compared with wild type (WT) mice based on behavioral testing for febrile seizures (Huang et al., 2015). In addition, TRPV1 gene deficiency decreased the susceptibility to PTZ-induced seizures following early-life hyperthermia challenges in mice (Kong et al., 2014).
Microglia, Neurons and Epilepsy
Many pathogenic effects, including neuronal loss, mossy fiber sprouting, hippocampus glial proliferation, and synaptic remodeling are caused by adult convulsions (Wolf et al., 1993; Cendes et al., 1995). Many studies have shown that microglia proliferate and secrete proinflammatory factors after epileptogenesis, causing glial scarring. Microglia also respond to the epileptic environment and alter their activity by releasing proinflammatory and anti-inflammatory cytokines (Benson et al., 2015), engulfing apoptotic and viable cells (Luo et al., 2016), phagocytosing synapses (Wyatt et al., 2017), and cause synaptic stripping Microglia display a diverse phenotype in the epileptic brain that can either be epileptic or antiepileptic. It has been suggested that while brief microglial activation is advantageous (Vinet et al., 2012), the development of epilepsy is adversely affected by persistent microglial activation (Qin et al., 2007). Hence, it is critical to understand how microglia change over each stage of epileptogenesis. Microglia regulate hippocampal structure and function after early seizures (Andoh et al., 2020), neurogenesis, mossy fiber sprouting from granulosa cells, and hyperexcitability (increased excitatory neurotransmitters (Shoudai et al., 2010) and decreased inhibitory neurotransmitters (Chavez et al., 2014)). Microglia may also regulate mossy fiber sprouting early in epileptic seizures. Studies have shown that in a neonatal rat model of sepsis, interleukin-1β (IL-1β) production by microglia decreased axon elongation factor (Han et al., 2017). Following glutardiazole-induced convulsions in rats, the expression level of repulsive guidance molecule-a(RGMa), a molecule that prevents mossy fiber budding, was also found to be diminished. Subsequent to spinal cord damage in rats, active microglia showed elevated RGMa expression, which slowed axonal development. These results imply that convulsions may lower RGMa microglial expression in the hippocampus, facilitating mossy fiber elongation and branching (Li et al., 2012; Song et al., 2015).
A pathological alteration documented in epilepsy is extensive synaptic remodeling. Microglia form neural circuits through the release of soluble factors and direct physical contact and also participate in neural network elimination and synapse formation (Rice et al., 2015). Small, soluble molecules known as soluble cytokines are involved in intercellular communication, which control the development, survival, differentiation, and function of cells. These include colony-stimulating factors, insulin-like growth factor (IGF), tumor necrosis factor (TNF), interferons (IFN), interleukins, chemokines, transforming growth factor (TGF), and neurotrophic factors like NGF and brain-derived neurotrophic factor (BDNF). TNF was shown to influence synaptic plasticity by interacting with the AMPA receptor (Parkhurst et al., 2013). However, excessive amounts of cytokines such as IL-1, IL-18, IFN, and TNFα have a detrimental effect on neurons and impair synaptic plasticity. Microglia contribute to synaptic formation and remodeling during development and regulate synaptic enhancement and plasticity through IL-1β release. Epileptic neuronal hyperexcitability enhances neuronal interaction with microglia, microglia activation, and IL-1β release. GABA is an inhibitory neurotransmitter that can regulate the remodeling and pruning of inhibitory synapses during neural development by acting on microglia. Patients with temporal lobe seizures have significantly more numbers of microglia in their neurons. GABA in microglia can slow down the presynaptic migration of microglia mediated by P2Y12 receptors, thereby reducing neuronal excitability and seizure susceptibility (Wan et al., 2020). Mice lacking P2Y12 receptors exhibit reduced microglia-neuron interactions and increased severe seizures during status epilepticus (Badimon et al., 2020). It has also been shown that the complement cascade systems C1q, C3, C4, and CD47-SIRPα are involved in synaptic eliminationin the brain (Stevens et al., 2007; Lehrman et al., 2018; Sekar et al., 2022). Further, genetic disruption of the microglia-specific protein DAP12 increased LTP and synaptic plasticity. Alterations in the glutamate receptor content at synapses and microglial brain-derived neurotrophic factor were proposed as the causes of these changes (Roumier et al., 2004). Microglia also display calcium level increases in response to increased neuronal activity with microglia having strong calcium activity during kainate administration-induced status epilepticus (Umpierre et al., 2020). Using high-resolution two-photon imaging in vivo and in vitro, investigators observed a significant increase in microglial process convergence (MPC) following experimental seizures induced by kainic acid or pilocarpine. A deficiency of the fractalkine receptor (CX3CR1) decreased MPC, while fractalkine (CX3CL1) treatment increased MPC. This suggests that fractalkine signaling is a key regulator of these microglia-neuron interactions (Eyo et al., 2016).
Microglia express a variety of neurotransmitter receptors, such as glutamate receptors, GABA receptors, and purinergic receptors to name a few. For instance, primary cultured rat microglia expressed functional AMPA-kainateGluRs, and stimulation of AMPA-kainateGluRs promoted the release of TNFα (Noda et al., 2000). Microglia also express purinergic receptors, which upon activation affect the release of potassium channels and cytokines from microglia and are essential for the maintenance of membrane resting potential and cytokine release. In addition, microglia also release ATP or adenosine, a signal that plays a key role in epileptogenesis, and experiments have shown increased expression of microglia purinergic receptors in patients with seizures and temporal lobe epilepsy (Ulmann et al., 2013). Microglia feedback inhibits neuronal activity while the elimination of microglia promotes epilepsy, and the ATP-adenosine-A1R pathway mediates its feedback inhibition.
Microglia Transient Receptor Potential Vanilloid Protein Type 1 and Epilepsy
The role of transient receptor potential vanilloid protein type 1 in microglial cells
Several studies on microglia and microglia cell lines have shown that TRPV1 is expressed in microglia cell membranes and in the membranes of microglial intracellular organelles (Marrone et al., 2017). These include the mitochondria, endoplasmic reticulum (ER), lysosomes, and the Golgi apparatus (Miyake et al., 2015). TRPV1 activation results in a variety of outcomes, including changes in microglial morphology, phagocytosis and death, cell migration, the production of cytokines, and the formation of ROS (Kim et al., 2006; Sappington and Calkins, 2008; Schilling and Eder, 2009) (Fig. 3).
Figure 3: The mechanisms associated with transient receptor potential vanilloid protein type 1 (TRPV1) in epilepsy: In animal models of epilepsy, TRPV1 activation exacerbates the pathological process of epilepsy by altering the release of excitatory and inhibitory neurotransmitters, oxidative stress, prominent remodeling, and mossy fiber outgrowth. In contrast, persistent epilepsy exacerbates oxidative neuronal damage, mossy outgrowth, and hippocampal sclerosis. Microglia TRPV1 can exacerbate neuronal damage by releasing cytokines, and inflammatory mediators, to activate microglia, leading to epilepsy.
