Open Access
ARTICLE
Growth, ROS Markers, Antioxidant Enzymes, Osmotic Regulators and Metabolic Changes in Tartary Buckwheat Subjected to Short Drought
1 Key Laboratory of Coarse Cereal Processing, Ministry of Agriculture and Rural Affairs, Sichuan Engineering & Technology Research Center of Coarse Cereal Industrialization, College of Food and Biological Engineering, Chengdu University, Chengdu, 610106, China
2 School of Preclinical Medicine, Chengdu University, Chengdu, 610106, China
3 Center for Agricultural Genetic Resources Research, Shanxi Agricultural University, Taiyuan, 030031, China
* Corresponding Authors: Liang Zou. Email: ; Dabing Xiang. Email:
# This author contributed equally to this work
(This article belongs to the Special Issue: Plant Physiology for Crop Production and Sustainable Agriculture)
Phyton-International Journal of Experimental Botany 2023, 92(1), 35-54. https://doi.org/10.32604/phyton.2022.021698
Received 31 January 2022; Accepted 18 April 2022; Issue published 06 September 2022
Abstract
Tartary buckwheat (Fagopyrum tataricum) is an important pseudocereal feed crop with medicinal and nutritional value. Drought is one of the main causes of reduced growth and yield in these plants. We investigated the growth, physiological, and metabolic responses of the widely promoted Tartary buckwheat variety Chuan Qiao No. 1 to polyethylene glycol (PEG)-mediated drought stress. Drought significantly decreased shoot length, shoot biomass and relative water content. Root length, malondialdehyde content, electrolyte leakage, activities of superoxide dismutase, peroxidase, catalase and amylase, and contents of soluble sugar, soluble protein and proline were increased by PEG-mediated drought. Untargeted metabolomics analysis identified 32 core metabolites in seedlings subjected to PEG-mediated drought, 16 of which increased—including quercetin, isovitexin, cyanidin 3-O-beta-D-glucoside, L-arginine, and glycerophosphocholine, while the other 16 decreased—including 3-methoxytyramine, 2, 6-diaminopimelic acid, citric acid, UDP-alpha-D-glucose, adenosine, keto-D-fructose. The 32 core metabolites were enriched in 29 metabolic pathways, including lysine biosynthesis, citrate (TCA) cycle, anthocyanin biosynthesis, and aminoacyl-tRNA biosynthesis. Among them, taurine and hypotaurine metabolism, flavor and flavor biosynthesis, indole alkaline biosynthesis, and alanine, aspartate and glutamate metabolism were the four main metabolic pathways affected by drought. Our findings provide new insights into the physiological and metabolic response mechanisms of Tartary buckwheat to drought stress.Keywords
Drought is the most important environmental constraint to crop productivity and food security worldwide [1]. Water deficit has pronounced effects on plant growth, survival, physiology, and yield [2,3]. Plants subjected to drought undergo evident morphological modifications, including reduced shoot growth (leaves and stems), and changes in root diameter, length, branches and lateral root formation, all of which disrupt plant–water relations and affect water-use efficiency [4,5]. Drought interferes with many physiological processes, including CO2 assimilation and metabolism, ultimately leading to a significant reduction in biomass accumulation [6,7]. However, plants respond to drought through physiological and metabolic changes, such as inhibited transpiration through a reduction in leaf area or stomatal closure, to successfully minimize water loss [8]. Drought induces the production of reactive oxygen species (ROS) and modifies antioxidant systems [5,9]. Plants can improve their capacity for osmotic adjustment by accumulating solutes to maintain cell turgidity, protecting against cellular oxidative damage, and altering metabolic pathways to tolerate or resist water deficit [10,11]. Furthermore, the plant cell undergoes changes at the transcript, protein, and metabolite levels to acclimate to water deficit [11–14].
Metabolomics, also known as metabonomics or metabolic profiling, provides a useful and rapid method for assessing the changes occurring in the metabolome as a consequence of the environment [1,15–17]. It is a powerful tool for analyzing plant responses to environmental stimuli, by identifying changes in the profile of primary and secondary metabolites [15,18–20]. Primary metabolism, including carbon and nitrogen metabolism, is altered by drought. Primary carbon metabolites such as polyols and sugars, and nitrogen-storage metabolites such as quaternary ammonium compounds or amino acids, have been found to increase in response to drought, mainly in plant roots [20]. Secondary metabolites, produced by pathways derived from primary metabolic routes, also play an important role in the drought response [17,21]. Water stress has been reported to lead to increases in gamma-aminobutyric acid, trigonelline, cyanogenic glycosides, glucosinolates, terpenoids, alkaloids and condensed tannins [2,16]. Under drought stress, the flavonoid pathway regulates the production of reactive oxygen species (ROS) [21]. Of the root-specific metabolites, gamma-glutamyl compounds have been found to accumulate significantly in response to drought stress reflecting induction of the glutathione pathway [21]. Plant hormones, such as gibberellic acid, indoleacetic acid (auxin), ethylene, cytokinin, abscisic acid and brassinosteroids, as well as signaling compounds involved in hormone metabolism, have been widely studied for their involvement in the drought response [22–26]. ROS and reactive nitrogen species metabolism is active in drought-stressed roots, characterized by increased protein nitration and accumulation of nitric oxide and S-nitrosothiols in cortical cells [3]. In addition to elucidating the metabolic differences between developmental stages or organs, it is essential to achieve an integrated understanding of the whole-plant response [21].
Tartary buckwheat (Fagopyrum tataricum) is a traditional short-season pseudocereal crop in the family Polygonaceae [27] that contains diverse health-promoting compounds, such as flavonoids, anthocyanins, phenolics, amino acids and vitamins [28–30]. Tartary buckwheat is widely distributed in Asia, in the marginal lands of mountainous areas of Western China, the Himalayas, Japan and Korea, as well as in Europe and the Americas [27,31]. Tartary buckwheat grows in environments that are harsh and cold, with strong ultraviolet radiation and frequent and severe drought conditions in high-altitude mountainous areas, and in arid or semiarid regions, causing defective growth and yield loss of Tartary buckwheat. However, a lack of metabolic profiling seriously limits our understanding of the drought-responsive mechanisms in Tartary buckwheat. An elucidation of these mechanisms should indicate specific traits to be targeted in selection programs, and help in implementing crop-management practices under drought conditions. In this study, we provide insight into the mechanism of Tartary buckwheat responses to drought in terms of plant growth and metabolome profile.
