Open Access
ARTICLE
Application of Plant Growth-Promoting Bacteria as an Eco-Friendly Strategy for Mitigating the Harmful Effects of Abiotic Stress on Plants
1 Social Studies Department, College of Arts, King Faisal University, Al-Ahsa, 31982, Saudi Arabia
2 Department of Agribusiness and Consumer Sciences, College of Agriculture & Food Sciences, King Faisal University, Al-Ahsa, 31982, Saudi Arabia
3 Agribusiness and Consumer Sciences Department, College of Agricultural and Food Sciences, King Faisal University, Al-Ahsa, 31982, Saudi Arabia
4 Date Palm Research Center of Excellence, King Faisal University, Al-Ahsa, 31982, Saudi Arabia
5 Department of Family and Community Medicine, College of Medicine, Taibah University, Al-Madinah Al-Munawara, 42353, Saudi Arabia
6 Biology Department, University College of Tayma, University of Tabuk, Tabuk, Saudi Arabia
7 Department of Biology, College of Science, University of Hail, P.O. Box 2440, Hail, 55476, Saudi Arabia
8 EPCRS Excellence Center, Plant Pathology and Biotechnology Laboratory, Agricultural Botany Department, Faculty of Agriculture, Kafrelsheikh University, 33516, Egypt
* Corresponding Authors: Ahmed Hassan Abdou. Email: ; Khaled Abdelaal. Email:
(This article belongs to the Special Issue: Abiotic and Biotic Stress Tolerance in Crop)
Phyton-International Journal of Experimental Botany 2023, 92(12), 3305-3321. https://doi.org/10.32604/phyton.2023.044780
Received 08 August 2023; Accepted 27 October 2023; Issue published 28 December 2023
Abstract
Plant growth-promoting bacteria (PGPB) play an important role in improving agricultural production under several abiotic stress factors. PGPB can be used to increase crop growth and development through hormonal balance and increase nutrient uptake. The positive effect of PGPB may be due to its pivotal role in morphophysiological and biochemical characteristics like leaf number, leaf area, and stem length. Furthermore, relative water content, chlorophyll content, carotenoids, antioxidant enzymes, and plant hormones were improved with PGPB treatment. Crop yield and yield components were also increased with PGPB treatment in numerous crops. The anatomical structure of plant organs was increased such as lamina thickness, stem diameter, xylem vessel diameter, and number of xylem vessels as well as phloem thickness under treatment with PGPB. Additionally, PGPB can alleviate the negative effects of several abiotic stresses by regulating the antioxidant defense system to scavenge the reactive oxygen species resulting in an improvement of yield production in the stressed plants. Additionally, gene expressions were controlled by calcium ion modulation during secondary messengers that act upon calcium-dependent protein kinase and protein phosphatases. This includes many transcription factors such as MYB, AP2/ERF, bZIP, and NAC which regulate genes related to salinity stress signals. PGPB can demonstrate induction genes of signaling under abiotic stress conditions. This review gives an outline of the PGPB role in alleviating the harmful effects of abiotic factors such as salinity, drought, and heat associated with the improvement of the morpho-physiological and biochemical features especially, leaves and branches number, leaf area, antioxidant compounds, plant hormones, and relative water content.Keywords
Under field or greenhouse conditions, plants are exposed to biotic and abiotic stress factors. Biotic stress factors are caused by living organisms such as fungi [1–3], bacteria [4,5], viruses [6], insects [7–10] and weeds [11–13]. Abiotic stress factors include many stress factors, among them; drought [14–16], heat [17], and salinity [18–20] are the main abiotic stress factors. Drought stress is one of the most harmful abiotic stresses that hinders the growth of stressed plants, especially in arid regions. Under drought conditions, plant height, leaf number, and leaf area were significantly decreased in wheat [16]. Also, physiological characteristics such as chlorophyll a and b concentrations, carotenoids, and relative water content were decreased under drought conditions in several economic plants such as pea plants [21], barley [22], and sugar beet [23]. Under heat stress, the reduction in chlorophyll concentrations, decrease in fatty acids, and increase in cell membrane permeability were recorded [24]. The plants have different mechanisms to cope with these conditions such as the reduction in photosystem II efficiency. Farooq et al. [25] found that the growth and physiological characteristics of chickpea plants such as relative water content, photosynthesis, and activity of antioxidant enzymes were negatively affected under chilling stress. Additionally, salinity is one of the main abiotic stress factors, it is a detrimental stress for many plants all over the world. Salinity stress led to a decrease in the morphological characters in the stressed plants like, faba bean [26] and sweet pepper plants [27]. Under salinity conditions, reactive oxygen species are important signs, superoxide and hydrogen peroxide were significantly augmented in the salt-stressed plants [28,29], furthermore, lipid peroxidation significantly increased in pea plants under salinity conditions. These characteristics were related to oxidative stress in the stressed plants under various stresses.
