Open Access
ARTICLE
Comparison of Apigenin, Quercetin and Kaempferol Accumulation and Total Flavonoid Content in Leaves, Embryogenic Cultures and Cell Suspension Cultures of Parsley (Petroselinum crispum)
1
Analytical Laboratory, National Technological Institute of Mexico, Tuxtla Gutierrez Campus, Tuxtla Gutierrez, Mexico
2
Plant Biotechnology Laboratory, Instrumental Analysis Laboratory, Plant Biochemistry Laboratory, National Technological
Institute of Mexico, Tlajomulco de Zuniga, Mexico
3
Department of Cellular Biology and Genetics, Faculty of Biological Sciences, Autonomous University of Nuevo Leon, San Nicolas de
los Garza, Mexico
* Corresponding Authors: Martha Alicia Rodríguez-Mendiola. Email: ; Carlos Arias-Castro. Email:
(This article belongs to the Special Issue: Biotechnology of Plant Secondary Metabolites)
Phyton-International Journal of Experimental Botany 2023, 92(10), 2807-2823. https://doi.org/10.32604/phyton.2023.030396
Received 04 April 2023; Accepted 25 June 2023; Issue published 15 September 2023
Abstract
In recent years, there has been a growing interest in exploring alternative treatments for bone defects. Bone tissue engineering has turned its attention to plant extracts containing osteogenic flavonoids as potential promoters of bone regeneration. In our study, we specifically investigated the extract of Petroselinum crispum, a plant known for its abundance of osteogenic flavonoids such as apigenin, quercetin, and kaempferol. Our objective was to compare the total flavonoid content (TFC) and their accumulation in different sources. We obtained hydrolyzed aqueous extracts from the leaves of parsley plants (grown for 12 weeks in the field), weekly embryogenic cultures, and suspension cell cultures. The TFC, measured in mg quercetin equivalent per gram of dry weight (QE mg/g dw), was found to be 53.81 ± 0.97 for 52 g dw of leaves (1 kg plant fw), 31.10 ± 1.52 for 420 g dw of embryogenic cultures, and 11.80 ± 0.76 for 210 g dw of cell suspensions. Notably, only the leaves and embryogenic cultures showed significant accumulation of osteogenic flavonoids, with apigenin levels of 1.8 ± 0.3 and 0.32 ± 0.02 mg/g dw, and kaempferol levels of 1.0 ± 0.18 and 0.2 ± 0.01 mg/g dw, respectively. Our findings indicate that embryogenic cultures have the potential to produce up to 40% more apigenin in 12 weeks compared to 1 kg of parsley plant, thanks to their superior biomass reproductive competence. These results present a promising biotechnological approach for generating extracts enriched with bioactive compounds, which can be further utilized in in vitro osteogenic testing.Keywords
Regeneration is a complex process that involves the replacement of lost tissue through the proliferation of specialized cells. While human regenerative capacity is naturally limited to certain tissues, such as bone, the field of Regenerative Medicine (RM) aims to push the boundaries of tissue regeneration by harnessing the potential of cell-based therapies and bioactive compounds. In the realm of Bone Tissue Engineering (BTE), there has been a growing interest in exploring the therapeutic potential of bioactive compounds derived from plant extracts. These compounds offer favorable attributes that could enhance therapeutic efficacy and reduce dosage requirements, thereby minimizing the risk of toxicity and side effects associated with conventional pharmacological agents. Natural compounds, including phytoestrogens with estrogenic effects (e.g., genistein, daidzein, icariin, dioscin, Ginkgo biloba), antioxidant and anti-inflammatory agents (e.g., acteoside, curcumin, resveratrol, Camellia sinensis), treatments that exert their effects by multiple actions (e.g., kinsenoside, berberine, Olea europaea, Prunus domestica, Allium cepa) could provide a safer alternative to primary pharmacological strategies [1–4].
Among these bioactive compounds, flavonoids have attracted considerable attention due to their remarkable biological and osteogenic activity. Flavonoids have demonstrated the ability to promote bone deposition while inhibiting bone resorption through their antioxidative properties and regulation of bone homeostasis. Recent studies utilizing plant extracts have unveiled a plethora of flavonoids that exhibit noteworthy effects on bone metabolism, including apigenin, luteolin, corylin, daidzein, kaempferol, puerarin, genistein, equol, clathrin, calycosin, formononetin, icariin, quercetin, rutin, hesperidin, among others [2,5–8].
P. crispum, commonly known as parsley, is an herb belonging to the family Apiaceae or Umbelliferae, originating from the Mediterranean but now cultivated worldwide. It contains several important chemical components, including flavonoids, apiol, phytol, essential oils, coumarins, and petroselinic acid. It has diuretic, anti-inflammatory, antioxidant, antiplatelet, antiurolithiasis, anticancer, anti-aging properties, and can decrease the risk of cardiovascular disease [9–11].
Parsley leaves (PL) contain significant amounts of phenolic compounds, which are present as aglycones (flavones and flavonols) and glycosides. Recent studies have identified up to twenty-nine flavonoid glycosides, with the majority of phenolic compounds corresponding to the glycoside apiin, which hydrolyzes to become the flavone apigenin. The aglycones kaempferol, luteolin, chrysoeriol, isorhamnetin, and quercetin are also present, albeit in significantly lower concentrations [11,12]. Furthermore, recent studies have investigated the isolated use of some of these mentioned flavonoids in preosteoblastic cell cultures, highlighting apigenin, quercetin, and kaempferol due to their specific regulatory effects. Apigenin increases alkaline phosphatase (ALP) activity, an early marker of osteoblastic (OB) differentiation, and enhances osteocalcin (OCN) gene expression in these cells, a later marker of osteoblastic differentiation. Quercetin has positive pharmacological effects on bone. Its administration in primary bone marrow mesenchymal stem cells (BMSCs) increased osteoblast differentiation through the upregulation of alkaline phosphatase (ALP), collagen-1A1 (Col-1A1), and osteocalcin (OCN) expression. It exerts its effects by enhancing mitogen-activated protein kinase (MAPK) activity, leading to the expression and activation of RUNX-2. The bone regeneration effects of kaempferol are mediated through the regulation of estrogen receptors, bone morphogenetic protein-2 (BMP-2), nuclear factor-kappa B (NF-κB), MAPK signaling pathways, and the mammalian target of rapamycin (mTOR). In summary, these compounds exhibit beneficial effects on bone cells, making them promising alternatives for bone regeneration treatments. In summary, these compounds exhibit beneficial effects on bone cells, making them promising alternatives for bone regeneration treatments. However, more clinical studies are needed to confirm their efficacy and safety in humans [2,13].