2.1 Plant Materials and Site Description
F. tataricum variety Chuan Qiao No. 1(CQ1), one of the main varieties in China which is widely used for field production, was used in this experiment. The seeds were cultured in a special device with nutritional supply and then placed in a growth chamber (25°C). The special device was designed for seed germination and growth under drought stress, without destroying the plant and allowing for seedling emergence [32]. For the water-deficit treatment, the 10-d-old Tartary buckwheat seedlings were treated with 25% (w/v) polyethylene glycol (PEG-6000) solution (Sigma-Aldrich, USA) for 3 days. Control plants were well-watered. Samples were collected from the aerial part of the seedlings in three independent biological replicates on days 1, 2 and 3 of the treatment for physiological index, while another six independent biological replicates were collected on day 2 of the treatment and immediately frozen in liquid nitrogen for metabolic profiling.
2.2 Sampling and Measurement Methods
2.2.1 Seedlings Growth, Biomass and Relative Water Content (RWC)
Stem lengths and main root lengths of 10 plants were recorded on day 3 of the treatment to calculate the ratio of root to stem length. Plants were oven-dried at 65°C until a constant weight was obtained. The average dry weight of 10 plants was calculated and expressed on a per plant basis (± SE). RWC was determined from fresh weight (FW), dry weight (DW), and turgid weight (TW) using the formula RWC (%) = [(FW-DW)/(TW-DW)] × 100 [33].
Electrolyte leakage was measured by the method as described in a previous study [34]. The content of malondialdehyde (MDA) was measured using the thiobarbituric acid colorimetric reaction (TBARS) assay [35].
2.2.3 Extraction and Analysis of Activity of Antioxidant Enzymes
Superoxide dismutase (SOD) activity was measured as described in a previous study [36]. Peroxidase (POD) and catalase (CAT) activities were determined according to a previous study [37], and amylase activity was measured as described before [38].
2.2.4 Analysis of Osmotic Regulators
Soluble protein content was measured by Bradford protein assay, soluble sugar content was measured as described before [39], and proline (Pro) content was quantified spectrophotometrically [38].
2.2.5 Gas Chromatography–Mass Spectrophotometry (GC–MS) Analysis
A plant sample (100 mg) was placed in a 5 mL centrifuge tube. Five steel balls were placed in liquid nitrogen for 5 min. The tubes were placed in a highflux organization grinding apparatus set at 70 Hz for 1 min. Methanol (1000 μL precooled at −20°C) was added and the mixture was vortexed for 30 s. The tubes were then placed into an ultrasound machine at room temperature for 30 min. Later, 750 μL chloroform (precooled at −20°C) and 800 μL deionized water (4°C) were added to the tubes. Then the tubes were vortexed for 60 s, centrifuged for 10 min at 12 000 rpm (GIVE IN g) at 4°C. 1 mL of the supernatant was transferred to a new centrifuge tube. Samples were vacuum-concentrated and then dissolved in 250 μL aqueous methanol solution (1:1, 4°C), filtered through a 0.22-μm membrane, and submitted for LC–MS analysis. As a quality control, 20 μL was taken from each prepared sample extract and mixed (quality control samples were used to monitor deviations of the analytical results from these pooled mixtures and compare them to the errors caused by the analytical instrument itself). The rest of the sample was used for LC–MS detection.
Chromatographic separation was accomplished in an ACQUITY UPLC system equipped with an ACQUITY UPLC® HSS T3 (150 × 2.1 mm, 1.8 μm, Waters) column maintained at 40°C. The temperature of the autosampler was 4°C. Gradient elution of the analytes was carried out with 0.1% formic acid in water (A) and 0.1% formic acid in acetonitrile (B) at a flow rate of 0.25 mL min−1. A 4-μL aliquot of the sample was injected after equilibration. An increasing linear gradient of solvent B (v/v) was used as follows: 0–1 min, 2% B; 1–9.5 min, 2%–50% B; 9.5–14 min, 50%–98% B; 14–15 min, 98% B; 15–15.5 min, 98%–2% B; 15.5–17 min, 2% B.
The HPLC-ESI-MSn experiments were executed in a Thermo LTQ–Orbitrap XL mass spectrometer with capillary voltages of 4.8 and 4.5 kV in negative (ESI−) and positive (ESI+) modes, respectively. Sheath gas and auxiliary gas were set at 45 and 15 arbitrary units, respectively. The capillary temperature was 325°C. The voltages of the capillary and tube were 35 and 50 V, and −15 and −50 V in positive and negative modes, respectively. The Orbitrap analyzer scanned over a mass range of m/z 89–1000 for full scan at a mass resolution of 60 000. Data-dependent acquisition in the MS/MS experiments was performed with collision-induced dissociation scan. The normalized collision energy was 30 eV. Dynamic exclusion was implemented with a repeat count of 2, and exclusion duration of 15 s.
2.3 Data Pretreatment and Multivariate Statistical Analysis
Data processing and analysis were performed according to Peng et al. [40] with slight modifications. The acquired MS data were analyzed by ChromaTOF software (v. 4.34, LECO, St. Joseph, MI). The dataset was normalized using the summed intensity of the peaks in each sample. In total, 546 peaks were detected in the tissues by GC–MS. The SIMCA-P + 13.0 software package (Umetrics, Umeå, Sweden) was used to analyze the GC–MS data. To visualize metabolic alterations in the experimental group, both unsupervised (principal component analysis, PCA) and supervised (orthogonal partial least squares discriminant analysis, OPLS-DA) segregation was exported. The parameters R2X(cum), R2Y(cum) and Q2(cum) were calculated to assess the quality of the models. After multivariate analysis, significant differences in each group were defined as having variable importance in projection (VIP) > 1.2, significance at P < 0.05 (t test) and fold change > 2; significantly altered metabolites were selected as candidates for further analysis.