The use of plant growth-promoting bacteria has become one of the most important strategies to overcome oxidative stress. PGPB naturally exists in various media, the most known genera in the agricultural improvement are Pseudomonas, Paenibacillus, Rhizobium, Azospirillum, Bacillus, and Azotobacter, they can enhance plant growth and yield via amino acids production, nitrogen fixation, and plant hormones production [25]. The positive effects of PGPB may be due to its role in the production of phytohormones, siderophores, phosphate solubilization, and antibiotics [30]. PGPB acts as biofertilizers by increasing the accessibility of water and nutrients [31] under stressful conditions [27]. Inoculation of PGPB led to an increase in the root yield of sugar beet plants [32] and the growth characteristics as well as the yield of sorghum [33]. Abdelaal [32] reported that the inoculation with plant growth-promoting bacteria led to an increase in the growth characteristics of sugar beet consequently improving the anatomical structure such as the thickness of lamina and mesophyll tissue. PGPB may take up the tryptophan exuded by roots and convert it to IAA, which may be taken up by the plant, consequently, facilitate plant growth. Application of Azospirillum brasilense and Pseudomonas fluorescens led to increased shoot biomass of Urochloa spp. [34]. The active role of A. brasilense was also recorded on shoot dry mass production of rangeland plants, dry biomass was increased in both years [35]. Several studies were carried out regarding the effect of PGPB, but the information about this effect on drought, heat, and salinity is still insufficient, therefore, this review aimed to study the pivotal role of PGPB as a promising strategy to alleviate the negative impacts of abiotic stresses in several crops and increase the yield production.
2 Alleviating Drought Stress Effects on Growth and Yield Characters Using PGPB
Drought has become a major problem threatening agricultural production and sustainability, the harmful effects on the growth and yield of drought or water deficit stress were recorded in various plants. Oxidative stress components such as superoxide (O2•−), peroxyl radical (ROO•), singlet oxygen (1O,2), and hydrogen peroxide (H2O2) were produced in mitochondria, chloroplasts, and peroxisomes as a result to stress conditions such as drought. Furthermore, plants have another defense system to deal with oxidative stress, this defense system is antioxidant components which include enzymatic antioxidants and non-enzymatic antioxidants. The main enzymatic antioxidants are glutathione reductase, peroxidase, catalase, superoxide dismutase, ascorbate peroxidase, and dehydroascorbate reductase [36,37]. However, non-enzymatic antioxidants include α-Tocopherols (Vitamin E), ascorbic acid, proline, carotenoids, and glutathione [38,39]. Additionally, under stress conditions, plants have important adaptive mechanisms like reducing the growth of the shoot system, improving solute content, and osmotic adjustment also, stomatal closing is a very significant response to reduce water loss under drought stress [40]. There are some signaling pathways regulated by phytohormones such as JA, auxins, ABA, and cytokinin that control in closure mechanism’s response [41]. Another adaptive mechanism depends on the drought periods; under a short drought period, plants regulate water loss to avoid significant damage to their water-transport system. Nevertheless, under a long drought period, the evaporation was significantly increased, however, soil water decreased, resulting in a significant reduction in soil water content, consequently, decreasing cell differentiation and seedling growth [21].