However, plants are generally unable to produce enough of these compounds, known as secondary metabolites (SMs), to meet the global demand for them in the field of health. In order to address this challenge, numerous publications in the 1980s focused on studying the cellular organization, differentiation, and synthesis of SMs in in vitro cultures. In particular, researchers isolated and identified twenty-four different flavonoid glycosides from cell suspension cultures of illuminated parsley cells (Petroselinum hortense). The identified aglycones included flavones such as apigenin, luteolin, chrysoeriol, and flavonols such as quercetin and isorhamnetin [14–16]. These studies demonstrated the advantages of the totipotent phenomenon in plant biology. However, it should be noted that the balance between primary metabolites and SMs in these cultures is dynamic and mainly influenced by factors such as growth, age, auxins, and the composition of the growth medium. Additionally, there is another process, commonly referred to as “embryogenesis”, which leads to the formation of specialized cells, tissues, organs, and ultimately the entire plant, all exhibiting the genetic makeup of somatic cells. Differentiated cultures undergoing embryogenesis can follow a similar morphogenetic pathway as the development that occurs in vivo, providing a cost-effective approach for the production of lead flavonoids [9,17,18]. Despite this, no studies have reported the accumulation of flavonoids using these cultures, even though data on the accumulation of secondary metabolites in plant cell cultures are crucial for achieving milestones in the targeted production of SMs and plants with recognized biomedical activities [17,19,20].
The present work aims to clarify the differences in total flavonoid content (TFC), composition, and accumulation between parsley’s cell suspension culture (CSC), embryogenic culture (EC), and parsley leaves, particularly focusing on the aglycones apigenin, quercetin, and kaempferol flavonoids. The findings from this research endeavor hold great potential in shedding light on the prospective applications of these compounds in future studies involving osteoblastic cells.
2.1 Plant and Cultures Material
2.1.1 Cell Suspension Cultures (CSC) Induction
Root explants were cut from germinated vitroplants of P. crispum with an incubation time of 4 weeks. The explants were carefully excised and transferred to 250 mL Erlenmeyer flasks containing 40 mL of liquid MS medium supplemented with 1 mL/L of 2,4-dichlorophenoxyacetic acid (2,4-D) from a stock solution of 3.91 mM, along with 3% sucrose. The flasks were closed with double-layer aluminum lids and left in constant agitation at 150 rpm in an incubation environment with natural light photoperiods at a temperature of 24°C ± 1°C.
The suspension cells obtained from the first culture were transferred to a new medium every two weeks, and the cultures were tested for vitality using the Widholm methodology and fluorescein diacetate (FDA) [21].
2.1.2 Embryogenic Cultures (EC) Induction
For the induction of somatic embryogenesis, a flask containing cells after 10 days of incubation was selected as a sample and filtered using sterile materials in a laminar flow hood. Once the cells were collected, 4 mL were transferred to flasks with 40 mL without hormones addition medium (HAM). The flasks were continuously agitated at 150 rpm at a temperature of 24°C ± 1°C in an environment with continuous light at 100 µmol m−2.
Once the first flask with differentiated cells was obtained, reseeding continued every 8 to 15 days according to the culture’s needs. The supernatant was recovered between each reseeding and kept in the same conditions mentioned above. The growth and development of new embryogenic cultures took up to 21 days. The samples were collected, frozen at −20°C for 24 h for lyophilization, and stored in empty dries for further extraction.
2.2 Plant Leaves (PL), Standards and Reagents
To prepare the plant leaves, 1 kg of parsley (P. crispum) was purchased from a local supermarket in Santa Anita, Tlaquepaque, Jalisco, Mexico. The leaves were carefully dislodged, washed, disinfected, and placed on absorbing paper for 4 h. They were subsequently frozen at −20°C for 24 h for lyophilization and stored in a desiccator dryer for further extraction.
The apigenin (A), quercetin (Q), and kaempferol (K) 95% standards were purchased from Sigma-Aldrich, Merck, Mexico. Methanol, toluene, acetic acid, diethyl ether, chloride acid, distilled water, aluminum chloride, acetonitrile analytical, and HPLC grade were acquired from the Instrumental Analysis Laboratory of National Technological Institute of Mexico, Tlajomulco de Zuñiga Campus.
2.3 Parsley Leaves, Cell Suspension and Embryogenic Cells Cultures Extractions
The extraction and hydrolysis used the methodology described by Delgado Flores [12] with some modifications. One gram of different samples (in triplicate) was added to boiling distilled water at a ratio of 1:15 (w/v). It was then mixed for 1 min in the vortex (Diagger, Scientific Industries) and filtered using paper No. 1. Subsequently, half of the recovered extract was used, and 2 M HCl was added at a ratio of 1:2 v/v and placed in a boiling water bath for 45 min. Then, the hydrolyzed extracts were transferred to a container with cold water for 5 min and diluted with distilled water at a ratio of 1:1 v/v. The aglycones were separated using a funnel and diethyl ether at a ratio of 6:2 v/v. The aqueous phase was discarded, and the ether phase was collected. This separation process was carried out in triplicate. The remaining solution was evaporated to dryness and resuspended in 1 mL of HPLC-grade methanol to obtain 1 g/mL concentration.
2.4 Total Flavonoid Content (TFC) Analysis
The aluminum chloride methodology analysis was performed following López-Hidalgo et al. [22] with some modifications. One hundred microliters of each extract were transferred to a 1.5 mL microcentrifuge tube containing 300 μL of methanol and mixed using a vortex. Subsequently, 20 µL of 10% (w/v) aluminum chloride and 20 μL of 1 M potassium acetate were added. The reaction mixture was diluted with 600 μL of methanol, covered, and incubated in the dark at room temperature for 30 min. The blank was prepared by diluting 100 μL of extract in 900 μL of methanol. Then, 150 µL of the samples, standards, and blank were transferred from the test tube to a clear 96-well polystyrene microplate, and the absorbance of each was measured at 415 nm. The calibration curve was constructed using standard quercetin solutions at concentrations of 0.1, 0.2, 0.3, 0.4 and 0.6 mg/mL. To ensure accuracy and reproducibility, three replicates were performed for the calibration curve and three replicates for each sample. The total flavonoid content was then expressed as milligrams of quercetin equivalent per gram of dry weight (mg/g dw).