2.4 Screening and Identification of Metabolic Differences
Differentially expressed compounds in the treatment group were selected by comparison to their control values using multivariate statistical method. Metabolites with both multivariate and univariate statistical significance (VIP > 1.0 and P < 0.05) were annotated with the aid of available reference standards in our laboratory, the NIST 05 standard mass spectral databases and the Feinh databases linked to ChromaTOF software. A similarity of > 70% was considered the reference standard. Based on the results of the enrichment, a customized vertical version of the histogram was created according to the significant vertical version of the top 10 entries in the column.
Statistical analyses were conducted using Excel 2010, SPSS 13.0 (Chicago, IL, USA) and SigmaPlot 10.0 (Aspire Software Intl., Ashburn, VA, USA). One-way ANOVA was used to determine the significance level, and means were compared by least significance difference (LSD) multiple comparison at a significance level of P < 0.05.
3.1 Seedling Growth, Total Biomass, and RWC
Compared to the well-watered controls, the samples treated with PEG-induced drought showed significantly decreased shoot length but increased root length (Fig. 1A). Shoot biomass was significantly reduced by the drought treatment, whereas root biomass showed the opposite trend. The ratio of root-to-shoot biomass was therefore enhanced by drought (Fig. 1B). Under conditions of drought stress, seedling RWC decreased sharply, whereas that in the control seedlings showed no significant change during the 3 days of the experiment (Fig. 1C).
Figure 1: Mean (± SE) root length (RL) and shoot length (SL) (A), root weight (RW), shoot weight (SW) and the ratio between root and shoot (ratio) (B), and relative water content (RWC) (C) under drought stress treatment
Notes: Different lowercase letters indicate significant differences between treatments overall and asterisks (*) represent significant differences between well-watered (CK) and water-stressed (TR) plants on corresponding days (P < 0.05).
3.2 MDA Content and Electrolyte Leakage
Compared to the control plants, MDA content in the drought-treated seedlings was significantly higher (Fig. 2A). During the 3-day treatment, MDA content in drought-treated seedlings increased from day 0 to day 2, then decreased from day 2 to day 3. Electrolyte leakage increased significantly on all 3 days under PEG-induced drought conditions, whereas it did not change in the controls (Fig. 2B).
Figure 2: Mean (± SE) MDA content(A) and electrolyte leakage(B) under drought stress treatment
Note: Different lowercase letters indicate significant differences between treatments overall, and asterisks (*) represent significant differences between well-watered (CK) and water-stressed (TR) plants on corresponding days (P < 0.05).
3.3 Activities of Antioxidant Enzymes and Amylase
Compared to the well-watered seedlings, SOD activity in the drought-treated seedlings was significantly higher on days 2 and 3 (Fig. 3A). Drought-stressed plants experienced a significant increase in POD activity compared to the well-watered controls on days 1 and 2 (Fig. 3B). CAT activity in the drought-treated seedlings was sharply elevated compared to the controls (Fig. 3C). Drought-stressed plants showed a significant increase in amylase activity compared to the well-watered controls over the 3 days of the experiment (Fig. 3D). During the 3-day period, SOD, POD, CAT and amylase activities of drought-stressed plants were highest on day 2.
Figure 3: Mean (± SE) the SOD activity (A), POD activity (B), CAT activity (C) and amylase activity (D) under drought stress treatment
Note: Different lowercase letters indicate significant differences between treatments overall, and asterisks (*) represent significant differences between well-watered (CK) and water-stressed (TR) plants on corresponding days (P < 0.05).
Drought stress had stimulatory effects on soluble sugar, soluble protein and Pro contents (Figs. 4A–4C). Soluble sugar content of drought-stressed seedlings was significantly higher than that of controls on days 1 and 2. Compared to the controls, soluble protein content was enhanced by drought treatment on all days, whereas Pro content increased on days 2 and 3. During the 3 days of the experiment, soluble sugar, soluble protein and Pro contents in the drought-treated seedlings were highest on day 2.
Figure 4: Mean (± SE) of the soluble sugar content (A), the soluble protein content (B), and Pro content (C) under drought stress treatment
Note: Different lowercase letters indicate significant differences between treatments overall, and asterisks (*) represent significant differences between well-watered (CK) and water-stressed (TR) plants on corresponding days (P < 0.05).
3.5 Metabolic Changes Caused by Drought
3.5.1 Identification of Differentially Expressed Metabolites
GC–MS was used for maximal separation of the wide range of metabolites present in the drought-treated seedlings. In total, 1017 differentially expressed metabolites were identified in the drought-treated compared to control treatments (592 increased and 425 decreased) comprising organic acids, sugars, sugar alcohols, fatty acids, amino acids, flavones, sterols and phospholipids, as identified using the NIST library.
PCA was performed using the six individual replicates from each comparison group. The two principal components could explain 54.1% and 54.7% of the variation between drought-treated and control seedlings for the negative and positive ESI modes, respectively (Figs. 5A and 5B). By OPLS-DA, the two principal components explained 53.4% and 50.0% of the variation between treated and control seedlings for the negative and positive ESI modes, respectively (Figs. 5C and 5D). To test for model reproducibility and model fit, response-sorting tests of the OPLS-DA model, namely, fixed X matrix and previously defined Y matrix variable classifications (i.e., 0 or 1) were randomly arranged. The OPLS-DA model was established to obtain the corresponding stochastic model of R2 and Q2 values. With the original model of R2Y and Q2Y linear regression, the regression line and the Y axis intercept values (R2 and Q2) were used to measure whether the model was over-fitted (Figs. 5E and 5F). The model remained valid after cross-validation.