Under drought conditions, plant growth-promoting bacteria can be used to improve plant growth by different mechanisms, these mechanisms include phosphorus solubilization, nitrogen uptake, and availability of essential nutrients as well as regulation of phytohormones that, maintain root structure to increase water and nutrient uptake [42,43]. Many studies showed the promotional effects of PGPB via improving water uptake and nutrient availability in soil, resulting in improving soil characters, consequently, increasing crop yield (Table 1). Application of PGPB led to improved morpho-physiological characters and yield production of various plants, through nitrogen fixation, and phosphate solubilization such as Azoarcus sp. [44], Serratia spp. [45] and Rhizobium spp. [46]. Additionally, the phosphorus availability was increased in the soil after treatment with phosphorus-solubilizing bacteria such as Rhizobium spp, Bacillus spp., and Pseudomonas spp, however, some genera of PGPB like Bacillus mucilaginosus, Ferrooxidans spp, Paenibacillus spp. and Pseudomonas sp. can solubilize potassium for plant uptake. Under drought conditions, the application of some genera of rhizobacteria led to an increase the growth characteristics and grain yield of wheat plants, this positive effect of rhizobacteria may be due to its role in increasing the production of phytohormones [47]. Moreover, García et al. [47] found that the application of Azospirillum led to an increase in the tolerance of maize plants to drought stress and enhanced its growth. In the experiment of Shirmohammadi et al. [48], they reported that the application of Pseudomonas (phosphate-solubilizing bacteria) led to increased phosphorus uptake and improved the yield of wheat plants. Previous studies showed that phosphate and phosphorus-solubilizing bacteria such as Bacillus edaphicus, Bacillus mucilaginosus, Acidithiobacillus sp, Ferrooxidans sp, Paenibacillus spp, and Pseudomonas sp. play a significant role as biofertilizer especially, under stress conditions to improve the growth and yield in the stressed plants via increasing the accessibility of free phosphorus, phosphate, increasing the efficiency of nitrogen fixation and improve the availability of essential nutrients for plant absorption [49,50].
The positive role of PGPB in enhancing plant growth under drought conditions was recorded with Azospirillum brasilense and Herbaspirillum seropedicae in maize plants [51] and Bacillus in pea plants [26]. This positive role could be due to the improvement of water availability and antioxidant components as well as accumulation of ABA and osmoprotectants which, improve the plant growth under drought conditions. Inoculation with Azospirillum led to improved growth of wheat roots and an increase in the formation of lateral roots due to indole acetic acid production under drought conditions [52,53]. Similarly, the application of Pseudomonas caused an increase in fresh weight and stem height as well as chlorophyll content and salicylic acid under drought conditions [54]. Inoculation with Azospirillum brasilense and Bacillus thuringiensis led to increased nutrient concentrations, enhanced metabolic activity, relative water contents, and improved membrane stability, consequently increasing the tolerance of wheat plants and Platycladus orientalis plants to drought stress conditions [55]. Meenakshi et al. [56] reported that the application of Bacillus subtilis, Bacillus thuringiensis, and Bacillus megaterium led to a significant increase in metabolic activities and chlorophyll concentration in the drought-stressed Cicer arietinum. The beneficial effects of PGPB under stress conditions are mediated by the formation of proline and osmolytes as well as improving the activity of the antioxidant system.
3 Mitigating the Effects of Salinity Stress on Growth and Yield Characters Using PGPB
Salinity is a main abiotic factor that detrimentally affects the growth and yield of many plants like strawberry [17], cucumber [19], faba bean [26], pea plants [28], and mung bean [69]. The negative effects of salt stress were observed on vegetative and reproductive stages particularly, physiological characteristics such as relative water content, chlorophyll content, the activity of antioxidant enzymes, and yield of several plants [16,34]. The reduction in chlorophyll content under salt stress might be due to the photooxidation process for chlorophyll [69,70]. Also, salinity stress is usually accompanied by oxidative stress due to the excessive production of ROS such as hydrogen peroxide and superoxide, which, cause lipid peroxidation in plant cells such as pepper plants [27] and pea plants [28]. The oxidative stress markers such as ROS, MDA, and EL% are the main indicators in the stressed plants and were significantly increased due to the oxidative damage to mitochondria and chloroplasts as well as other organelles under salt stress [71]. Under salinity conditions the plants can produce antioxidant components to scavenge the excessive formation of ROS, these components include enzymatic and nonenzymatic antioxidants. The production of enzymatic antioxidants for example SOD, CAT, and POX were recorded in stressed plants under salt stress to scavenge the ROS [16,34]. Also, nonenzymatic antioxidants like proline, carotenoids, and total phenolic compounds were observed under salinity stress. These antioxidants play a key role as osmo-regulators to protect the plant cells against oxidative damage. The adverse effects of salinity may be due to the inhibition of carbon fixation and the accumulation of hydrogen peroxide and superoxide, consequently reducing chlorophyll concentration [72].