In a report, TRPV1 could promote microglial injury through calcium signaling and mitochondrial destruction. TRPV1 promoted both in vivo and in vitro microglial cell death via Ca2+-mediated mitochondrial damage and cytochrome c release (Kim et al., 2006) and Ca2+ influx may promote microglia hyperactivation. Microglia TRPV1 was found to be altered during microglial overactivation in a mouse model by using immunofluorescence sections Further, TRPV1 protein expression was significantly upregulated on microglia under stress, revealing that TRPV1 protein in microglia was involved in a rapid response process to stress. The observations suggest that TRPV1 channels are involved in regulating NADPH oxidase-mediated ROS production in microglia (Schilling and Eder, 2009). Stimulation of microglia TRPV1 can control cortical microglia activation and indirectly enhance glutamatergic transmission in neurons by promoting extracellular microvesicular shedding (Marrone et al., 2017). TRPV1 was found to control the expression of genes necessary for aerobic glycolysis and mitochondrial respiration to regulate microglial metabolic activities (Ding et al., 2021).
TRPV1 may also mediate neuroinflammation by regulating microglial activity. TRPV1 is activated in response to stimulation by changing the morphology and characteristics of microglial cells that monitor the neuronal network and the surroundings of the brain, clear away cellular debris, and aid in tissue healing. Inflammatory pathways and neuronal dysfunction may become self-sustaining and result in persistent neuroinflammation after triggering events if there is ineffective control. Further, M1 and M2 phenotypes can be formed from polarized microglial activation (Benson et al., 2015; Hu et al., 2015). While the M1 phenotype expresses pro-inflammatory cytokines and induces neuroinflammation, the M2 phenotype expresses anti-inflammatory cytokines, which inhibit neuroinflammation and repair tissue. By boosting the production and release of pro-inflammatory mediators, such as TNF, IL-1, IL-6, HMGB1, and ROS, activation of TRPV1 may directly impact the microglial pathophysiological response and shift microglia to an amoeboid-like morphology and M1 polarization state (Bok et al., 2018; Landucci et al., 2022). Relevant studies have shown that the dysregulation of microglial TRPV1 can lead to neuroinflammation and further aggravate neuronal damage. Microglial TRPV1 is located in the mitochondria, and activation of this channel induces mitochondrial depolarization, which then generates mtROS (activation of cell migration via mitogen-activated protein kinases or MAPK activation) (Marrone et al., 2017). Patients with temporal lobe epilepsy had higher levels of TRPV1 expression in their cortex and hippocampus, which could cause inflammation (Sun et al., 2013). TRPV1 was also implicated in the inflammation caused by microglia when capsaicin therapy increased bradykinin B1 receptor levels, which were linked to increased interleukin-1 (IL-1) and ROS generation as well as the redox-regulated NF-κB pathway in spinal microglia (Talbot et al., 2012). As a TRPV1 antagonist, Win-55, 212-2(WIN-2) decreased microglial activation and the production of proinflammatory cytokines while increasing the production of the anti-inflammatory cytokine IL-1α. However, it also activates cannabinoid receptors to increase hippocampal neurogenesis. The antagonist actions of WIN-2 at the TRPV1 receptor are responsible for the reduction in microglial activation, which indicates that TRPV1 is involved in hippocampal inflammation (Marchalant et al., 2009). In addition to the cannabinoid agonists WIN-55 and WIN-2, recent studies have shown that epoxides are also able to affect the activity of cannabinoid receptor 1 (CB1), and cannabinoid receptor 2 (CB2), and TRPV1 receptors. These epoxides raise the levels of the anti-inflammatory IL-10 cytokine in activated microglia and reduce proinflammatory indicators interleukins: IL-6, IL-1β, tumor necrosis factor-alpha (TNF-α), and NO. Activated microglia also themselves produce epoxides, and the presence of anandamide promotes this process. Epoxidase is activated by inflammation, which speeds up the metabolism of N-arachidonoyl-dopamine, N-arachidonoyl-serotonin, and other eCBs to produce the appropriate epoxides. Further, the anti-inflammatory drugs dopamine and 5-hydroxytryptamine can modulate cannabinoid receptors and TRPV1 channels by forming epoxides through the binding of epoxides to the endogenous cannabinoid eCB (Arnold et al., 2021).
Depending on the cytokine environment, TRPV1 modulation has pro- or anti-inflammatory effects. Additionally, TRPV1 antagonism can change the phenotype of microglia into an anti-inflammatory type. In addition, TRPV1 inhibitors alter calcium ion levels, which has neuroprotective effects. As expected, although many studies have shown proinflammatory effects of TRPV1, different experiments have shown a protective role of TRPV1 in several neurological disorders. In a rat model of ischemia-reperfusion injury, TRPV1 has been found to have advantageous neuroprotective effects as a crucial anti-inflammatory modulator in the process of neurological diseases. It was proposed that TRPV1 agonists may act as anti-inflammatory molecules and immunomodulators in the rat model of experimental autoimmune encephalomyelitis (EAE) by inhibiting the levels of cytokines, such as TNF-a, IL-1b, IL-12, IL17 and interferon (IFN) (Tsuji et al., 2010).
Role of microglia transient receptor potential vanilloid protein type 1 in epilepsy
Current studies also suggest that TRPV1 is involved in the secretion of inflammatory mediators and cytokines. There is strong proof that certain inflammatory mediators, such as cytokines, chemokines, neurotransmitters, peptides or small proteins, lipids, and growth factors, could sensitize TRPV1 (Wang et al., 2017). For instance, in primary cultured rodent microglia, TRPV1 is functionally expressed and is especially upregulated in activated microglia. Further, TNF-α is also induced in microglia. In the central terminals of DRG neurons, TNF-α may function as an endogenous activator of TRPV1 to trigger glutamate release. TNF-α induces spinal cord LTP by increasing sEPSC frequency through TRPV1 (Park et al., 2011). Subsequent to TRPV1 receptor activation, the expression levels of IL-1β, IL-6, TNF-α and HMGB1 were increased in wild-type mice with febrile convulsions in one report. The increase in proinflammatory factors aggravated brain inflammation and aggravated the occurrence of epilepsy (Huang et al., 2015). Further, capsaicin-based stimulation of TRPV1 in in vitro experiments greatly decreased the levels of TNF and IL-6 released by activated microglia. TRPV1 influenced the core inflammation by regulating the release of cytokines from activated microglia, which then affected the occurrence of epilepsy (Stampanoni Bassi et al., 2019). In mice, TRPV1 activation inhibits the neuroprotective impact of the interaction between microglial transforming growth factor-1 (TGF-1) and Toll-like receptor 4 (TLR4), promoting microglia activation and indirectly increasing epilepsy susceptibility. TRPV1 gene deletion, on the other hand, prevented aberrant microglial activation brought on by lipopolysaccharide or hyperthermia and reestablished a balanced inflammatory microenvironment in the brain (Kong et al., 2019). In addition, a surplus calcium influx causes depolarization of nerve endings to generate action potentials and releases calcitonin gene-related peptide (CGRP) and substance P (SP), which are involved in various physiological and pathological activities of the body. Further, TRPV1 activation induces an increase in intracellular calcium concentration, which in turn stimulates NO production by nitric oxide synthase, leading to increased cellular excitability (Bhandari et al., 2021). In addition, calcitonin gene-related peptide (CGRP) upregulation activates the endothelial nitric oxide synthase and nitric oxide signaling pathways, resulting in the inhibition of inflammatory responses, and thus lowers neuronal damage. It has also been shown that the excessive activation of TRPV1 channels can reduce the release of neurotrophic factor, aggravate the inflammatory response, and affect nerve regeneration and repair after injury (Hsu et al., 2017). Conversely, the inward flux of calcium ions causes changes in microtubule disassembly and F-actin reorganization, which in turn promotes cell migration. Endoplasmic reticulum (ER) stress and calcium overload are also caused by TRPV1 activation. These conditions then cause the opening of mitochondrial permeability transition pores and the production of ROS, which results in death (Naziroglu et al., 2019). During the development of epilepsy, microglia are activated to release TNFα, which drives the release of astrocyte ATP/ADP, with such astrocyte purinergic signaling playing an important role in the development of epilepsy (Bedner and Steinhauser, 2019). IL-10 produced by microglia stimulates astrocytes to produce TGFβ, which thereby attenuates microglia activation and provides negative feedback to microglia activation and reduces the neuroinflammatory response in the brain (Norden et al., 2014). In addition, astrocytes may regulate microglial cytokine release in status epilepticus through an ATP-dependent mechanism (Bianco et al., 2005). Further, TRPV1 assisted astrocyte migration, allowing pro-inflammatory cytokines such as TNF, IL-1, IL-6, and iNOS to permeate the area around neurons and cause seizures (Wang et al., 2019). The activation of TRPV1 on microglia membranes and on microglial organellar membranes increases calcium ion concentration, which leads to the increased presynaptic release of glutamate. This acts not only on neurons but also on postsynaptic neurons as well as astrocytes. In addition, malfunctioning of glutamate transporters and the astrocytic glutamate-converting enzyme, glutamine synthetase has been observed in epileptic tissue (Eid et al., 2004). In the kainic acid-induced status epilepticus model of temporal lobe epilepsy (TLE), astrocytes mediated glutamate uptake (Takahashi et al., 2010). Another study reported that reduced monocarboxylate transporter 4 (MCT4) and excitatory amino acid transporter 1 (EAAT1) expression in astrocytes may lead to neuronal hyperexcitability and epileptogenesis in the temporal lobe epileptic foci excised from patients with intractable epilepsy (Liu et al., 2014). An increase in extracellular glutamate may also precipitate seizures (During and Spencer, 1993), where glutamine synthetase (GS) converts glutamate to glutamine. In the chronic epileptic hippocampus, loss of GS-impaired extracellular glutamate clearance and glutamine supply to neurons results in decreased GABA (Fricke et al., 2007; Yang and Cox, 2011).