Figure 5: Principal component analysis (PCA) score plots of all samples (ESI-and ESI+) for the negative (A) and positive (B) ESI modes, PLS-DA score plots for the negative (C) and positive (D) ESI modes, permutation test for the negative (E) and positive (F) ESI modes. Each data point represents an independent sample
3.5.2 Metabolic Profiles and key Metabolites Affected by Drought
For the metabolomics analysis, 32 core metabolites of Tartary buckwheat were identified and quantified (Table 1, Fig. 6). A heat map showed overall changes in the 32 metabolites under normal vs. drought conditions (Fig. 7). Among them, quercetin, isovitexin, cyanidin 3-O-beta-D-glucoside, L-arginine, glycerophosphocholine, L-aspartic acid, tryptamine, (-)-epicatechin, taurine, quercetin 3-O-beta-D-glucopyranoside, N-acetyl-D-phenylalanine, carprofen, 2-deoxy-alpha-D-ribopyranose, 1-aminocyclobutane carboxylic acid, dimethyl phthalate, L-asparagine, and 2-dehydro-D-gluconic acid accumulated in the drought-treated seedlings (Figs. 6 and 7). The compounds 3-methoxytyramine, 2, 6-diaminopimelic acid, citric acid, UDP-alpha-D-glucose, adenosine, keto-D-fructose, enterolactone, L-pipecolic acid, erbstatin analog, vanillylmandelic acid, UMP, fumaric acid, CMP, galactitol, and thio-m-toluthioamide showed higher accumulations in the well-watered seedlings (Figs. 6 and 7).
Figure 6: Metabolites changes in Tartary buckwheat at 2 days after treatment. Green histograms correspond to the control and blue histograms to the treatment
Figure 7: Heat map of changes in 32 metabolites in Tartary buckwheat at 2 days after treatment
Results show log2 fold change ratios; red indicates an upregulation; green indicates a down-regulation. Color intensity codes are given in the color bar at the right side of the figures. Different clusters containing the metabolites with the same behavior are outlined.
3.5.3 Metabolic Pathways Affected by Drought
The 32 core metabolites were enriched in 29 metabolic pathways (Table 2). The pathways included alanine, aspartate and glutamate metabolism, flavone and flavonol biosynthesis, lysine biosynthesis, citrate cycle (TCA cycle), anthocyanin biosynthesis, aminoacyl-tRNA biosynthesis, pyrimidine metabolism, zeatin biosynthesis, and purine metabolism. With fold change > 0.2 as the screening condition, the KEGG metabolic pathways taurine and hypotaurine metabolism, flavor and flavor biosynthesis, indole alkaline biosynthesis, and alanine, aspartate and glutamate metabolism were most highly represented.
Tartary buckwheat is an important pseudocereal crop worldwide. Due to its limited growing area, Tartary buckwheat is typically exposed to a number of environmental stresses, such as high light/UV radiation, cold, drought, salt, and heavy metals. Drought severely affects growth and yield of Tartary buckwheat in agricultural production. Unfortunately, little is known about the effect of drought on Tartary buckwheat and its response to water stress. This motivated the current study.
4.1 Seedling Growth, Physiological and Biochemical Indexes
Previous research has shown the many adverse effects of drought stress on plant growth, physiological metabolism and biochemical parameters [41], ultimately resulting in yield reduction or even plant death [42,43] . We found a significant reduction in shoot biomass and shoot length of Tartary buckwheat plants under drought, indicating inhibition of plant shoot growth under the drought treatment, as reported in wheat and other plants [44]. However, root length increased under drought, confirming the accessibility of moisture deep in the soil and ensuring adaptation to drought stress. The observed decline of RWC under water stress can damage the plant’s ability to maintain its water balance [45]. Stressed plants exhibit excess generation of ROS which can cause membrane lipid peroxidation, leading to cell damage or death [46]. Enhanced H2O2, MDA contents and electrolyte leakage are common symptoms of membrane damage under water stress [45,47]. MDA is the final product of membrane lipid peroxidation and indicates cell membrane injury [35], and electrolyte leakage is considered a marker of membrane damage under drought [47]. In our study, MDA and electrolyte leakage were significantly increased in the treated compared to control seedlings, indicating that drought can result in membrane damage; significant differences in MDA content and electrolyte leakage appeared at 24 h into the drought treatment.
To alleviate drought-induced oxidative stress, antioxidant enzymes function as ROS scavengers [47]. SOD is the first enzyme to play a key role in oxidative defense by catalyzing superoxide free radical dismutation of O2− to H2O2 [48]; the generated H2O2 is then eliminated by CAT and POD [47,49] through its catalysis to H2O and O2 [45]. SOD activity in Tartary buckwheat increased significantly 24 h after drought induction, and continued to increase to 48 h of drought. Significant increases in POD and CAT activity have been shown in stressed plants [45]. POD and CAT activity in Tartary buckwheat showed the same trend as SOD activity under the drought treatment. Increases in SOD, POD and CAT activities in Tartary buckwheat after rehydration could reflect an adaptation toward alleviating oxidative stress and improving growth, as in other plants [50].
Typically, osmotically active solutes such as Pro, soluble proteins and soluble sugars can mitigate the oxidative damage caused by ROS under water stress [51]. These molecules stimulate plants to absorb more water to withstand dehydration by maintaining turgor, buffering against ROS and protecting the redox balance [51] . Among them, Pro exhibits antioxidant activity and promotes redox to maintain homeostasis, thereby reducing lipid peroxidation [52]. The Pro content of Tartary buckwheat showed a significant and remarkable increase under drought stress 48 h after drought initiation. Plant accumulation of soluble proteins and soluble sugars under drought stress has been found in many studies [48]. Here, the contents of these two osmotic-adjustment substances showed the same trends, mitigating drought damage.
4.2 Secondary Metabolites and Pathways
Plants tend to biosynthesize specialized metabolites to adapt to drought stress. We identified 32 core metabolites that were enriched in 29 metabolic pathways in a Tartary buckwheat variety CQ1. Of these core metabolites, taurine accumulated significantly in response to drought stress (Fig. 6). In plants, an increase in taurine reflects induction of the taurine and hypotaurine metabolic pathway (Table 2). Taurine plays an important role in adjusting normal physiological functions to confer stress resistance via osmotic regulation or antioxidation [53].