2.5 Thin-Layer Chromatography (TLC) Analysis
For TLC analysis, 3 µL of apigenin, quercetin, and kaempferol (0.25 mg/mL) were placed on silica gel plates 60 F254 (Merck Millipore Corporation, Germany), along with 3 µL of PL extracts (1 g/20 mL dw), and 1 and 0.5 µL (1 g/mL dw) of EC and CSC extracts, respectively. The distance between the spots was 0.9 cm from the shores and 0.8 cm from the side of the plate. The run limit was 1 cm from the top edge. Two running times with a mobile phase of toluene, methanol, and acetic acid in two different ratios, 10:4:1 v/v/v and 12:2:1 v/v, respectively, were used. After the run, the plate was allowed to dry at room temperature, and fractions were analyzed and identified using a UV transilluminator (DyNA Dual-Intensity UV transilluminator, Labnet Company, USA) at 254 nm. The reactive (NP-PEG) was sprayed to determine phenolic compounds, and the plate was observed in a UV transilluminator.
For identification and quantification analysis, a Thermo Fischer® HPLC system consisting of a High-Performance Anionic Exchange Chromatography-Pulsed Amperometry Detector coupled to a diode array detector (DAD) was used, with a Gemini-NX C18 Column (150 mm × 4.60 mm) Phenomenex®. Elution was done in a gradient system with A) water acidified with acetic acid 97:3 and B) acetonitrile, starting with 10% B that increased to 90% for 2 to 15 min. The injection volume was 15 μL with a flow rate of 1 mL/min, and the column temperature was kept at 30°C. Two wavelengths (337 and 363 nm) were used to detect the flavonoids. Calibration curves were prepared using apigenin, kaempferol, and quercetin 95% standards (Sigma-Aldrich, Germany) dissolved in HPLC grade MeOH at six concentrations (µg/mL): 0.125, 0.25, 0.50, 1.00, 2.00, 10.00. The hydrolyzed extracts were diluted at 1:300 v/v with the same MeOH.
The results are presented as mean ± standard deviation of at least three independent experiments. Statistical differences were analyzed using ANOVA followed by Tukey post-test with a p-value of 0.05, using IBM SPSS statistic 21.0.0.0 version.
3.1 Cell Suspension Cultures Induction
Cell suspension cultures were established after 2 weeks of incubation. Ten subsequent subcultures were performed at intervals of 8 to 15 days. It was in the tenth subculture that a viable cells suspension was obtained, as illustrated in Fig. 1.
Figure 1: Process of obtaining suspension cells from P. crispum roots. (A) P. crispum vitroplant (B) Transfer of initial root explant to liquid medium. (C) Suspension cells in their second and third subculture. (D) Suspension cells in their fourth and fifth subculture. (E) Suspension cells finely distributed in the sixth and seventh subculture. (F) Suspension cell culture without aggregates, finely distributed, and viability test performed with Fluorescein Diacetate (FDA)
3.2 Embryogenic Cultures (EC) Induction
Fig. 2 shows the different stages observed in the embryogenic cultures obtained after 60 days of transfer to without HAM. The microscopic examination revealed the glomerular, heart, torpedo, dicotyledon, and complete plant development stages. Additionally, the observation of bipolar growth ruled out unipolar differentiation, which would have resulted in the development of a single organ type (root, stem, or leaf). Therefore, we ensured that our differentiation was embryogenic in nature, leading to the formation of new in vitro plants with root and shoot structures.
Figure 2: Embryogenic culture of P. crispum at different developmental stages: (A) globular stage, (B) heart-shaped stage, (C) torpedo stage, (D) cotyledonary stage, (E) embryogenic culture obtained at 15 days of incubation
Once the flask reached its maximum capacity with the developed culture containing roots, stems, and leaves, the supernatant was recovered and incubated again in fresh medium. This procedure facilitated the restart of the cell duplication and differentiation cycle, allowing for the maintenance of the embryogenic culture in hormone-free medium with continuous production of vitroplants.
3.3 Total Flavonoid Content (TFC) Determination
Table 1 presents the total flavonoid content of the aqueous hydrolysate extracts, which was calculated using the calibration curve (R² = 0.9885) and expressed as mg of quercetin equivalents per gram of dry weight (QE/g). The results of the ANOVA statistical comparison showed that each extract is significantly different from the others. The extract from PL had the highest flavonoid content, followed by EC and CSC. This study provides a first-ever simultaneous stage comparison and visualization of the differences in flavonoid accumulation in P. crispum
This method of quantification allows for a rapid estimation of the levels of compounds present in various extracts. However, as flavonoids in plants are often found alongside other metabolites and classes, there is no precise method for their simultaneous quantification. Therefore, it is important to supplement this information with other methods of identification and quantification such as TLC or HPLC.
3.4 Qualitative Analysis by TLC
Due to the high number of bands present in the PL extract, it was diluted twenty times to allow for adequate separation of the spots. Apigenin, quercetin, and kaempferol were detected in this extract, as well as two unknown flavonoid bands. In contrast, the EC extract only showed apigenin and one additional flavonoid band, while CSC exhibited only a slight apigenin band. The identification of these compounds was confirmed using standard Rf-values and colorimetric changes, which were observed after spraying the NP-PEG reagent (as described in Table 2).
As shown in Fig. 3, the EC appears to accumulate compounds that may serve as precursors for interesting flavonoids not present in PL, while only apigenin was detected in CSC.
Figure 3: Photographs. Qualitative analysis of flavonoids by one dimensional TLC ascendent UV at 254 nm. (A) Comparison of different compositions of extracts parsley leaves (PL), embryogenic cultures (EC) and cell suspension cultures (CSC). Using standards of apigenin (A), quercetin (Q) and kaempferol (K). (B) Detection of flavonoids in each extract using NP-PEG reagent
3.5 Quantitative Analysis by HPLC
The HPLC results revealed the presence of apigenin and kaempferol in all samples, as shown in Fig. 4. However, quantification of quercetin using this method was challenging due to the higher concentration required to obtain a definite peak. Therefore, only some traces of quercetin were detected in parsley leaves samples, as confirmed by the TLC results. The quantification of apigenin and kaempferol is presented in Table 3. Additionally, the accumulation of apigenin and kaempferol in PL and EC showed a similar ratio of 1.8 and 1.6, respectively. These comparative results demonstrate that our EC can produce apigenin and kaempferol without the use of HAM.