To summarize, these findings strongly demonstrate the fact that TRPV1 is expressed in microglia and is crucial for glial activation. It is also involved in epilepsy caused by inflammation (Fig. 2).
TRPV1 Acts as a Potential Therapeutic Target for Epilepsy
TRPV1 is now being studied in the laboratory and is being viewed as a potential target molecule for epilepsy treatment in the future (Naziroglu, 2015). CBD is the only TRPV1 medication currently being used clinically to treat epilepsy. Further, CBD has been approved by the US Food and Drug Administration (FDA) for the treatment of seizures associated with Dravet syndrome, Lennox–Gastaut syndrome, and tuberous sclerosis complex. In the European Union, the drug has been authorized by the European Medicines Agency for the treatment of seizures associated with Dravet syndrome and Lennox–Gastaut syndrome, in conjunction with clobazam. Further, it is under regulatory review for the treatment of seizures in patients with tuberous sclerosis complex (Lattanzi et al., 2021).
In conclusion, the study of the role of TRPV1 in microglia in epilepsy is a meaningful and promising area of research. Clinical studies on epilepsy are now examining a variety of TRPV1 agonists and antagonists. More research is still needed to fully understand the therapeutic potential and regulatory mechanisms of TRPV1 in microglia. The majority of the evidence for the widespread expression of TRPV1 in the brain comes from research employing neonatal and adult rat models of epilepsy, pain, and other inflammatory diseases. Rodent studies have similarly demonstrated that TRPV1 improves the CNS response to inflammatory stress. The activity, transport, and expression of TRPV1 are also regulated by inflammatory mediators. The increase in TRPV1 in microglia following epilepsy has been shown by various studies that have examined the impact of inflammatory mediators, cytokines, and oxidative stress on microglia TRPV1 expression in epilepsy models and patients. TRPV1 plays a crucial role in the control of synaptic transmission and its plasticity, as well as in the communication between microglia and neurons and the process of development. Thus, the impact of TRPV1 in microglia and on the onset and progression of epilepsy cannot be ignored.
There is a need for a better understanding of the role of TRPV1 in neurogenic inflammatory pathways in microglia and its role in inflammatory injury-induced epilepsy. The results will provide clinicians with valuable insights and provide them with new therapeutic targets and improved approaches for treating epilepsy. The pharmaceutical industry has not yet properly acknowledged the TRPV1 channel as a viable new drug target. Future research should focus on TRPV1 channel inhibitors as potential novel antiepileptic medications.
Funding Statement: The authors received no specific funding for this study.
Author Contributions: The authors confirm contribution to the paper as follows: study conception and manuscript revision: Jiao Hu; draft manuscript preparation: Jiao Hu, Jialu Mo, Xianglin Cheng. Both authors reviewed the manuscript and approved the final version of the manuscript.
Ethics Approval: Not applicable.
Conflicts of Interest: The authors declare that they have no conflicts of interest to report regarding the present study.
References
Abdel-Salam OME, Sleem AA, Sayed M, Youness ER, Shaffie N (2020). Correction to: Capsaicin exerts anti-convulsant and neuroprotective effects in pentylenetetrazole-induced seizures. Neurochemical Research 45: 1062–1063. https://doi.org/10.1007/s11064-020-02995-3 [Google Scholar] [PubMed] [CrossRef]
Adams B, Sazgar M, Osehobo P, van der Zee CE, Diamond J, Fahnestock M, Racine RJ (1997). Nerve growth factor accelerates seizure development, enhances mossy fiber sprouting, and attenuates seizure-induced decreases in neuronal density in the kindling model of epilepsy. The Journal of Neuroscience 17: 5288–5296. https://doi.org/10.1523/JNEUROSCI.17-14-05288.1997 [Google Scholar] [PubMed] [CrossRef]
Andoh M, Ikegaya Y, Koyama R (2020). Microglia modulate the structure and function of the hippocampus after early-life seizures. Journal of Pharmacological Sciences 144: 212–217. https://doi.org/10.1016/j.jphs.2020.09.003 [Google Scholar] [PubMed] [CrossRef]
Arnold WR, Carnevale LN, Xie Z, Baylon JL, Tajkhorshid E, Hu H, Das A (2021). Anti-inflammatory dopamine- and serotonin-based endocannabinoid epoxides reciprocally regulate cannabinoid receptors and the TRPV1 channel. Nature Communications 12: 926. https://doi.org/10.1038/s41467-021-20946-6 [Google Scholar] [PubMed] [CrossRef]
Badimon A, Strasburger HJ, Ayata P, Chen X, Nair A et al. (2020). Negative feedback control of neuronal activity by microglia. Nature 586: 417–423. https://doi.org/10.1038/s41586-020-2777-8 [Google Scholar] [PubMed] [CrossRef]
Barrett KT, Wilson RJ, Scantlebury MH (2016). TRPV1 deletion exacerbates hyperthermic seizures in an age-dependent manner in mice. Epilepsy Research 128: 27–34. https://doi.org/10.1016/j.eplepsyres.2016.10.016 [Google Scholar] [PubMed] [CrossRef]
Bear MF, Malenka RC (1994). Synaptic plasticity: LTP and LTD. Current Opinion in Neurobiology 4: 389–399. https://doi.org/10.1016/0959-4388(94)90101-5 [Google Scholar] [PubMed] [CrossRef]
Bedner P, Steinhauser C (2019). TNFα-driven astrocyte purinergic signaling during epileptogenesis. Trends in Molecular Medicine 25: 70–72. https://doi.org/10.1016/j.molmed.2018.12.001 [Google Scholar] [PubMed] [CrossRef]
Benson MJ, Manzanero S, Borges K (2015). Complex alterations in microglial M1/M2 markers during the development of epilepsy in two mouse models. Epilepsia 56: 895–905. https://doi.org/10.1111/epi.12960 [Google Scholar] [PubMed] [CrossRef]