Flavonoids are postulated to contribute antioxidant protection, due to their hydroxyl groups, the presence of double bonds, and their predisposition toward glycosylation and methylation [54]. Quercetin is considered one of the best electron donors among the flavonoids as it has an ortho-dihydroxy pattern in the B-ring of the flavonoid skeleton [55]. In Tartary buckwheat, we found quercetin to be associated with quercetin 3-O-beta-D-glucopyranoside in flavone and flavonol biosynthesis improved under drought conditions (Fig. 6, Table 2), and the increase in quercetin concentration conferred greater H2O2− scavenging activity [56], which might be a strategy for drought tolerance due to the powerful antioxidant activity of flavonoids [57].
Tryptophan is the substrate for alkaloids, indoles, auxin, phytomelatonin, glucosinolates and phytoalexins, and it therefore plays an important role in the plant stress response [58]. Tryptamine is biosynthesized from tryptophan by trytophan decarboxylase and oxidized by monoamine oxidase [59]. Tryptamine is one of the major contributors to the water-stress response in barley [60]. In rice, the indole alkaloid tryptamine inhibited infection by Magnaporthe oryzae as a key factor in light-enhanced resistance [59]. In Tartary buckwheat, tryptamine increased through the indole alkaline biosynthesis pathway under drought conditions.
The accumulation of amino acids has been reported in various plants under abiotic stress. They are considered to have a beneficial effect during stress acclimation in some species, or to indicate cell damage in others [61,62]. In maize, arginine was considered an osmoprotectant as it increased under water stress [61]. In a Fagus sylvatica L. population, aspartic acid together with valine, isoleucine, threonine, serine and glycine, increased in response to water stress [63]. In our study, L-aspartic acid and L-asparagine involved in alanine, aspartate and glutamate metabolism were upregulated under drought conditions, whereas fumaric acid decreased.
To our knowledge, this is the first study of physiological responses and identification of the metabolic profile of Tatary buckwheat under drought stress. ROS markers, including MDA content and electrolyte leakage, activities of the antioxidant enzymes SOD, POD and CAT, and contents of the osmotic regulators soluble sugar, soluble protein and Pro all increased in response to drought stress in Tatary buckwheat, along with metabolites that included organic acids, sugars, sugar alcohols, fatty acids, amino acids, flavones, sterols and phospholipids. Taurine and hypotaurine metabolism, flavone and flavonol biosynthesis, indole alkaline biosynthesis, alanine, aspartate and glutamate metabolism might be the main metabolic pathways used to cope with drought stress. The presented information on the metabolic profile should provide new insights into the biochemical pathways underlying drought tolerance in Tartary buckwheat plants.
Authorship: Conceptualization, Yan Wan, Dabing Xiang and Liang Zou; Data curation, Yan Wan, Yuan Liang, Xuxiao Gong, and Jianyong Ouyang; Formal analysis, Jingwei Huang and Xiaoyong Wu; Funding acquisition, Yan Wan, Dabing Xiang and Liang Zou; Investigation, Liang Yuan, and Changying Liu; Methodology, Yuan Liang, Xuxiao Gong, and Xiaoning Cao; Project administration, Xuxiao Gong and Liang Yang, and Jiangyong Ouyang; Resources, Dabing Xiang, Qi Wu and Gang Zhao; Supervision, Dabing Xiang, Gang Zhao and Liang Zou; Visualization, Liang Zou; Writing–original draft, Yan Wan, Yuan Liang, Xuxiao Gong, Jianyong Ouyang,; Writing–review & editing, Xueling Ye, Changying Liu, Xiaoyong Wu, Qi Wu and Gang Zhao.
Funding Statement: We acknowledge the Project of National Key Research and Development Program of China (2020YFD1001403), China Agriculture Research System (CARS-07-B-1), Science & Technology Department of Sichuan Province (2022YFQ0041), the National Natural Science Foundation of China (31601260, 32160428), Innovative Training Program for College Students (S202111079058) and Special Research Fund from Key Laboratory of Coarse Cereal Processing, Ministry of Agriculture and Rural Affairs (2020CC012) to facilitate the research.
Conflicts of Interest: The authors declare that they have no conflicts of interest to report regarding the present study.
References
1. Omena-Garcia, P. P., Oliveira, M., Medeiros, D. B., Vallarino, J. G., Ribeiro, D. M. et al. (2019). Growth and metabolic adjustments in response to gibberellin deficiency in drought stressed tomato plants. Environmental and Experimental Botany, 159, 95–107. DOI 10.1016/j.envexpbot.2018.12.011. [Google Scholar] [CrossRef]
2. Charlton, A. J., Donarski, J. A., Harrison, M., Jones, S. A., Godward, J. et al. (2008). Responses of the pea (Pisum sativum L.) leaf metabolome to drought stress assessed by nuclear magnetic resonance spectroscopy. Metabolomics, 4, 312. DOI 10.1007/s11306-008-0128-0. [Google Scholar] [CrossRef]
3. Santiago, S., Corpas, F. J., Marta, R. R., Raquel, V., Barroso, J. B. et al. (2019). Drought stress triggers the accumulation of NO and SNOS in cortical cells of Lotus japonicus L. rootsand the nitration of proteins with relevant metabolic function. Environmental and Experimental Botany, 161, 228–241. DOI 10.1016/j.envexpbot.2018.08.007. [Google Scholar] [CrossRef]