Figure 4: Chromatograms of standards and P. crispum extracts at 337 nm. (A) Standards (I) apigenin (II) kaempferol (B) cell suspension culture (C) embryogenic cultures (D) parsley leaves
Note: The terms in parentheses represent the subcategories or specific compounds mentioned.
4.1 Cell Suspension Cultures (CSC) Induction
Cell suspension cultures of parsley have been extensively investigated due to their ability to easily obtain cells using growth hormones. These cultures have been employed to explore various enzymatic pathways [14] and the accumulation of diverse metabolites in the plant [23–25]. However, these previous studies have primarily utilized cell suspension cultures derived from callus, as it ensures reliable production of suspensions through this route. Unprecedented results have been achieved through the direct pathway. This can be attributed primarily to the liquid medium’s ability to directly contact the entire explant (root). Additionally, the agitation speed (60–150 rpm) allows the culture medium to modify the properties of the cell wall, facilitating the separation of cells from one another through the use of growth regulators. This enables the isolation of individual cells, which subsequently adapt to the medium’s presence of salts, minerals, and sugars, leading to the establishment of the initial suspended cells [26].
On the other hand, viability monitoring was employed to assess the state of the suspensions for subsequent embryogenic induction. Notably, a well-established suspension comprises isolated cells, cellular aggregates of varying sizes, residual fragments from the inoculum, and remnants of deceased cells, as depicted in Fig. 1 [26].
It is crucial to emphasize that while the utilization of growth hormones is a decisive factor in the acquisition of cell suspension cultures, it must be duly acknowledged that the response of individual plants to specific hormones or cultivation conditions can diverge significantly owing to their unique metabolic attributes. This inherent variability underscores the necessity for meticulous optimization and tailored strategies in order to attain optimal outcomes within cell suspension culture systems [27].
4.2 Embryogenic Cultures (EC) Induction
The demonstrated adaptability of the cultures after 60 days of transfer to without HAM can be explained by considering the cellular differentiation as a process comprising different phases: a) Competence acquisition phase, b) Induction phase, and c) Realization phase [28]. In other words, only embryos in more advanced stages of histodifferentiation are capable of germination and giving rise to complete plants. The maturation or realization stage is a complex period of somatic embryo development in which cell expansion, accumulation of reserve substances, and acquisition of desiccation tolerance occur [26]. This differentiation is associated with an increase in the production of secondary metabolites, as evidenced by increased cell aggregation, production of specific cell types, development of chloroplasts and green pigmentation, and initiation of more organized structures such as embryos, roots, and shoots [29].
Despite extensive research in this field, the initial signaling process for somatic embryo development remains variable, and the specific pathway or variables initiating it are still unclear. Recent studies have started to unravel the complex networks of interactions between transcription factors, endogenous auxin concentrations, cytokinins, receptors, transporters, and the explant origin, aiming to establish a clear pathway for obtaining somatic embryos [30]. However, this study did not focus on establishing those pathways. Nevertheless, the constant production observed in our study can be explained based on findings from other studies where the presence of embryogenic cells led to the continuous proliferation and formation of proembryogenic masses (PEM). According to these studies, since the auxin required for PEM proliferation is released into the medium, transferring the supernatant restarts the cycle, leading to the reproduction of new embryogenic cells. In contrast, cultures that developed tissues (roots, stems, and leaves) were affected by the concentrations of auxin released, prompting their transfer to fresh medium to facilitate the growth and development of advanced-stage vitroplants (torpedo and dicotyledonous) [31]. Further research on the culture obtained in this study could provide valuable insights into embryogenic cultures of P. crispum, contributing to a deeper understanding of the subject matter.
4.3 Total Flavonoid Content (TFC) Determination
The methodology used in this study is based on the principle that aluminum chloride forms acid-stable complexes with the C-4 keto group and the C-3 or C-5 hydroxyl group of flavones and flavonols. Each flavonoid group has a maximum absorbance in different wavelengths between 305 to 415 nm, depending on their structure [32,33]. It should be noted that only one reading was taken at 415 nm, so the results mostly reflect the flavanols (quercetin, rutin, kaempferol) and a minor group of flavones (tangerine, luteolin, apigenin, diosmetin, among others) [33]. Although this study does not focus on obtaining a complete analysis of each of the components of the extracts, this methodology allows for a global comparison of the medicinal potential of each extract, mainly based on the presence of flavanols, which are known to confer antioxidant properties and medicinal benefits, such as antibiotic, anti-inflammatory, anti-cancer, and anti-allergic properties [11].
Table 1 demonstrates that the total flavonoid content (TFC) in parsley leaves (PL) was 3.5 times higher compared to a comparative study utilizing hydrolyzed extracts of parsley leaves, which reported 15.05 ± 2.20 mg quercetin equivalents/g (QE) [11]. This result can be attributed to two factors. Firstly, the extraction methodologies used were based on different sample conditions. Using dried material for extraction may result in a better obtaining of flavonoids compared to fresh leaves, which are fragile and tend to deteriorate faster than dried samples, as supported by the results we observed [34]. Secondly, commercial parsley obtained from different countries may have variations in their yield and composition due to geographical, seasonal and environmental factors. In contrast, in vitro cultures offer an advantage of a defined production system, ensuring a continuous supply of products with uniform quality and yield, albeit with lower concentrations as observed in our results [35].
On the other hand, it was observed that embryogenic cultures exhibited a significantly higher TFC compared to the previously mentioned leaf study, while the cell suspension cultures showed TFC values in close proximity. Due to the primary objective of this study being the initial comparative analysis of flavonoid production among various cultures, the cultures were predominantly optimized for biomass production rather than flavonoid accumulation. However, it is widely recognized in plant cell culture research that various factors, including the composition of the culture medium, light intensity, and photoperiod, play a crucial role in influencing the accumulation of secondary metabolites, such as flavonoids. However, it is widely acknowledged in the field of plant cell culture research that various factors, such as the composition of the culture medium, light intensity, and photoperiod, play a crucial role in influencing the accumulation of secondary metabolites, including flavonoids. Therefore, while the specific aim of this study does not revolve around increasing flavonoid production, it is important to acknowledge the significant impact of these factors on flavonoid accumulation. Moreover, the establishment of a comprehensive strategy to efficiently recover and purify flavonoids from the culture medium has significant potential in terms of optimizing the process and enhancing economic viability [35].