Bhandari R, Gupta R, Vashishth A, Kuhad A (2021). Transient Receptor Potential Vanilloid 1 (TRPV1) as a plausible novel therapeutic target for treating neurological complications in ZikaVirus. Medical Hypotheses 156: 110685. https://doi.org/10.1016/j.mehy.2021.110685 [Google Scholar] [PubMed] [CrossRef]
Bhaskaran MD, Smith BN (2010). Effects of TRPV1 activation on synaptic excitation in the dentate gyrus of a mouse model of temporal lobe epilepsy. Experimental Neurology 223: 529–536. https://doi.org/10.1016/j.expneurol.2010.01.021 [Google Scholar] [PubMed] [CrossRef]
Bianco F, Pravettoni E, Colombo A, Schenk U, Moller T, Matteoli M, Verderio C (2005). Astrocyte-derived ATP induces vesicle shedding and IL-1 beta release from microglia. Journal of Immunology 174: 7268–7277. https://doi.org/10.4049/jimmunol.174.11.7268 [Google Scholar] [PubMed] [CrossRef]
Bok E, Chung YC, Kim KS, Baik HH, Shin WH, Jin BK (2018). Modulation of M1/M2 polarization by capsaicin contributes to the survival of dopaminergic neurons in the lipopolysaccharide-lesioned substantia nigra in vivo. Experimental & Molecular Medicine 50: 1–14. https://doi.org/10.1038/s12276-018-0111-4 [Google Scholar] [PubMed] [CrossRef]
Cao E, Liao M, Cheng Y, Julius D (2013). TRPV1 structures in distinct conformations reveal activation mechanisms. Nature 504: 113–118. https://doi.org/10.1038/nature12823 [Google Scholar] [PubMed] [CrossRef]
Caterina MJ, Schumacher MA, Tominaga M, Rosen TA, Levine JD, Julius D (1997). The capsaicin receptor: A heat-activated ion channel in the pain pathway. Nature 389: 816–824. https://doi.org/10.1038/39807 [Google Scholar] [PubMed] [CrossRef]
Cavanaugh DJ, Chesler AT, Jackson AC, Sigal YM, Yamanaka H et al. (2011). Trpv1 reporter mice reveal highly restricted brain distribution and functional expression in arteriolar smooth muscle cells. The Journal of Neuroscience 31: 5067–5077. https://doi.org/10.1523/JNEUROSCI.6451-10.2011 [Google Scholar] [PubMed] [CrossRef]
Cendes F, Cook MJ, Watson C, Andermann F, Fish DR et al. (1995). Frequency and characteristics of dual pathology in patients with lesional epilepsy. Neurology 45: 2058–2064. https://doi.org/10.1212/WNL.45.11.2058 [Google Scholar] [PubMed] [CrossRef]
Chavez AE, Chiu CQ, Castillo PE (2010). TRPV1 activation by endogenous anandamide triggers postsynaptic long-term depression in dentate gyrus. Nature Neuroscience 13: 1511–1518. https://doi.org/10.1038/nn.2684 [Google Scholar] [PubMed] [CrossRef]
Chavez AE, Hernandez VM, Rodenas-Ruano A, Chan CS, Castillo PE (2014). Compartment-specific modulation of GABAergic synaptic transmission by TRPV1 channels in the dentate gyrus. The Journal of Neuroscience 34: 16621–16629. https://doi.org/10.1523/JNEUROSCI.3635-14.2014 [Google Scholar] [PubMed] [CrossRef]
Chuang HH, Prescott ED, Kong H, Shields S, Jordt SE et al. (2001). Bradykinin and nerve growth factor release the capsaicin receptor from PtdIns(4,5)P2-mediated inhibition. Nature 411: 957–962. https://doi.org/10.1038/35082088 [Google Scholar] [PubMed] [CrossRef]
Cunningham CL, Martinez-Cerdeno V, Noctor SC (2013). Microglia regulate the number of neural precursor cells in the developing cerebral cortex. The Journal of Neuroscience 33: 4216–4233. https://doi.org/10.1523/JNEUROSCI.3441-12.2013 [Google Scholar] [PubMed] [CrossRef]
Devinsky O, Vezzani A, Najjar S, de Lanerolle NC, Rogawski MA (2013). Glia and epilepsy: Excitability and inflammation. Trends in Neurosciences 36: 174–184. https://doi.org/10.1016/j.tins.2012.11.008 [Google Scholar] [PubMed] [CrossRef]
Ding D, Zhou D, Sander JW, Wang W, Li S, Hong Z (2021). Epilepsy in China: Major progress in the past two decades. The Lancet Neurology 20: 316–326. https://doi.org/10.1016/S1474-4422(21)00023-5 [Google Scholar] [PubMed] [CrossRef]
Doyle MW, Bailey TW, Jin YH, Andresen MC (2002). Vanilloid receptors presynaptically modulate cranial visceral afferent synaptic transmission in nucleus tractus solitarius. The Journal of Neuroscience 22: 8222–8229. https://doi.org/10.1523/JNEUROSCI.22-18-08222.2002 [Google Scholar] [PubMed] [CrossRef]
During MJ, Spencer DD (1993). Extracellular hippocampal glutamate and spontaneous seizure in the conscious human brain. The Lancet 341: 1607–1610. https://doi.org/10.1016/0140-6736(93)90754-5 [Google Scholar] [PubMed] [CrossRef]
Eid T, Thomas MJ, Spencer DD, Runden-Pran E, Lai JC et al. (2004). Loss of glutamine synthetase in the human epileptogenic hippocampus: Possible mechanism for raised extracellular glutamate in mesial temporal lobe epilepsy. The Lancet 363: 28–37. https://doi.org/10.1016/S0140-6736(03)15166-5 [Google Scholar] [PubMed] [CrossRef]
Eyo UB, Peng J, Murugan M, Mo M, Lalani A et al. (2016). Regulation of physical microglia-neuron interactions by fractalkine signaling after status epilepticus. eNeuro 3. https://doi.org/10.1523/ENEURO.0209-16.2016 [Google Scholar] [PubMed] [CrossRef]
Fricke MN, Jones-Davis DM, Mathews GC (2007). Glutamine uptake by System A transporters maintains neurotransmitter GABA synthesis and inhibitory synaptic transmission. Journal of Neurochemistry 102: 1895–1904. https://doi.org/10.1111/j.1471-4159.2007.04649.x [Google Scholar] [PubMed] [CrossRef]
Gibson HE, Edwards JG, Page RS, van Hook MJ, Kauer JA (2008). TRPV1 channels mediate long-term depression at synapses on hippocampal interneurons. Neuron 57: 746–759. https://doi.org/10.1016/j.neuron.2007.12.027 [Google Scholar] [PubMed] [CrossRef]
Gonzalez-Reyes LE, Ladas TP, Chiang CC, Durand DM (2013). TRPV1 antagonist capsazepine suppresses 4-AP-induced epileptiform activity in vitro and electrographic seizures in vivo. Experimental Neurology 250: 321–332. https://doi.org/10.1016/j.expneurol.2013.10.010 [Google Scholar] [PubMed] [CrossRef]
Gray RA, Whalley BJ (2020). The proposed mechanisms of action of CBD in epilepsy. Epileptic Disorders 22: 10–15. https://doi.org/10.1684/epd.2020.1135 [Google Scholar] [PubMed] [CrossRef]