4. Lynch, J. P., Chimungu, J. G., Brown, K. M. (2014). Root anatomical phenes associated with water acquisition from drying soil: Targets for crop improvement. Journal of Experimental Botany, 65, 6155–6166. DOI 10.1093/jxb/eru162. [Google Scholar] [CrossRef]
5. Ye, H., Manish, R., Babu, V., Zhou, L., Chen, P. Y. et al. (2018). Genetic diversity of root system architecture in response to drought stress in grain legumes. Journal of Experimental Botany, 697, 3267–327. DOI 10.1093/jxb/ery082. [Google Scholar] [CrossRef]
6. Flexas, J., Bota, J., Loreto, F., Cornic, G., Sharkey, T. D. (2004). Diffusive and metabolic limitations to photosynthesis under drought and salinity in C3 plants. Plant Biology, 6, 269–279. DOI 10.1055/s-2004-820867. [Google Scholar] [CrossRef]
7. Farooq, M., Wahid, A., Kobayashi, N., Fujita, D., Basra, S. (2009). Plant drought stress: Effects, mechanisms and management. Agronomy for Sustainable Development, 29, 185–212. DOI 10.1051/agro:2008021. [Google Scholar] [CrossRef]
8. Claeys, H., Inze, D. (2013). The agony of choice: How plants balance growth and survival under water-limiting conditions. Plant Physiology, 162, 1768–1779. DOI 10.1104/pp.113.220921. [Google Scholar] [CrossRef]
9. Xu, Y., Burgess, P., Zhang, X., Huang, B. (2016). Enhancing cytokinin synthesis by overexpressing IPT alleviated drought inhibition of root growth through activating ROS-scavenging systems in agrostis stolonifera. Journal of Experimental Botany, 67, 1979–1992. DOI 10.1093/jxb/erw019. [Google Scholar] [CrossRef]
10. Carla, P., Maria, M. C., Ricardo, C. P. (2001). Alterations in carbon and nitrogen metabolism induced by water deficit in the stems and leaves of Lupinus albus L. Journal of Experimental Botany, 52, 1063–1070. DOI 10.1093/jexbot/52.358.1063. [Google Scholar] [CrossRef]
11. Shulaev, V. C., Miller, G., Mittler, R. (2008). Metabolomics for plant stress response. Physiology Plantarum, 132, 199–208. DOI 10.1111/j.1399-3054.2007.01025.x. [Google Scholar] [CrossRef]
12. Ahuja, I., Vos, R. D., Bones, A. M., Hall, R. D. (2010). Plant molecular stress responses face climate change. Trends Plant Science, 15, 664–674. DOI 10.1016/j.tplants.2010.08.002. [Google Scholar] [CrossRef]
13. Chae, L., Sudat, S., Dudoit, S., Zhu, T., Luan, S. (2009). Diverse transcriptional programs associated with environmental stress and hormones in the arabidopsis receptor-like kinase gene family. Molecular Plant, 2, 84–107. DOI 10.1093/mp/ssn083. [Google Scholar] [CrossRef]
14. Yates, S. A., Swain, M. T., Hegarty, M. J., Chernukin, I., Lowe, M. et al. (2014). De novo assembly of red clover transcriptome based on RNA-SEQ data provides insight into drought response, gene discovery and marker identification. BMC Genomics, 15, 453–453. DOI 10.1186/1471-2164-15-453. [Google Scholar] [CrossRef]
15. Cai, X., Sun, X. G., Che, N. X., Fu, J. Q., Zhang, Y. L. (2016). Metabolic and growth responses of maize to successive drought and re-watering cycles. Agricultural Water Management, 172, 62–73. DOI 10.1016/j.agwat.2016.04.016. [Google Scholar] [CrossRef]
16. Pan, L., Chen, M., Wang, J., Ma, X., Fan, X. et al. (2018). Integrated omics data of two annual ryegrass (Lolium multiflorum L.) genotypes reveals core metabolic processes under drought stress. BMC Plant Biology, 18, 26–42. DOI 10.1186/s12870-018-1239-z. [Google Scholar] [CrossRef]
17. Xiong, Q., Cao, C., Shen, T., Zhong, L., He, H. H. et al. (2019). Comprehensive metabolomic and proteomic analysis in biochemical metabolic pathways of rice spikes under drought and submergence stress. BBA-Proteins & Proteomic, 1967, 237–247. DOI 10.1016/j.bbapap.2019.01.001. [Google Scholar] [CrossRef]
18. Warren, C. R., Aranda, I., Cano, F. J. (2011). Responses to water stress of gas exchange and metabolites in eucalyptus and acacia spp. Plant Cell and Environment, 34, 1609–1629. DOI 10.1111/j.1365-3040.2011.02357.x. [Google Scholar] [CrossRef]
19. Diego, N. D., Sampedro, M. C., Barrio, R. J., Saiz-Fernández, I., Moncaleán, P. et al. (2013). Solute accumulation and elastic modulus changes in six radiata pine breeds exposed to drought. Tree Physiology, 33, 69–80. DOI 10.1093/treephys/tps125. [Google Scholar] [CrossRef]
20. Pinheiro, C., António, C., Ortuño, M. F., Dobrev, P. I., Hartung, W. et al. (2011). Initial water deficit effects on lupinus albus photosynthetic performance, carbon metabolism, and hormonal balance: Metabolic reorganization prior to early stress responses. Journal of Experimental Botany, 62, 4965–4974. DOI 10.1093/jxb/err194. [Google Scholar] [CrossRef]
21. Miguel, M. D., Guevara, M. N., Sánchez-Gómez, D., María, N. D., Díaz, L. M. et al. (2016). Organ-specific metabolic responses to drought in Pinus pinaster Ait. Plant Physiology and Biochemsity, 102, 17–26. [Google Scholar]
22. Dong, Q., Zhang, Z., He, J. X., Zhang, P., Ou, X. B. et al. (2019). Arabidopsis ADF5 promotes stomatal closure by regulating actin cytoskeleton remodeling in response to aba and drought stress. Journal of Experimental Botany, 70, 435–446. DOI 10.1093/jxb/ery385. [Google Scholar] [CrossRef]
23. Nurunnaher, A., Islam, M. R., Karim, M. A., Hossain, T. (2014). Alleviation of drought stress in maize by exogenous application of gibberellic acid and cytokinin. Journal of Crop Science & Biotechnology, 17, 41–48. DOI 10.1007/s12892-013-0117-3. [Google Scholar] [CrossRef]