Therefore, through further optimization of the culture conditions in each specific cultivation system, an enhancement in flavonoid production can be achieved in embryogenic and cell suspension cultures of P. crispum in future studies. Overall, these findings underscore the significance of meticulously selecting extraction methodologies and emphasize the potential of in vitro parsley cultures as a promising alternative for flavonoid production. Furthermore, they emphasize the necessity of conducting additional research to optimize their application in commercial settings.
4.4 Qualitative Analysis by TLC
The application of TLC facilitated the identification of distinct flavonoid compounds present in the extracts, as depicted in Fig. 3. Hydrolysis treatment played a crucial role in obtaining aglycone-type compounds, enabling their comparison and identification against established standards. The isolation of flavonoids from the sample matrix represents a pivotal step in any analytical scheme. As demonstrated by the results obtained, EC exhibit a greater biosynthetic similarity to leaves and the ability to produce and accumulate higher concentrations of specific metabolites, which are biosynthesized in individual plant tissues in contrast to CSC [36]. Furthermore, it is evident that the process of cellular differentiation led to an increase in flavonoid production and the emergence of other bioactive compounds, including potentially chlorophylls, carotenoids, phenols, non-flavonoids, and vitamin C [37].
The findings of the TLC analysis corroborated the previous TFC results, demonstrating that parsley cultures of varying developmental stages exhibited different quantities of flavonoids. The production and accumulation of flavonoids such as apigenin, quercetin, or kaempferol in the cultures were influenced by the cellular developmental stage (cellular, embryogenic, or vegetative) and the underlying metabolic pathways. Notably, suspension-induced cell differentiation led to the formation of organized structures, such as meristemoids/embryos, which subsequently matured into organ cultures or complete plants. This process triggered metabolic alterations, resulting in enhanced flavonoid production by mimicking the secondary metabolic pathways found in the whole plant [38,39].
While this comparative analysis enables the rapid, effective, and clear acquisition of results, it carries substantial implications from a commercial standpoint, as it aids in determining whether in vitro cultures provide a favorable alternative to traditional cultivation methods, particularly in terms of generating novel compounds with significant pharmaceutical value that may be absent or produced in lower quantities in the parent plant [35,40].
4.5 Quantitative Analysis by HPLC
From the results obtained by HPLC, several points can be emphasized that provide relevant information from a biomedical perspective for CSC and CE extracts. Although CSC offers a lower concentration of apigenin than leaves and embryogenic cultures, this culture was initially studied and generated using callus and Hormone Addition Medium (HAM) by Harborne in 1972, where the synthesis of flavonols and flavones by cell suspension cultures of P. crispum was detected for the first time, demonstrating that it is dependent on light. It was not until 1999 that Hamplet et al. quantified flavonoid aglycones from an illuminated parsley cell suspension culture and identified and quantified apigenin, luteolin, and chrysoeriol as flavones and kaempferol, quercetin, and isorhamnetin as flavonols, represented as a percentage (kaempferol 3%, quercetin 20%, isorhamnetin 22%, apigenin 17%, luteolin 1%, and chrysoeriol 37%) [15,16]. Based on these results, we can infer that the initial production of apigenin and kaempferol in this culture is not necessarily conditioned by the induction origin of the culture (callus). Furthermore, this culture is capable of producing kaempferol and apigenin in inverse quantities compared to EC and PL. This can be explained by the fact that these types of cultures tend to experience higher oxidative stress due to their conditions and the metabolic pathways associated with the production of flavonols (kaempferol) or flavones (apigenin) depend on the oxidative stress conditions in which the culture is in at a given stage. Therefore, in the case of CSC, the cells show the presence of flavonols that are more reactive, leading cells to produce compounds that reduce the presence of reactive oxygen species (ROS), such as kaempferol [41]. However, as mentioned previously, although this study does not focus on evaluating the stimulation or potentiation of flavonoid production, such as apigenin, quercetin, and kaempferol, studies conducted with suspension cultures of other plants have shown that the effect of cytokinins and auxins found in the MS medium plays an essential role in the stimulation of different flavonoids [40]. Therefore, CSC offers an interesting alternative for future studies since it could become a key source of production biomedicinal compounds, such as rosmarinic acid in cell lines of Coleus blumei, ginsenosides in cell cultures of Panax ginseng, raucaffricin in cell lines of Rauvolfia serpentina, or currently, the best-known example, the production of paclitaxel by Phyton [42]. Today, various strategies can be used, including designing an appropriate bioreactor system, selecting highly productive lines, optimizing nutrient medium, elicitation, metabolic engineering, two-phase cultivation, plant cell immobilization, molecular approaches, and nutritional management studies [36].
On the other hand, the comparison of concentrations observed between PL and EC suggests a close relationship between compound accumulation and plant development, particularly in the leaves. This accumulation is predominantly influenced by specific variations in signaling molecules, amino acids, proteins, and primary and secondary metabolites that are dependent on tissue development [7]. Additionally, it was observed that the accumulation ratio of apigenin and kaempferol in both samples remained consistent (1.8 ± 0.2), indicating that the in vitro genetic variation in plant cell culture did not have an impact on this characteristic. This observation is particularly relevant considering that somaclonal variation, which is well-known to occur during multiple subculture cycles prior to embryogenesis, can potentially affect the accumulation of these compounds [43].
However, despite the significant difference in flavonoid accumulation between EC and PL, the consistent procurement of embryogenic culture at the laboratory level provides scalability advantages over traditional culture methods offering an advantage in terms of production time compared to traditional cultivation. Table 3 presents a comparative analysis of flavonoid production using EC and PL extracts, demonstrating significant differences in yield between the two. For instance, while obtaining 1 kg of parsley leaves requires a 12-week waiting period until harvest, the embryogenic culture remains constant and reproductive during this period, resulting in up to 40% more apigenin production. From this perspective, it is highly relevant to emphasize the economic and commercial implications of this study, as flavonoids derived from plant extracts are in high demand in the pharmaceutical industry and are expected to play a fundamental role in market growth. According to a report, the global flavonoids market reached a value of $1,497.7 million in 2020 and is projected to reach $2,717.8 million by 2030, with a compound annual growth rate (CAGR) of 6.2% between 2021 and 2030 [18]. Thus, these findings underscore the lucrative potential of this field and offer a promising outlook for stakeholders in the pharmaceutical industry.