Grueter BA, Brasnjo G, Malenka RC (2010). Postsynaptic TRPV1 triggers cell type-specific long-term depression in the nucleus accumbens. Nature Neuroscience 13: 1519–1525. https://doi.org/10.1038/nn.2685 [Google Scholar] [PubMed] [CrossRef]
Han Q, Lin Q, Huang P, Chen M, Hu X et al. (2017). Microglia-derived IL-1β contributes to axon development disorders and synaptic deficit through p38-MAPK signal pathway in septic neonatal rats. Journal of Neuroinflammation 14: 52. https://doi.org/10.1186/s12974-017-0805-x [Google Scholar] [PubMed] [CrossRef]
Hsu ST, Yao CH, Hsu YM, Lin JH, Chen YH, Chen YS (2017). Effects of taxol on regeneration in a rat sciatic nerve transection model. Scientific Reports 7: 42280. https://doi.org/10.1038/srep42280 [Google Scholar] [PubMed] [CrossRef]
Hu X, Leak RK, Shi Y, Suenaga J, Gao Y, Zheng P, Chen J (2015). Microglial and macrophage polarization-new prospects for brain repair. Nature Reviews Neurology 11: 56–64. https://doi.org/10.1038/nrneurol.2014.207 [Google Scholar] [PubMed] [CrossRef]
Huang WX, Yu F, Sanchez RM, Liu YQ, Min JW et al. (2015). TRPV1 promotes repetitive febrile seizures by pro-inflammatory cytokines in immature brain. Brain, Behavior, and Immunity 48: 68–77. https://doi.org/10.1016/j.bbi.2015.01.017 [Google Scholar] [PubMed] [CrossRef]
Hurtado-Zavala JI, Ramachandran B, Ahmed S, Halder R, Bolleyer C et al. (2017). TRPV1 regulates excitatory innervation of OLM neurons in the hippocampus. Nature Communications 8: 15878. https://doi.org/10.1038/ncomms15878 [Google Scholar] [PubMed] [CrossRef]
Iannotti FA, Hill CL, Leo A, Alhusaini A, Soubrane C et al. (2014). Nonpsychotropic plant cannabinoids, cannabidivarin (CBDV) and cannabidiol (CBDactivate and desensitize transient receptor potential vanilloid 1 (TRPV1) channels in vitro: Potential for the treatment of neuronal hyperexcitability. ACS Chemical Neuroscience 5: 1131–1141. https://doi.org/10.1021/cn5000524 [Google Scholar] [PubMed] [CrossRef]
Ivanova B, Spiteller M (2014). Evodiamine and rutaecarpine alkaloids as highly selective transient receptor potential vanilloid 1 agonists. International Journal of Biological Macromolecules 65: 314–324. https://doi.org/10.1016/j.ijbiomac.2014.01.059 [Google Scholar] [PubMed] [CrossRef]
Jiang J, Yu Y (2023). Pharmacologically targeting transient receptor potential channels for seizures and epilepsy: Emerging preclinical evidence of druggability. Pharmacology & Therapeutics 244: 108384. https://doi.org/10.1016/j.pharmthera.2023.108384 [Google Scholar] [PubMed] [CrossRef]
Kim SH, Hadley SH, Maddison M, Patil M, Cha B, Kollarik M, Taylor-Clark TE (2020). Mapping of sensory nerve subsets within the vagal ganglia and the brainstem using reporter mice for pirt. eNeuro 7. https://doi.org/10.1523/ENEURO.0494-19.2020 [Google Scholar] [PubMed] [CrossRef]
Kim SR, Kim SU, Oh U, Jin BK (2006). Transient receptor potential vanilloid subtype 1 mediates microglial cell death in vivo and in vitro via Ca2+-mediated mitochondrial damage and cytochrome c release. Journal of Immunology 177: 4322–4329. https://doi.org/10.4049/jimmunol.177.7.4322 [Google Scholar] [PubMed] [CrossRef]
Kong WL, Min JW, Liu YL, Li JX, He XH, Peng BW (2014). Role of TRPV1 in susceptibility to PTZ-induced seizure following repeated hyperthermia challenges in neonatal mice. Epilepsy & Behavior 31: 276–280. https://doi.org/10.1016/j.yebeh.2013.10.022 [Google Scholar] [PubMed] [CrossRef]
Kong W, Wang X, Yang X, Huang W, Han S et al. (2019). Activation of TRPV1 contributes to recurrent febrile seizures via inhibiting the microglial M2 phenotype in the immature brain. Frontiers in Cellular Neuroscience 13: 442. https://doi.org/10.3389/fncel.2019.00442 [Google Scholar] [PubMed] [CrossRef]
Koplas PA, Rosenberg RL, Oxford GS (1997). The role of calcium in the desensitization of capsaicin responses in rat dorsal root ganglion neurons. The Journal of Neuroscience 17: 3525–3537. https://doi.org/10.1523/JNEUROSCI.17-10-03525.1997 [Google Scholar] [PubMed] [CrossRef]
Landucci E, Mazzantini C, Lana D, Calvani M, Magni G, Giovannini MG, Pellegrini-Giampietro DE (2022). Cannabidiol inhibits microglia activation and mitigates neuronal damage induced by kainate in an in-vitro seizure model. Neurobiology of Disease 174: 105895. https://doi.org/10.1016/j.nbd.2022.105895 [Google Scholar] [PubMed] [CrossRef]
Lattanzi S, Trinka E, Striano P, Rocchi C, Salvemini S, Silvestrini M, Brigo F (2021). Highly purified cannabidiol for epilepsy treatment: A systematic review of epileptic conditions beyond dravet syndrome and lennox-gastaut syndrome. CNS Drugs 35: 265–281. https://doi.org/10.1007/s40263-021-00807-y [Google Scholar] [PubMed] [CrossRef]
Lawson LJ, Perry VH, Dri P, Gordon S (1990). Heterogeneity in the distribution and morphology of microglia in the normal adult mouse brain. Neuroscience 39: 151–170. https://doi.org/10.1016/0306-4522(90)90229-W [Google Scholar] [PubMed] [CrossRef]
Lee SH, Ledri M, Toth B, Marchionni I, Henstridge CM et al. (2015). Multiple forms of endocannabinoid and endovanilloid signaling regulate the tonic control of GABA release. The Journal of Neuroscience 35: 10039–10057. https://doi.org/10.1523/JNEUROSCI.4112-14.2015 [Google Scholar] [PubMed] [CrossRef]
Lee TH, Lee JG, Yon JM, Oh KW, Baek IJ et al. (2011). Capsaicin prevents kainic acid-induced epileptogenesis in mice. Neurochemistry International 58: 634–640. https://doi.org/10.1016/j.neuint.2011.01.027 [Google Scholar] [PubMed] [CrossRef]
Lehrman EK, Wilton DK, Litvina EY, Welsh CA, Chang ST et al. (2018). CD47 protects synapses from excess microglia-mediated pruning during development. Neuron 100: 120–134 e126. https://doi.org/10.1016/j.neuron.2018.09.017 [Google Scholar] [PubMed] [CrossRef]