Plant growth-promoting bacteria (PGPB) can improve physiological processes such as water uptake, nutrient absorption, and photosynthesis processes that enhance plant growth and development [73]. Application of plant growth-promoting bacteria was used to improve plant growth and increase yield production under salt stress (Table 2). Inoculation with PGPB led to an increase in relative water content and improved the structure of the root system and growth characters under salinity conditions, this increased because of the phytohormones production like IAA which increases the length and weight of roots [74]. The beneficial impact of PGPB might be attributed to the production of exopolysaccharides (EPSs) and an increase in the concentration of ABA, which decreases the negative effects on stomatal conductance and photosynthesis [75]. Azotobacter vinelandii, Enterobacter cloacae, and Rhizobium sp. were recorded to produce exopolysaccharides and enhance soil structure and fertility, improve water potential, and increase the plant tolerance to abiotic stress factors such as drought, and salinity to improve crop production [76]. Moreover, PGPB can produce important organic compounds such as ACC-deaminase and siderophores which enhance Fe concentrations and increase its availability in the soil. The activity ACC deaminase was reported for Enterobacter might alleviate salinity stress by reducing ethylene concentration [77]. The application of Bradyrhizobium japonicum + Enterobacter Delta PSK significantly improved the growth characteristics of soybean, while malondialdehyde, electrolyte leakage, and hydrogen peroxide were significantly decreased under salt stress conditions. Also, chlorophyll concentrations were increased with the application of Bradyrhizobium japonicum + Enterobacter Delta PSK and Pseudomonas 42P4 under salinity stress, this effect may be due to alleviatingalleviating the oxidative damages of salinity in soybean [78] and in tomato plants [79]. Ali et al. [80] reported that inoculation with Enterobacter cloacae PM23 led to improved growth characteristics and increase relative water content, proteins, and flavonoid content in maize plants under salinity conditions. Under salt stress, Mahmood et al. [81] found that Enterobacter cloacae and Bacillus drentensis treatment caused an improvement in the growth of mung beans by increasing the water uptake and nutrient accessibility in the stressed plants. Mukherjee et al. [82] studied the role of Halomonas sp. in promoting the growth characteristics and productivity of rice plants growing under salt conditions via siderophores production. Also, Sen et al. [83] found that the application of Pseudomonas species under salinity conditions increased root colonization of rice plants by secreting exopolysaccharides. Secretion of phytohormones, increased nutrient uptake, and nitrogen fixation are some of the various mechanisms that plant growth-promoting microbes use to promote plant growth and reduce the adverse effects of salt stress [83]. Several studies indicated that PGPB was effective in alleviating the harmful effects of stress factors improving the growth characteristics of many plant species that belong to cereals legumes, and vegetables, and enhancing stress tolerance [84]. Application with PGPB led to an increase in nutrient uptake such as N, K, and P due to the solubilizing power of P-solubilizing PGPB and K-solubilizing PGPB [85,86]. Under salt stress, Streptomyces sp. and Achromobacter sp. displayed the highest activity of ACC deaminase, however, Bacillus sp. gave the highest activity of siderophore compounds. These isolates also produced antioxidant enzyme activities such as superoxide dismutase, glutathione oxidase, and catalase in rice plants [87]. Also, Klebsiella variicola SURYA6 produced many compounds under salt stress conditions such as organic acid, high phosphorous solubilization index and siderophore, indole acetic acid, 1 aminocyclopropane-1-carboxylate deaminase, and exopolysaccharides as well higher activities of antioxidant enzymes like catalase, superoxide dismutase and glutathione oxidase [88]. Moreover, Bisht et al. [89] and AlKahtani et al. [90] reported that the application of plant growth-promoting bacteria markedly improved photosynthetic rate, stomatal conductance, carotenoids and chlorophylls concentration in Trigonella foenumgraecum and lettuce under salinity conditions.