Regarding the concentrations of apigenin detected in PL, it has been determined that the non-centrifugation process and hydrolysis time play a crucial role in achieving high levels of metabolites, especially carbohydrates like polysaccharides (apiin) [11,12,44]. However, studies using various extraction methodologies, times, and acid concentrations in hydrolysis have reported higher amounts of apigenin (9.50 ± 1.91 mg/g dry weight) [12], while others have reported lower amounts (185 ± 65 mg/100 g fresh weight) [36] and 510–630 mg/100 g fresh weight [45,46].
On another note, the presence of flavonoids such as luteolin, chrysoeriol, kaempferol, quercetin, and isorhamnetin in parsley plants has generated controversy in the scientific literature. This confusion may arise from variations in isolation techniques and the age of the plant material used in different laboratories [38]. Consequently, the selection of the methodology depends on the specific objectives of the extract, as the type and concentration of the desired flavonoids obtained will vary accordingly.
Furthermore, based on the preliminary analysis of the extracts using TLC, it was observed that the selected HPLC methodology with the specified wavelengths did not allow the identification of other flavonoids. This suggests that these compounds possess structures different from the flavone and flavonol groups, possibly belonging to other categories such as biflavonoids (e.g., ginkgetin), prenyl-flavonoids, flavonolignans (e.g., silybin), glycosides, flavonoid esters, chalcones, and proanthocyanidins [47]. The above is relevant, as it is known that plant extracts have the ability to act synergistically, giving them the potential to be bioactive at lower doses and with a lower risk of side effects [48]. An example of this was reported in a recent comparative study in in vivo arthritis models, where methanolic extracts of wild Buddleja cordata and its cell cultures showed significant effects on body weight gain without causing lethality, as well as a decrease in serum concentrations of total cholesterol, HDL, and LDL. It is worth noting that the decrease in LDL was observed only with the administration of the cell culture extract for 28 days, while the extracts from the leaves of the wild plant reduced the concentration of serum alkaline phosphatase [49].
In relation to the results obtained in the study, no previous investigations comparing the effect of parsley extract on bone regeneration have been conducted. However, existing evidence on the activity of flavonoids such as apigenin and kaempferol, present in the analyzed extracts, has demonstrated their ability to stimulate osteoblast activity, suggesting their potential use as a viable alternative [13,19,20,50,51]. It is important to consider that the complexity of the chemical composition and the underlying mechanisms responsible for the biomedical effects of plant extracts often involve complex synergistic interactions, posing a challenge in ongoing research [51,52]. Therefore, the examination and evaluation of the composition of each extracted sample facilitate the exploration of plant cell cultures as a viable alternative to leaf extracts. Specifically, the utilization of EC at a specific developmental stage presents an opportunity to generate and accumulate intermediate levels of flavonoids, bridging the gap between PL and CSC extracts. This avenue of investigation holds promise for advancing research and developing novel approaches in the field.
The utilization of bioactive natural products derived from plants for sustainable and environmentally friendly medicinal applications has emerged as a rising trend in modern medicine, particularly in the fields of Regenerative Medicine (RM) and Bone Tissue Engineering (BTE). In this pioneering study in the field of plant biotechnology, we have conducted the identification of compounds with high pharmaceutical value by employing innovative approaches such as suspension cell culture and the induction of parsley (Petroselinum crispum) embryogenic cultures from root explants. Our findings reveal significant variations in flavonoid production depending on the type of culture utilized.
Embryogenic cultures have shown a clear advantage in biomass and flavonoid production, particularly with apigenin and kaempferol, surpassing traditional cultures in both yield and time efficiency. Furthermore, this study represents the first attempt to compare extracts obtained from different stages of parsley plant cells, thereby opening new avenues for future in vitro investigations evaluating the potential of these extracts in biomedical applications. These findings provide a promising and stimulating perspective for the development of more effective and environmentally friendly therapies in the realms of regenerative medicine and bone tissue engineering.
Acknowledgement: Acknowledgments are extended to the Plant Biotechnology, Instrumental Analysis and Plant Biochemistry Laboratories at the Technological Institute of Tlajomulco, Jalisco, as well as the Analytical Laboratory at the Technological Institute of Tuxtla Gutiérrez, for providing the various solvents and equipment essential for conducting this research. We also express our gratitude to the National Council of Science and Technology (CONACYT) for their scholarship support.
Funding Statement: This study was supported by financial assistance from Smart Biotechnology S.A. de C.V. We gratefully acknowledge their contribution and support in the form of funding for this research project.
Author Contributions: Martha Alicia Rodríguez Mendiola conceptualized the study’s design and provided the in vitro parsley cultures. Carlos Arias Castro conducted the experiments and the instrumental analysis, statistical analysis and interpretation. Laura Isabel Arias Rodríguez participated in the work conception, designed and carried out all the experiment work, including data analysis, wrote the manuscript, and interpreted it with Carlos Arias Castro. María Celina Lújan Hidalgo and Alejandra Mancilla Margalli performed the HPLC laboratory analyses. Federico Gutiérrez Miceli, Diana Reséndez Pérez, Juan José Villalobos Maldonado and Carlos Arias Castro revised and gave the final approval of the article version to be published.
Availability of Data and Materials: The data generated and/or analyzed during the current study are accessible upon request from the corresponding author.
Ethics Approval: The standard procedures and appropriate precautions were followed to ensure personnel safety and minimize environmental impacts, including the reduction of waste generation and the prevention of water contamination.
Conflicts of Interest: The authors declare that they have no conflicts of interest to report regarding the present study.