Leite JP, Neder L, Arisi GM, Carlotti Jr CG, Assirati JA, Moreira JE (2005). Plasticity, synaptic strength, and epilepsy: What can we learn from ultrastructural data? Epilepsia 46: 134–141. https://doi.org/10.1111/j.1528-1167.2005.01021.x [Google Scholar] [PubMed] [CrossRef]
Li Y, Du XF, Liu CS, Wen ZL, Du JL (2012). Reciprocal regulation between resting microglial dynamics and neuronal activity in vivo. Developmental Cell 23: 1189–1202. https://doi.org/10.1016/j.devcel.2012.10.027 [Google Scholar] [PubMed] [CrossRef]
Li HB, Mao RR, Zhang JC, Yang Y, Cao J, Xu L (2008). Antistress effect of TRPV1 channel on synaptic plasticity and spatial memory. Biological Psychiatry 64: 286–292. https://doi.org/10.1016/j.biopsych.2008.02.020 [Google Scholar] [PubMed] [CrossRef]
Liao M, Cao E, Julius D, Cheng Y (2013). Structure of the TRPV1 ion channel determined by electron cryo-microscopy. Nature 504: 107–112. https://doi.org/10.1038/nature12822 [Google Scholar] [PubMed] [CrossRef]
Liu B, Niu L, Shen MZ, Gao L, Wang C et al. (2014). Decreased astroglial monocarboxylate transporter 4 expression in temporal lobe epilepsy. Molecular Neurobiology 50: 327–338. https://doi.org/10.1007/s12035-013-8619-z [Google Scholar] [PubMed] [CrossRef]
Luo C, Koyama R, Ikegaya Y (2016). Microglia engulf viable newborn cells in the epileptic dentate gyrus. Glia 64: 1508–1517. https://doi.org/10.1002/glia.23018 [Google Scholar] [PubMed] [CrossRef]
Marchalant Y, Brothers HM, Norman GJ, Karelina K, DeVries AC, Wenk GL (2009). Cannabinoids attenuate the effects of aging upon neuroinflammation and neurogenesis. Neurobiology of Disease 34: 300–307. https://doi.org/10.1016/j.nbd.2009.01.014 [Google Scholar] [PubMed] [CrossRef]
Marinelli S, Di Marzo V, Berretta N, Matias I, Maccarrone M, Bernardi G, Mercuri NB (2003). Presynaptic facilitation of glutamatergic synapses to dopaminergic neurons of the rat substantia nigra by endogenous stimulation of vanilloid receptors. The Journal of Neuroscience 23: 3136–3144. https://doi.org/10.1523/JNEUROSCI.23-08-03136.2003 [Google Scholar] [PubMed] [CrossRef]
Marinelli S, Vaughan CW, Christie MJ, Connor M (2002). Capsaicin activation of glutamatergic synaptic transmission in the rat locus coeruleus in vitro. The Journal of Physiology 543: 531–540. https://doi.org/10.1113/jphysiol.2002.022863 [Google Scholar] [PubMed] [CrossRef]
Marrone MC, Morabito A, Giustizieri M, Chiurchiu V, Leuti A et al. (2017). TRPV1 channels are critical brain inflammation detectors and neuropathic pain biomarkers in mice. Nature Communications 8: 15292. https://doi.org/10.1038/ncomms15292 [Google Scholar] [PubMed] [CrossRef]
Marshall IC, Owen DE, Cripps TV, Davis JB, McNulty S, Smart D (2003). Activation of vanilloid receptor 1 by resiniferatoxin mobilizes calcium from inositol 1,4,5-trisphosphate-sensitive stores. British Journal of Pharmacology 138: 172–176. https://doi.org/10.1038/sj.bjp.0705003 [Google Scholar] [PubMed] [CrossRef]
Menigoz A, Boudes M (2011). The expression pattern of TRPV1 in brain. The Journal of Neuroscience 31: 13025–13027. https://doi.org/10.1523/JNEUROSCI.2589-11.2011 [Google Scholar] [PubMed] [CrossRef]
Miyake T, Shirakawa H, Nakagawa T, Kaneko S (2015). Activation of mitochondrial transient receptor potential vanilloid 1 channel contributes to microglial migration. Glia 63: 1870–1882. https://doi.org/10.1002/glia.22854 [Google Scholar] [PubMed] [CrossRef]
Miyamoto A, Wake H, Ishikawa AW, Eto K, Shibata K et al. (2016). Microglia contact induces synapse formation in developing somatosensory cortex. Nature Communications 7: 12540. https://doi.org/10.1038/ncomms12540 [Google Scholar] [PubMed] [CrossRef]
Nasrallah K, Frechou MA, Yoon YJ, Persaud S, Goncalves JT, Castillo PE (2022). Seizure-induced strengthening of a recurrent excitatory circuit in the dentate gyrus is proconvulsant. Proceedings of the National Academy of Sciences of the United States of America 119: e2201151119. https://doi.org/10.1073/pnas.2201151119 [Google Scholar] [PubMed] [CrossRef]
Naziroglu M (2015). TRPV1 channel: A potential drug target for treating epilepsy. Current Neuropharmacology 13: 239–247. https://doi.org/10.2174/1570159X13666150216222543 [Google Scholar] [PubMed] [CrossRef]
Naziroglu M, Ovey IS (2015). Involvement of apoptosis and calcium accumulation through TRPV1 channels in neurobiology of epilepsy. Neuroscience 293: 55–66. https://doi.org/10.1016/j.neuroscience.2015.02.041 [Google Scholar] [PubMed] [CrossRef]
Naziroglu M, Ozkan FF, Hapil SR, Ghazizadeh V, Cig B (2015). Epilepsy but not mobile phone frequency (900 MHz) induces apoptosis and calcium entry in hippocampus of epileptic rat: Involvement of TRPV1 channels. The Journal of Membrane Biology 248: 83–91. https://doi.org/10.1007/s00232-014-9744-y [Google Scholar] [PubMed] [CrossRef]
Naziroglu M, Taner AN, Balbay E, Cig B (2019). Inhibitions of anandamide transport and FAAH synthesis decrease apoptosis and oxidative stress through inhibition of TRPV1 channel in an in vitro seizure model. Molecular and Cellular Biochemistry 453: 143–155. https://doi.org/10.1007/s11010-018-3439-0 [Google Scholar] [PubMed] [CrossRef]
Neacsu C, Babes A (2010). The M-channel blocker linopirdine is an agonist of the capsaicin receptor TRPV1. Journal of Pharmacological Sciences 114: 332–340. https://doi.org/10.1254/jphs.10172FP [Google Scholar] [PubMed] [CrossRef]
Noda M, Nakanishi H, Nabekura J, Akaike N (2000). AMPA-kainate subtypes of glutamate receptor in rat cerebral microglia. The Journal of Neuroscience 20: 251–258. https://doi.org/10.1523/JNEUROSCI.20-01-00251.2000 [Google Scholar] [PubMed] [CrossRef]
Norden DM, Fenn AM, Dugan A, Godbout JP (2014). TGFβ produced by IL-10 redirected astrocytes attenuates microglial activation. Glia 62: 881–895. https://doi.org/10.1002/glia.22647 [Google Scholar] [PubMed] [CrossRef]
Olowe R, Sandouka S, Saadi A, Shekh-Ahmad T (2020). Approaches for reactive oxygen species and oxidative stress quantification in epilepsy. Antioxidants 9: 990. https://doi.org/10.3390/antiox9100990 [Google Scholar] [PubMed] [CrossRef]