Salinity stress is associated with oxidative damage to cell structures in the plant cells. Under salt stress conditions, the presence of PGPB can activate the antioxidant defense system to scavenge the reactive oxygen species that are produced under salt stress. Antioxidant enzymes such as CAT, SOD, and GSH were recorded to protect plants from oxidative stress. In this regard Klebsiella sp. IG 3 produced IAA, antioxidant enzymes, and proline, under salinity conditions, and increased growth characters in A. sativa [91]. Previous studies on wheat plants showed that the application of PGPB such as Pseudomonas sp. and Serratia sp. led to improved growth characteristics in the salt-stressed wheat plants (Table 2) via increasing ACC-deaminase production [92]. Also, Abdelaal [32] reported that the anatomical characteristics of sugar beet stems and leaves were improved with biofertilizer treatment which are clean and inexpensive sources of fertilizers, and act as alternative sources for chemical fertilizers.
4 Justifying the Effects of Heat Stress Using PGPB
Heat stress is one of the most common abiotic stresses that negatively affects morphophysiological and biochemical functions in many plants, like stem height, branch number, membrane permeability, relative water content, and photosynthetic rate. Photosynthesis is an important physiological process and is highly sensitive to temperature changes, so, the photosynthetic rate in leaves was decreased under high temperatures. Chlorophyll fluorescence parameters were decreased under heat stress conditions, this negative effect of heat stress may be due to heat-induced inhibition of light energy absorption and energy distribution. Ahammed et al. [99] reported that heat stress negatively affected the electron transport efficiency in tomato plants, also, enzyme activity, cell division, reactive oxygen species, and other oxidative stress markers have been linked to heat-stressed plants [100]. Exposure to heat stress negatively affects growth characteristics, metabolic processes, chlorophyll concentrations, and the efficiency of photosynthetic apparatus [101].
The effect of plant growth-promoting bacteria was studied to protect the plants from heat stress, in this concern, Mukhtar et al. [102] reported that the application of Bacillus safensis (SCAL1) caused an increase in growth characteristics of tomato plants under various heat stress regimes via increasing ACC deaminase and exopolysaccharide. Shaffique et al. [103] stated the increase of various heat stress regulators such as IAA, gibberellin, cytokinin, and organic acids in stressed plants under heat stress conditions. Previous studies have proved that PGPB plays a significant role in the justification of heat stress. Inoculated wheat plants with Bacillus amyloliquefaciens UCMB5113 reduced heat stress parameters such as ascorbate peroxidase, glutathione reductase, and heat-shock protein (HSP17) expression [104]. In another study, inoculated soybean plants with Bacillus cereus improved chlorophyll a, b concentrations, carotenoids, and proteins [104]. Application of Pseudomonas aeruginosa strain 2CpS1 led to ameliorating heat stress effects in wheat plants [105]. Another report stated that Rhizobia increases thermotolerance by activating the heat shock proteins [106]. Application of Bacillus licheniformis strain BE-L60 can ameliorate the harmful effects of heat stress via secreting IAA and dissolving phosphorus, increasing proline content, and soluble protein, and improving the activity of antioxidant enzymes such as superoxide dismutase, peroxidase, and catalase in heat-stressed spinach plants [107]. Duarte et al. [108] studied the role of plant growth-promoting bacteria on Salicornia ramosissima plants under heat stress, they found that physiological stress levels were decreased such as excessive fluidity and several antioxidant enzymes as well as lipid peroxidation products, however, membrane stability was improved in the stressed plants.
5 Gene Expression and Signaling Mechanisms under Salinity Stress and PGPB Application
Plants have genetic traits and developed mechanisms to cope with abiotic stress factors such as salinity stress. Plant growth-promoting bacteria become one of the most important methods to improve the salt tolerance mechanism in stressed plants [108]. The signaling mechanisms start with surface receptors that perceive the signals, then, secondary messengers are produced such as ROS that start the plant signal transduction. During the stress period, gene expressions are managed by calcium ion regulation brought about by the secondary messengers that act upon calcium-dependent protein kinase and protein phosphatases [109,110]. Cotton plants treated with Bacillus pumilus markedly expressed ascorbate metabolism and glyoxylate pathways as well as dicarboxylate metabolism pathways. However, cotton plants treated with Bacillus subtilis caused expressed pentose and glucuronate pathway genes [111].