References
1. Vallet-Regi, M., Salinas, A. J. (2021). Mesoporous bioactive glasses for regenerative medicine. Materials Today Bio, 11, 100121. [Google Scholar] [PubMed]
2. Bellavia, D., Dimarco, E., Costa, V., Carina, V., de Luca, A. et al. (2021). Flavonoids in bone erosive diseases: Perspectives in osteoporosis treatment. Trends in Endocrinology & Metabolism, 32(2), 76–94. [Google Scholar]
3. Gupta, T., Das, N., Imran, S. (2019). The prevention and therapy of osteoporosis: A review on emerging trends from hormonal therapy to synthetic drugs to plant-based bioactives. Journal of Dietary Supplements, 16(6), 699–713. [Google Scholar] [PubMed]
4. Martiniakova, M., Babikova, M., Omelka, R. (2020). Pharmacological agents and natural compounds: Available treatments for osteoporosis. Journal of Physiology and Pharmacology, 71(3), 307–320. [Google Scholar]
5. Ranjith, R. (2020). Prospects of polycaprolactone-based nanocomposite porous scaffolds for sustained release of flavonoids in bone tissue engineering. International Journal for Research in Applied Science and Engineering Technology, 8(11), 919–928. [Google Scholar]
6. Saquib, M., Hussain, M. K., Negi, D. S., Khan, M., Swamy, M. K. (2020). Bioflavonoids as promising antiosteoporotic agents. In: Plant-derived bioactives. Singapore: Springer. [Google Scholar]
7. Swamy, M. K. (2020). Plant-derived bioactives production, properties and therapeutic applications. Singapore: Springer. [Google Scholar]
8. Miranda, L. L., de Paula Guimarães-Lopes, V., Altoé, L. S., Mariáurea Matias, S., Fabiana Cristina Silveira Alves, M. et al. (2019). Plant extracts in the bone repair process: A systematic review. Mediators of Inflammation, 2019, 1–22. [Google Scholar]
9. Reyes-Munguía, A., Zavala-Cuevas, D., Alonso-Martínez, A. (2012). Parsley (Petroselinum crispumChemical composes and applications. Tlatemoani: Revista Académica de Investigación, 11, 216–233. [Google Scholar]
10. Agyare, C., Appiah, T., Boakye, Y. D., Apenteng, J. A. (2017). Petroselinum crispum: A review. In: Medicinal spices and vegetables from Africa. Dschang, Cameroon: Elsevier. [Google Scholar]
11. Mara de Menezes, N., Rykiel, L., Falcão dos Santos, K., Soares, P., Kachlicki, P. et al. (2020). Chemical characterization and antioxidant activity in vivo of parsley (Petroselinum crispum) aqueous extract. Food & Function, 11(6), 5346–5356. [Google Scholar]
12. Delgado, M. (2015). Characterisation and quantification of flavonoids in two petroselinum species with termical treatments (Master). Universidad Autónoma de Nuevo León, México. [Google Scholar]
13. Wong, S. K., Chin, K. Y., Ima-Nirwana, S. (2019). The osteoprotective effects of kaempferol: The evidence from in vivo and in vitro studies. Drug Design, Development and Therapy, 13, 3497–3514. [Google Scholar] [PubMed]
14. Kreuzaler, F., Hahlbrock, K. (1973). Flavonoid glycosides from illuminated cell suspension cultures of Petroselinum hortense. Phytochemistry, 12(5), 1149–1152. [Google Scholar]
15. Hahlbrock, K. (1972). Malonyl coenzyme a: Flavone glycoside malonyl-transferase from illuminated cell suspension cultures of parsley. FEBS Letters, 28(1), 65–68. [Google Scholar] [PubMed]
16. Hempel, J., Pforte, H., Raab, B., Engst, W., Böhm, H. et al. (1999). Flavonols and flavones of parsley cell suspension culture change the antioxidative capacity of plasma in rats. Nahrung, 43(3), 201–204. [Google Scholar] [PubMed]
17. Swamy, M. K. (2020). Plant-derived bioactives chemistry and mode of action. Singapore: Springer. [Google Scholar]
18. Research and Markets the World’s Largest Market Research Store (2021). Flavonoid market by product type, form, application: Global opportunity analysis and industry forecast, 2021–2030. https://www.researchandmarkets.com/reports/5521637/flavonoid-market-by-product-type-form#tag-pos-2(2022) [Google Scholar]
19. Salehi, B., Venditti, A., Sharifi-Rad, M., Kręgiel, D., Sharifi-Rad, J. et al. (2019). The therapeutic potential of apigenin. International Journal of Molecular Sciences, 20(6), 1305. [Google Scholar] [PubMed]
20. Sharma, A. R., Nam, J. S. (2019). Kaempferol stimulates WNT/β-catenin signaling pathway to induce differentiation of osteoblasts. The Journal of Nutritional Biochemistry, 74, 108228. [Google Scholar] [PubMed]
21. Widholm, J. M. (1972). The use of fluorescein diacetate and phenosafranine for determining viability of cultured plant cells. Stain Technology, 47(4), 189–194. [Google Scholar] [PubMed]
22. López-Hidalgo, C., Meijón, M., Lamelas, L., Valledor, L. (2021). The rainbow protocol: A sequential method for quantifying pigments, sugars, free amino acids, phenolics, flavonoids and MDA from a small amount of sample. Plant Cell and Environment, 44(6), 1977–1986. [Google Scholar]
23. Flodrová, D., Dzúrová, M., Lišková, D., Ait Mohand, F., Mislovičová, D. (2007). Pectate hydrolases of parsley (Petroselinum crispum) roots. Zeitschrift für Naturforschung C, 62(5–6), 382–388. [Google Scholar]
24. López, M. G., Sánchez-Mendoza, I. R., Ochoa-Alejo, N. (1999). Compartive study of volatile components and fatty acids of plants and in vitro cultures of parsley (Petroselinum crispum (Mill) Nym ex Hill). Journal of Agricultural and Food Chemistry, 47(8), 3292–3296. [Google Scholar]
25. Reil, G., Berger, R. G. (1996). Elicitation of volatile compounds in photomixotrophic cell culture of Petroselinum crispum. Plant Cell, Tissue and Organ Culture, 46, 131–136. [Google Scholar]
26. Freire, M. (2003). Basic aspects of somatic embryogenesis. Biotecnología Vegetal, 3(4), 195–209. [Google Scholar]
27. Chattopadhyay, S., Farkya, S., Srivastava, A. K., Bisaria, S. (2002). Bioprocess considerations for production of secondary metabolites by plant cell suspension cultures. Biotechnology and Bioprocess Engineering, 7(3), 138–149. [Google Scholar]