24. Remy, E., Cabrito, T. R., Baster, P., Batista, R. A., Teixeira, M. C. et al. (2013). A major facilitator superfamily transporter plays a dual role in polar auxin transport and drought stress tolerance in Arabidopsis. Plant Cell, 25, 901–926. DOI 10.1105/tpc.113.110353. [Google Scholar] [CrossRef]
25. Shi, J. R., Habben, J. E., Archibald, R. L., Drummond, B. J., Chamberlin, M. A. et al. (2015). Overexpression of argos genes modifies plant sensitivity to ethylene, leading to improved drought tolerance in both Arabidopsis and maize. Plant Physiology, 169, 266–282. DOI 10.1104/pp.15.00780. [Google Scholar] [CrossRef]
26. Yuan, G. F., Jia, C. G., Li, Z., Sun, B., Zhang, L. P. et al. (2010). Effect of brassinosteroids on drought resistance and abscisic acid concentration in tomato under water stress. Scientia Horticulturae, 126, 103–108. DOI 10.1016/j.scienta.2010.06.014. [Google Scholar] [CrossRef]
27. Zhang, L., Li, X., Ma, B., Gao, Q., Du, H. et al. (2017). The tartary buckwheat genome provides insights into rutin biosynthesis and abiotic stress tolerance. Molecular Plant, 10, 1224–1237. DOI 10.1016/j.molp.2017.08.013. [Google Scholar] [CrossRef]
28. Zhu, F. (2016). Chemical composition and health effects of tartary buckwheat. Food Chemistry, 203, 231–245. DOI 10.1016/j.foodchem.2016.02.050. [Google Scholar] [CrossRef]
29. Bonafaccia, G., Marocchini, M., Kreft, I. (2003). Composition and technological properties of the flour and bran from common and tartary buckwheat. Food Chemistry, 80, 9–15. DOI 10.1016/S0308-8146(02)00228-5. [Google Scholar] [CrossRef]
30. Zou, L., Wu, D. T., Ren, G. X., Hu, Y. C., Peng, L. X. et al. (2021). Bioactive compounds, health benefits, and industrial applications of tartary buckwheat (Fagopdyrum tataricum). Critical Reviews in Food Science and Nutrition, DOI 10.1080/10408398.2021.1952161. [Google Scholar] [CrossRef]
31. Ohnism, O., Tomiyosm, M. (2005). Distribution of cultivated and wild buckwheat species in the NU river valley of Southwestern China. Fagopyrum, 22, 1–5. [Google Scholar]
32. Wan, Y., Ouyang, J. Y., Zhao, G., Xiang, D., Wu, X. et al. (2018). Equipment for Tartary buckwheat during the seed germination. China’s Intellectual Property Office, ZL201820533089.1. Beijing, China: China National Intellectual Property Administration. [Google Scholar]
33. Barrs, H. D., Weatherley, P. E. (1962). A re-examination of the relative turgidity technique for estimating water deficits in leaves. Australian Journal of Biological Sciences, 115, 413–428. DOI 10.1071/BI9620413. [Google Scholar] [CrossRef]
34. Blum, A., Ebercon, A. (1981). Cell membrane stability as a measure of drought and heat tolerance in wheat. Crop Science, 21, 43–47. DOI 10.2135/cropsci1981.0011183X002100010013x. [Google Scholar] [CrossRef]
35. Hodges, D. M., Delong, J. M., Prange, K. R. K. (1999). Improving the thiobarbituric acid-reactive-substances assay for estimating lipid peroxidation in plant tissues containing anthocyanin and other interfering compounds. Planta, 207, 604–611. DOI 10.1007/s004250050524. [Google Scholar] [CrossRef]
36. Giannopolitis, C. N., Ries, S. K. (1977). Superoxide dismutases: I. occurrence in higher plants. Plant Physiology, 59, 309–314. DOI 10.1104/pp.59.2.309. [Google Scholar] [CrossRef]
37. Chance, M., Maehly, A. C. (1954). Assay of catalases and peroxidases. Methods Enzymol, 2, 764–775. DOI 10.1016/S0076-6879(55)02300-8. [Google Scholar] [CrossRef]
38. Bates, L. S., Waldren, R. P., Teare, I. D. (1973). Rapid determination of free proline for water-stress studies. Plant Soil, 39, 205–207. DOI 10.1007/BF00018060. [Google Scholar] [CrossRef]
39. Yemm, E. W., Willis, A. J. (1954). The estimation of carbohydrates in plant extracts by anthrone. Biochemical. Journal, 57, 508–514. [Google Scholar]
40. Peng, B. J., Kuo, M. Y., Yang, P. H., Hewitt, J. T., Boswell, P. G. (2014). A practical methodology to measure unbiased gas chromatographic retention factor vs. temperature relationships. Journal of Chromatography A, 1374, 207–215. DOI 10.1016/j.chroma.2014.11.018. [Google Scholar] [CrossRef]
41. Okunlola, G. O., Olatunji, O. A., Akinwale, R. O., Tariq, A., Adelusi, A. A. (2017). Physiological response of the three most cultivated pepper species (Capsicum spp.) in Africa to drought stress imposed at three stages of growth and development. Scientia Horticulturae, 224, 198–205. DOI 10.1016/j.scienta.2017.06.020. [Google Scholar] [CrossRef]
42. Deeba, F., Pandey, A. K., Ranjan, S., Mishra, A., Singh, R. et al. (2012). Physiological and proteomic responses of cotton (Gossypium herbaceum L.) to drought stress. Plant Physiology and Biochemistry, 53, 6–18. DOI 10.1016/j.plaphy.2012.01.002. [Google Scholar] [CrossRef]
43. Qureshi, M. K., Munir, S., Shahzad, A. N., Rasul, S., Nouman, W. et al. (2018). Role of reactive oxygen species and contribution of new players in defense mechanism under drought stress in rice. International Journal of Agriculture and Biology, 20, 1339–1352. [Google Scholar]
44. Faraji, J., Sepehri, A. (2019). Ameliorative effects of TiO2 nanoparticles and sodium nitroprusside on seed germination and seedling growth of wheat under peg-stimulated drought stress. Journal of Seed Science, 41, 309–317. DOI 10.1590/2317-1545v41n3213139. [Google Scholar] [CrossRef]