Under environmental stresses, plants have two important pathways (MAPK and CDPK) to overcome the adverse effects of various stresses. Under stress conditions, MAPK is activated by many signals and the response to environmental stress occurs quickly by the presence of proteins sensing calcium (CDPK and calmodulins) and via modifying cytoplasmic calcium ions. CDPK plays a significant role in ROS production and ABA in plants under abiotic stresses. Additionally, plant-promoting growth-promoting bacteria can produce various phytohormones such as gibberellin, cytokinin, IAA, ABA, and ethylene which act as important signals in the plant tolerance to cope with stress factors [112]. Also, the application of PGPB led to an increase in the production of organic compounds that help in plant-bacteria communication [113]. Under salt stress, PGPB plays an important role in salinity tolerance by regulating ion homeostasis and uptake. The results of Ilangumaran et al. [114] proved that numerous genes such as MAPK, DREB, and CIPK12 of PGPB are involved in modulating phytohormones which play a significant role in the plant defense and adaptation against salinity stress (Table 3).
6 Conclusions and Future Perspectives
This review displays the significant role of plant growth-promoting bacteria in improving growth and yield under various environmental conditions such as salinity, drought, and heat. Generally, the application of plant growth-promoting bacteria in alleviating the adverse effects of abiotic stress factors such as salinity, drought, and heat in the agricultural system is a promising, safe, and effective strategy, they can improve growth characteristics of plants under abnormal conditions such as drought, salinity, and heat via production of growth hormone, fixation of atmospheric nitrogen, solubilizing of inorganic phosphate and exopolysaccharides production. Furthermore, PGPB helps in the accumulation of osmoprotectants, activating the antioxidant enzymes, organic compounds production, and the production of the ACC deaminase enzyme as well as scavenging the reactive oxygen species. It can be concluded that PGPB is an important alternative method to control abiotic stress in plants, improve plant growth characteristics, and increase yield production under stress conditions. Additional studies are still required to find the best strain of plant growth-promoting bacteria for plant species to improve the growth and yield under several stress factors as well as improve agricultural sustainability.
Acknowledgement: This work was supported by the Deanship of Scientific Research, Vice Presidency for Graduate Studies and Scientific Research, King Faisal University, Saudi Arabia (Grant No. 3783).
Funding Statement: This work was supported by the Deanship of Scientific Research, Vice Presidency for Graduate Studies and Scientific Research, King Faisal University, Saudi Arabia (Grant No. 3783).
Author Contributions: Conceptualization, Literature Review, Research Design, Editing and reviewing, supervision, and Funding: A.H.A; Conceptualization, Literature Review, Research Design, Analysis, and Funding: O.A.A; Conceptualization, Literature Review, Research Design, Analysis, and References and Citations: H.E.H.M; H.S.G; Conceptualization, Literature Review, Data Collection, writing—original draft preparation, and References and Citations: M.S.A; Conceptualization, Literature Review, Data Collection, writing—original draft preparation, and editing and reviewing: N.A.A; Conceptualization, Literature Review, Data Collection, writing—original draft preparation: W.B.A; Conceptualization, Literature Review, Research Design, Data Collection, Analysis, writing—original draft preparation, and supervision: K.A.
Availability of Data and Materials: Data sharing is not applicable to this article as no new data were created or analyzed in this study.
Ethics Approval: Not applicable.
Conflicts of Interest: Data sharing is not applicable to this article as no new data were created or analyzed in this study.