Park CK, Lu N, Xu ZZ, Liu T, Serhan CN, Ji RR (2011). Resolving TRPV1- and TNF-α-mediated spinal cord synaptic plasticity and inflammatory pain with neuroprotectin D1. The Journal of Neuroscience 31: 15072–15085. https://doi.org/10.1523/JNEUROSCI.2443-11.2011 [Google Scholar] [PubMed] [CrossRef]
Parkhurst CN, Yang G, Ninan I, Savas JN, Yates JR et al. (2013). Microglia promote learning-dependent synapse formation through brain-derived neurotrophic factor. Cell 155: 1596–1609. https://doi.org/10.1016/j.cell.2013.11.030 [Google Scholar] [PubMed] [CrossRef]
Pasierski M, Szulczyk B (2022). Beneficial effects of capsaicin in disorders of the central nervous system. Molecules 27: 2484. https://doi.org/10.3390/molecules27082484 [Google Scholar] [PubMed] [CrossRef]
Qin L, Wu X, Block ML, Liu Y, Breese GR et al. (2007). Systemic LPS causes chronic neuroinflammation and progressive neurodegeneration. Glia 55: 453–462. https://doi.org/10.1002/(ISSN)1098-1136 [Google Scholar] [CrossRef]
Rice RA, Spangenberg EE, Yamate-Morgan H, Lee RJ, Arora RP et al. (2015). Elimination of microglia improves functional outcomes following extensive neuronal loss in the hippocampus. The Journal of Neuroscience 35: 9977–9989. https://doi.org/10.1523/JNEUROSCI.0336-15.2015 [Google Scholar] [PubMed] [CrossRef]
Roumier A, Bechade C, Poncer JC, Smalla KH, Tomasello E et al. (2004). Impaired synaptic function in the microglial KARAP/DAP12-deficient mouse. The Journal of Neuroscience 24: 11421–11428. https://doi.org/10.1523/JNEUROSCI.2251-04.2004 [Google Scholar] [PubMed] [CrossRef]
Saffarzadeh F, Eslamizade MJ, Ghadiri T, Modarres Mousavi SM, Hadjighassem M, Gorji A (2015). Effects of TRPV1 on the hippocampal synaptic plasticity in the epileptic rat brain. Synapse 69: 375–383. https://doi.org/10.1002/syn.21825 [Google Scholar] [PubMed] [CrossRef]
Sappington RM, Calkins DJ (2008). Contribution of TRPV1 to microglia-derived IL-6 and NFκB translocation with elevated hydrostatic pressure. Investigative Ophthalmology & Visual Science 49: 3004–3017. https://doi.org/10.1167/iovs.07-1355 [Google Scholar] [PubMed] [CrossRef]
Scheffer IE, Berkovic S, Capovilla G, Connolly MB, French J et al. (2017). ILAE classification of the epilepsies: Position paper of the ILAE commission for classification and terminology. Epilepsia 58: 512–521. https://doi.org/10.1111/epi.13709 [Google Scholar] [PubMed] [CrossRef]
Schilling T, Eder C (2009). Importance of the non-selective cation channel TRPV1 for microglial reactive oxygen species generation. Journal of Neuroimmunology 216: 118–121. https://doi.org/10.1016/j.jneuroim.2009.07.008 [Google Scholar] [PubMed] [CrossRef]
Sekar A, Bialas AR, de Rivera H, Davis A, Hammond TR et al. (2022). Author correction: Schizophrenia risk from complex variation of complement component 4. Nature 601: E4–E5. https://doi.org/10.1038/s41586-021-04202-x [Google Scholar] [PubMed] [CrossRef]
Shoudai K, Peters JH, McDougall SJ, Fawley JA, Andresen MC (2010). Thermally active TRPV1 tonically drives central spontaneous glutamate release. The Journal of Neuroscience 30: 14470–14475. https://doi.org/10.1523/JNEUROSCI.2557-10.2010 [Google Scholar] [PubMed] [CrossRef]
Socala K, Nieoczym D, Pierog M, Wlaz P (2015). α-Spinasterol, a TRPV1 receptor antagonist, elevates the seizure threshold in three acute seizure tests in mice. Journal of Neural Transmission 122: 1239–1247. https://doi.org/10.1007/s00702-015-1391-7 [Google Scholar] [PubMed] [CrossRef]
Song MY, Tian FF, Wang YZ, Huang X, Guo JL, Ding DX (2015). Potential roles of the RGMa-FAK-Ras pathway in hippocampal mossy fiber sprouting in the pentylenetetrazole kindling model. Molecular Medicine Reports 11: 1738–1744. https://doi.org/10.3892/mmr.2014.2993 [Google Scholar] [PubMed] [CrossRef]
Squarzoni P, Oller G, Hoeffel G, Pont-Lezica L, Rostaing P et al. (2014). Microglia modulate wiring of the embryonic forebrain. Cell Reports 8: 1271–1279. https://doi.org/10.1016/j.celrep.2014.07.042 [Google Scholar] [PubMed] [CrossRef]
Stampanoni Bassi M, Gentile A, Iezzi E, Zagaglia S, Musella A et al. (2019). Transient receptor potential vanilloid 1 modulates central inflammation in multiple sclerosis. Frontiers in Neurology 10: 30. https://doi.org/10.3389/fneur.2019.00030 [Google Scholar] [PubMed] [CrossRef]
Stevens B, Allen NJ, Vazquez LE, Howell GR, Christopherson KS et al. (2007). The classical complement cascade mediates CNS synapse elimination. Cell 131: 1164–1178. https://doi.org/10.1016/j.cell.2007.10.036 [Google Scholar] [PubMed] [CrossRef]
Sun FJ, Guo W, Zheng DH, Zhang CQ, Li S et al. (2013). Increased expression of TRPV1 in the cortex and hippocampus from patients with mesial temporal lobe epilepsy. Journal of Molecular Neuroscience 49: 182–193. https://doi.org/10.1007/s12031-012-9878-2 [Google Scholar] [PubMed] [CrossRef]
Svahn AJ, Graeber MB, Ellett F, Lieschke GJ, Rinkwitz S, Bennett MR, Becker TS (2013). Development of ramified microglia from early macrophages in the zebrafish optic tectum. Developmental Neurobiology 73: 60–71. https://doi.org/10.1002/dneu.22039 [Google Scholar] [PubMed] [CrossRef]
Szallasi A, Cortright DN, Blum CA, Eid SR (2007). The vanilloid receptor TRPV1: 10 years from channel cloning to antagonist proof-of-concept. Nature Reviews Drug Discovery 6: 357–372. https://doi.org/10.1038/nrd2280 [Google Scholar] [PubMed] [CrossRef]
Takahashi DK, Vargas JR, Wilcox KS (2010). Increased coupling and altered glutamate transport currents in astrocytes following kainic-acid-induced status epilepticus. Neurobiology of Disease 40: 573–585. https://doi.org/10.1016/j.nbd.2010.07.018 [Google Scholar] [PubMed] [CrossRef]