45. Gao, S. S., Wang, Y. L., Yu, S., Huang, Y. Q., Liu, H. C. et al. (2020). Effects of drought stress on growth, physiology and secondary metabolites of two adonis species in Northeast China. Scientia Horticulturae, 259, 108795DOI 10.1016/j.scienta.2019.108795. [Google Scholar] [CrossRef]
46. Reddy, A. R., Chaitanya, K. V., Vivekanandan, M. (2004). Drought-induced responses of photosynthesis and antioxidant metabolism in higher plants. Journal of Plant Physiology, 161, 1189–1202. DOI 10.1016/j.jplph.2004.01.013. [Google Scholar] [CrossRef]
47. Sarker, U., Oba, S. (2018). Catalase, superoxide dismutase and ascorbate-glutathione cycle enzymes confer drought tolerance of amaranthus tricolor. Scientific Reports, 8, 16496. DOI 10.1038/s41598-018-34944-0. [Google Scholar] [CrossRef]
48. Cheng, L., Han, M., Yang, L. M., Yang, L., Sun, Z. et al. (2018). Changes in the physiological characteristics and baicalin biosynthesis metabolism of Scutellaria baicalensis georgi under drought stress. Industrial Crops and Products, 122, 473–482. DOI 10.1016/j.indcrop.2018.06.030. [Google Scholar] [CrossRef]
49. Liu, C. C., Liu, Y. G., Guo, K., Fan, D. Y., Li, G. G. et al. (2011). Effect of drought on pigments, osmotic adjustment and antioxidant enzymes in six woody plant species in karst habitats of Southwestern China. Environmental and Experimental Botany, 71, 174–183. DOI 10.1016/j.envexpbot.2010.11.012. [Google Scholar] [CrossRef]
50. Shi, Q., Yin, Y. L., Wang, Z. Q., Fan, W. C., Hua, J. F. (2016). Physiological acclimation of taxodium hybrid ‘Zhongshanshan 118’ plants to short-term drought stress and recovery. HortScience, 51, 1159–1166. DOI 10.21273/HORTSCI10997-16. [Google Scholar] [CrossRef]
51. Szabados, L., Savoure, A. (2010). Proline: A multifunctional amino acid. Trends Plant Science, 15, 89–97. DOI 10.1016/j.tplants.2009.11.009. [Google Scholar] [CrossRef]
52. Mansour, M. M. F., Ali, E. (2017). Evaluation of proline functions in saline conditions. Phytochemistry, 140, 52–68. DOI 10.1016/j.phytochem.2017.04.016. [Google Scholar] [CrossRef]
53. Cai, J. G., Luo, L. M., Tang, H., Zhou, L. (2018). Cytotoxicity of malondialdehyde and cytoprotective effects of taurine via oxidative stress and PGC-1α signal pathway in C2C12 cells. Molecular Biology, 52, 532–542. DOI 10.1134/S0026893318040040. [Google Scholar] [CrossRef]
54. Sadowska-Bartosz, I., Adamczyk, R., Bartosz, G. (2014). Protection against peroxynitrite reactions by flavonoids. Food Chemistry, 164, 228–233. DOI 10.1016/j.foodchem.2014.04.105. [Google Scholar] [CrossRef]
55. Jovanovic, S. V., Steenken, S., Hara, Y., Simic, M. G. (1996). Reduction potentials of flavonoid and model phenoxyl radicals. which ring in flavonoids is responsible for antioxidant activity? Journal of the Chemical Society, Perkin Transactions, 2(11), 2497–2504. DOI 10.1039/p29960002497. [Google Scholar] [CrossRef]
56. Fini, A., Guidi, L., Ferrini, F., Brunetti, C., Ferdinando, M. et al. (2012). Drought stress has contrasting effects on antioxidant enzymes activity and phenylpropanoid biosynthesis in fraxinus ornus leaves: An excess light stress affair?. Journal of Plant Physiology, 169, 929–939. DOI 10.1016/j.jplph.2012.02.014. [Google Scholar] [CrossRef]
57. Gharibi, S., Tabatabaei, B. E. S., Saeidi, G., Talebi, M., Matkowski, A. (2019). The effect of drought stress on polyphenolic compounds and expression of flavonoid biosynthesis related genes in achillea pachycephala rech. Phytochemistry, 162, 90–98. DOI 10.1016/j.phytochem.2019.03.004. [Google Scholar] [CrossRef]
58. Yu, Y., Lv, Y., Shi, Y. N., Li, T., Chen, Y. C. et al. (2018). The role of phyto-melatonin and related metabolites in response to stress. Molecules, 23, 1887. DOI 10.3390/molecules23081887. [Google Scholar] [CrossRef]
59. Ueno, M., Kihara, J., Arase, S. (2015). Tryptamine and sakuranetin accumulation in sekiguchi lesions associated with the light-enhanced resistance of the lesion mimic mutant of rice to Magnaporthe Oryzae. Journal of General Plant Pathology, 81, 1–4. DOI 10.1007/s10327-014-0560-0. [Google Scholar] [CrossRef]
60. Marchetti, C. F., Ugena, L., Humplik, J. F., Polak, M., Zeljkovic, S. C. et al. (2019). A novel image-based screening method to study water-deficit response and recovery of barley populations using canopy dynamics phenotyping and simple metabolite profiling. Frontiers in Plant Science, 10, 20. DOI 10.3389/fpls.2019.01252. [Google Scholar] [CrossRef]
61. Sun, C. X., Li, M. Q., Gao, X. X., Liu, L. N., Wu, X. F. et al. (2016). Metabolic response of maize plants to multi-factorial abiotic stresses. Plant Biology, 18(Suppl 1), 120–9. DOI 10.1111/plb.12305. [Google Scholar] [CrossRef]
62. Singh, M., Kumar, J., Singh, S., Singh, V. P., Prasad, S. M. (2015). Roles of osmoprotectants in improving salinity and drought tolerance in plants: A review. Reviews in Environmental Science & Biotechnology, 14, 407–426. DOI 10.1007/s11157-015-9372-8. [Google Scholar] [CrossRef]
63. Aranda, I., Sanchez-Gomez, D., Cadahia, E., de Simon, B. F. (2018). Ecophysiological and metabolic response patterns to drought under controlled condition in open-pollinated maternal families from a Fagus sylvatica lL population. Environmental and Experimental Botany, 150, 209–221. DOI 10.1016/j.envexpbot.2018.03.014. [Google Scholar] [CrossRef]
Cite This Article
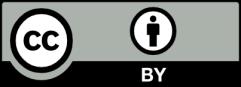