28. Klerk, G. J., Arnholdt-Schmitt, B., Lieberei, R., Neumann, K. H. (1997). Regeneration of roots, shoots and embryos: Physiological, biochemical and molecular aspects. Biologia Plantarum, 39(1), 53–66. [Google Scholar]
29. Orozco, F., Hoyos, R., Arias, M. E. (2002). Cultivation of plant cells in bioreactors: A potential system for the production of secondary metabolites. Revista Facultad Nacional de Agronomía Medellín, 55(1), 1473–1495. [Google Scholar]
30. Méndez-Hernández, H. A., Ledezma-Rodríguez, M., Avilez-Montalvo, R. N., Juárez-Gómez, Y., Skeete, A. et al. (2019). Signaling overview of plant somatic embryogenesis. Frontiers in Plant Science, 10, 77. [Google Scholar]
31. Yang, X., Zhang, X. (2010). Regulation of somatic embryogenesis in higher plants. Critical Reviews in Plant Sciences, 29(1), 36–57. [Google Scholar]
32. Chang, C. C., Yang, M. H., Wen, H. M., Chern, J. C. (2020). Estimation of total flavonoid content in propolis by two complementary colometric methods. Journal of Food and Drug Analysis, 10(3). [Google Scholar]
33. Huang, R., Wu, W., Shen, S., Fan, J., Chang, Y. et al. (2018). Evaluation of colorimetric methods for quantification of citrus flavonoids to avoid misuse. Analytical Methods, 10(22), 2575–2587. [Google Scholar]
34. Azwanida, N. N. (2015). A review on the extraction methods use in medicinal plants, principle, strength and limitation. Medicinal & Aromatic Plants, 4(3), 196–202. [Google Scholar]
35. Ramachandra, S., Ravishankar, G. A. (2002). Plant cell cultures: Chemical factories of secondary metabolites. Biotechnology Advances, 20(2), 101–153. [Google Scholar]
36. Marchev, A. S., Yordanova, Z. P., Georgiev, M. I. (2020). Green (cell) factories for advanced production of plant secondary metabolites. Critical Reviews in Biotechnology, 40(4), 443–458. [Google Scholar] [PubMed]
37. Waksmundzka-Hajnos, M., Sherma, J., Kowalska, T. (2008). Application of TLC in the isolation and analysis of flavonoids. In: Thin layer chromatography in phytochemistry. Boca Raton: CRC Press. [Google Scholar]
38. Heller, W., Egin-Bühler, B., Gardiner, S., Knobloch, K. H., Matern, U. et al. (1979). Enzymes of general phenylpropanoid metabolism and of flavonoid glycoside biosynthesis in parsley: Differential inducibility by light during the growth of cell suspension cultures. Plant Physiology, 64(3), 371–373. [Google Scholar] [PubMed]
39. Ramawat, K. G., Ekiert, H. M., Goyal, S. (2021). Plant cell and tissue differentiation and secondary metabolites: Fundamentals and applications. Switzerland: Springer Cham. [Google Scholar]
40. Shilpashree, H. P., Ravishankar, R. (2009). In vitro plant regeneration and accumulation of flavonoids in Hypericum mysorense. International Journal of Integrative Biology, 8(1), 43–49. [Google Scholar]
41. Agati, G., Azzarello, E., Pollastri, S., Tattini, M. (2012). Flavonoids as antioxidants in plants: Location and functional significance. Plant Science, 196, 67–76. [Google Scholar] [PubMed]
42. Heine-Dobbernack, E., Kiesecker, H., Schumacher, H. M., Reed, B. M. (2008). Cryopreservation of dedifferentiated cell cultures. In: Plant cryopreservation: A practical guide. New York: Springer. [Google Scholar]
43. Bourgaud, F., Gravot, A., Milesi, S., Gontier, E. (2001). Production of plant secondary metabolites: A historical perspective. Plant Science, 161(5), 839–851. [Google Scholar]
44. Martínez, A. (2020). Chemical of natural products. Colombia: Universidad de Antioquía. [Google Scholar]
45. Justesen, U., Knuthsen, P., Leth, T. (1998). Quantitative analysis of flavonols, flavones, and flavanones in fruits, vegetables and beverages by high-performance liquid chromatography with photo-diode array and mass spectrometric detection. Journal of Chromatography A, 799(1–2), 101–110. [Google Scholar] [PubMed]
46. Justesen, U., Knuthsen, P. (2001). Composition of flavonoids in fresh herbs and calculation of flavonoid intake by use of herbs in traditional Danish dishes. Food Chemistry, 73(2), 245–250. [Google Scholar]
47. Brodowska, K. M. (2017). Natural flavonoids: Classification, potential role, and application of flavonoid analogues. European Journal of Biological Research, 7(2), 108–123. [Google Scholar]
48. Eibl, R., Meier, P., Stutz, I., Schildberger, D., Hühn, T. et al. (2018). Plant cell culture technology in the cosmetics and food industries: Current state and future trends. Applied Microbiology and Biotechnology, 102(20), 8661–8675. [Google Scholar] [PubMed]
49. Gutiérrez-Rebolledo, G. A., Estrada-Zúñiga, M. E., Garduño-Siciliano, L., García-Gutiérrez, G., Reséndiz, C. et al. (2019). In vivo anti-arthritic effect and repeated dose toxicity of standardized methanolic extracts of Buddleja cordata Kunth (Scrophulariaceae) wild plant leaves and cell culture. Journal of Ethnopharmacology, 240, 111875. [Google Scholar]
50. Abdal, T. (2015). Efectos de la aplicación tópica de melatonina y apigenina en la aceleración de la formación ósea en procesos de osteointegración implantológica (Tesis doctoral). Universidad de Murcia, España. [Google Scholar]
51. Jiang, L., Liu, Z., Cui, Y., Shao, Y., Tao, Y. et al. (2019). Apigenin from daily vegetable celery can accelerate bone defects healing. Journal of Functional Foods, 54, 412–421. [Google Scholar]
52. Tang, E. L., Rajarajeswaran, J., Fung, S., Kanthimathi, M. (2015). Petroselinum crispum has antioxidant properties, protects against DNA damage and inhibits proliferation and migration of cancer cells. Journal of the Science of Food and Agriculture, 95(13), 2763–2771. [Google Scholar] [PubMed]
Cite This Article
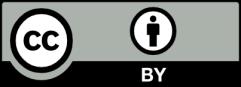