Talbot S, Dias JP, Lahjouji K, Bogo MR, Campos MM, Gaudreau P, Couture R (2012). Activation of TRPV1 by capsaicin induces functional kinin B1 receptor in rat spinal cord microglia. Journal of Neuroinflammation 9: 16. https://doi.org/10.1186/1742-2094-9-16 [Google Scholar] [PubMed] [CrossRef]
Tominaga M, Caterina MJ, Malmberg AB, Rosen TA, Gilbert H et al. (1998). The cloned capsaicin receptor integrates multiple pain-producing stimuli. Neuron 21: 531–543. https://doi.org/10.1016/S0896-6273(00)80564-4 [Google Scholar] [PubMed] [CrossRef]
Toth A, Boczan J, Kedei N, Lizanecz E, Bagi Z et al. (2005). Expression and distribution of vanilloid receptor 1 (TRPV1) in the adult rat brain. Molecular Brain Research 135: 162–168. https://doi.org/10.1016/j.molbrainres.2004.12.003 [Google Scholar] [PubMed] [CrossRef]
Tremblay ME, Lowery RL, Majewska AK (2010). Microglial interactions with synapses are modulated by visual experience. PLoS Biology 8: e1000527. https://doi.org/10.1371/journal.pbio.1000527 [Google Scholar] [PubMed] [CrossRef]
Tsuji F, Murai M, Oki K, Seki I, Ueda K et al. (2010). Transient receptor potential vanilloid 1 agonists as candidates for anti-inflammatory and immunomodulatory agents. European Journal of Pharmacology 627: 332–339. https://doi.org/10.1016/j.ejphar.2009.10.044 [Google Scholar] [PubMed] [CrossRef]
Tyagi S, Shekhar N, Thakur AK (2022). Protective role of capsaicin in neurological disorders: An overview. Neurochemical Research 47: 1513–1531. https://doi.org/10.1007/s11064-022-03549-5 [Google Scholar] [PubMed] [CrossRef]
Ueno M, Fujita Y, Tanaka T, Nakamura Y, Kikuta J, Ishii M, Yamashita T (2013). Layer V cortical neurons require microglial support for survival during postnatal development. Nature Neuroscience 16: 543–551. https://doi.org/10.1038/nn.3358 [Google Scholar] [PubMed] [CrossRef]
Ulmann L, Levavasseur F, Avignone E, Peyroutou R, Hirbec H, Audinat E, Rassendren F (2013). Involvement of P2X4 receptors in hippocampal microglial activation after status epilepticus. Glia 61: 1306–1319. https://doi.org/10.1002/glia.22516 [Google Scholar] [PubMed] [CrossRef]
Umpierre AD, Bystrom LL, Ying Y, Liu YU, Worrell G, Wu LJ (2020). Microglial calcium signaling is attuned to neuronal activity in awake mice. eLife 9. https://doi.org/10.7554/eLife.56502.sa2 [Google Scholar] [CrossRef]
van der Stelt M, di Marzo V (2004). Endovanilloids. Putative endogenous ligands of transient receptor potential vanilloid 1 channels. European Journal of Biochemistry 271: 1827–1834. https://doi.org/10.1111/j.1432-1033.2004.04081.x [Google Scholar] [PubMed] [CrossRef]
Vezzani A (2014). Epilepsy and inflammation in the brain: Overview and pathophysiology. Epilepsy Currents 14: 3–7. https://doi.org/10.5698/1535-7511-14.s2.3 [Google Scholar] [PubMed] [CrossRef]
Vinet J, Weering HR, Heinrich A, Kalin RE, Wegner A et al. (2012). Neuroprotective function for ramified microglia in hippocampal excitotoxicity. Journal of Neuroinflammation 9: 27. https://doi.org/10.1186/1742-2094-9-27 [Google Scholar] [PubMed] [CrossRef]
Wallace MJ, Martin BR, DeLorenzo RJ (2002). Evidence for a physiological role of endocannabinoids in the modulation of seizure threshold and severity. European Journal of Pharmacology 452: 295–301. https://doi.org/10.1016/S0014-2999(02)02331-2 [Google Scholar] [PubMed] [CrossRef]
Wan Y, Feng B, You Y, Yu J, Xu C et al. (2020). Microglial displacement of GABAergic synapses is a protective event during complex febrile seizures. Cell Reports 33: 108346. https://doi.org/10.1016/j.celrep.2020.108346 [Google Scholar] [PubMed] [CrossRef]
Wang J, Kollarik M, Ru F, Sun H, McNeil B et al. (2017). Distinct and common expression of receptors for inflammatory mediators in vagal nodose versus jugular capsaicin-sensitive/TRPV1-positive neurons detected by low input RNA sequencing. PLoS One 12: e0185985. https://doi.org/10.1371/journal.pone.0185985 [Google Scholar] [PubMed] [CrossRef]
Wang X, Yang XL, Kong WL, Zeng ML, Shao L et al. (2019). TRPV1 translocated to astrocytic membrane to promote migration and inflammatory infiltration thus promotes epilepsy after hypoxic ischemia in immature brain. Journal of Neuroinflammation 16: 214. https://doi.org/10.1186/s12974-019-1618-x [Google Scholar] [PubMed] [CrossRef]
Wang X, Zhao L, Zhang J, Fariss RN, Ma W, Kretschmer F, Wong WT et al. (2016). Requirement for microglia for the maintenance of synaptic function and integrity in the mature retina. The Journal of Neuroscience 36: 2827–2842. https://doi.org/10.1523/JNEUROSCI.3575-15.2016 [Google Scholar] [PubMed] [CrossRef]
Wolf HK, Campos MG, Zentner J, Hufnagel A, Schramm J, Elger CE, Wiestler OD (1993). Surgical pathology of temporal lobe epilepsy. Experience with 216 cases. Journal of Neuropathology and Experimental Neurology 52: 499–506. https://doi.org/10.1097/00005072-199309000-00008 [Google Scholar] [PubMed] [CrossRef]
Wyatt SK, Witt T, Barbaro NM, Cohen-Gadol AA, Brewster AL (2017). Enhanced classical complement pathway activation and altered phagocytosis signaling molecules in human epilepsy. Experimental Neurology 295: 184–193. https://doi.org/10.1016/j.expneurol.2017.06.009 [Google Scholar] [PubMed] [CrossRef]
Yang S, Cox CL (2011). Attenuation of inhibitory synaptic transmission by glial dysfunction in rat thalamus. Synapse 65: 1298–1308. https://doi.org/10.1002/syn.20964 [Google Scholar] [PubMed] [CrossRef]
Zhang X, Huang J, McNaughton PA (2005). NGF rapidly increases membrane expression of TRPV1 heat-gated ion channels. The EMBO Journal 24: 4211–4223. https://doi.org/10.1038/sj.emboj.7600893 [Google Scholar] [PubMed] [CrossRef]
Zhang M, Ruwe D, Saffari R, Kravchenko M, Zhang W (2020). Effects of TRPV1 activation by capsaicin and endogenous N-arachidonoyl taurine on synaptic transmission in the prefrontal cortex. Frontiers in Neuroscience 14: 91. https://doi.org/10.3389/fnins.2020.00091 [Google Scholar] [PubMed] [CrossRef]
Cite This Article
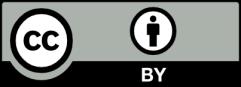