References
1. Mahmoud, G. A. E. (2021). Biotic stress to legumes: Fungal diseases as major biotic stress factor. Sustainable Agriculture Reviews 51: Legume Agriculture and Biotechnology, 2, 181–212. [Google Scholar]
2. Hafez, Y., Ali, G., Shahin, A., Badr, M., Esmaeil, R. et al. (2022). Screening of resistance sources to stem rust (Ug99) in international wheat genotypes (CIMMYT)I Egypt. Fresenius Environmental Bulletin, 31(6), 5940–5948. [Google Scholar]
3. Hafez, Y., Mazrou, Y., Shahin, A., Nehiar, F., Eid, M. et al. (2022). Yield losses in wheat genotypes caused by stripe rust (Puccinia striifarmis f. sp. tritici) in North Delta, Egypt. Notulae Botanicae Horti Agrobotanici Cluj-Napoca, 50(2), 12622. https://doi.org/10.15835/nbha50212622 [Google Scholar] [CrossRef]
4. ALKahtani, M. D. F., Fouda, A., Attia, K., Al-Otaibi, F., Eid, A. M. et al. (2020). Isolation and characterization of plant growth promoting endophytic bacteria from desert plants and their application as bioinoculants for sustainable agriculture. Agronomy, 10(9), 1325. https://doi.org/10.3390/agronomy10091325 [Google Scholar] [CrossRef]
5. Hafez, Y., El-Kazzaz, K., El-Kady, E., Riad, N. M., Attia, A. et al. (2022). Molecular identification of new races of agrobacterium spp using genomic sequencing-based on 16s primers: Screening of biological agents and nano-silver which mitigated the microbe in vitro. Fresenius Environmental Bulletin, 31(10), 10447–10458. [Google Scholar]
6. Roossinckm, M. J. (2020). Persistent plant viruses: Molecular hitchhikers or epigenetic elements? In: Witzany, G. (Ed.Viruses: Essential agents of life, pp. 177–186. Dordrecht, The Netherlands: Springer. [Google Scholar]
7. Essawy, M. M., Keratum, A. Y., Abdallah, F., Mohamed, H. M., Mazrou, Y. et al. (2020). Susceptibility of some faba bean varieties to infestation with the main insect pests associated with physiological, biochemical and yield characters. Fresenius Environmental Bulletin, 29(7A), 6147–4758. [Google Scholar]
8. El-Tokhy, A. I. A., Ali, M., Hafez, Y., Abdelaal, K. H (2020). Impacts of different insecticides on Tuta absoluta (Meyrick) larvae with their effects on physiological characteristics and fruits yield of stressed tomato plants. Journal of Plant Protection and Pathology, Mansoura University, 11(6), 269–274. https://doi.org/10.21608/jppp.2020.111557 [Google Scholar] [CrossRef]
9. Abu Arab, H. R., Keratum, A., Abouelatta, A. M., El-Tawelah, N. M., Hafez, Y. et al. (2022). Fumigant and mixing with medium effect of Citrus reticulata and Tagetes minuta essential oil against adults of Sitophilus oryzae (L.) and Tribolium castaneum (Herbst). Fresenius Environmental Bulletin, 31(11), 10758–10766. [Google Scholar]
10. Abu Arab, H. R., Keratum, A. Y., Abouelatta, A. M., El-Zun, H. M., Hafez, Y. et al. (2022). Fumigant and contact toxicity of some essential components against three stored product insects. Fresenius Environmental Bulletin, 31(10), 10136–10143. [Google Scholar]
11. EL-Sayed, A. A., Mazrou, Y., Khaffagy, A. E., Shaheen, F. E. M., Hafez, Y. et al. (2021). Impacts of herbicides and some growth characters of maize and associated weeds. Fresenius Environmental Bulletin, 30(7A), 9380–9388. [Google Scholar]
12. Khaffagy, A. E., Mazrou, Y. S. A., Morsy, A. R., El-Mansoury, M. A. M., El-Tokhy, A. I. et al. (2022). Impact of irrigation levels and weed control treatments on annual weeds, physiological traits and productivity of soybean under clay soil conditions. Agronomy, 12(5), 1037. https://doi.org/10.3390/agronomy12051037 [Google Scholar] [CrossRef]
13. Mohamed, A., Mazrou, Y., Al-Shammari, W., El-Shamy, M., EL-Kholy, K. et al. (2022). Impact of intercropping faba bean with onion, garlic and fenugreek on crop productivity and control of Orobanche crenata. Fresenius Environmental Bulletin, 31(8), 7596–7603. [Google Scholar]
14. Sabagh, E. L., Hossain, A., Barutcular, A., Islam, C., Awan, M. S. et al. (2019). Wheat (Triticum aestivum L.) production under drought and heat stress-adverse effects mechanisms and mitigation: A review. Applied Ecology and Environmental Research, 17(4), 8307–8332. https://doi.org/10.15666/aeer/1704_83078332 [Google Scholar] [CrossRef]
15. Rashwan, E., Alsohim, A. S., El-Gammaal, A., Hafez, Y., Abdelaal, KH. (2020). Foliar application of nano zink-oxide can alleviate the harmful effects of water deficit on some flax cultivars under drought conditions. Fresenius Environmental Bulletin, 29(10), 8889–8904. [Google Scholar]
16. Abdelaal, K. H, AlKahtani, M. D. F., Attia, K., Hafez, Y., Lóránt, K. et al. (2021). The role of plant growth-promoting bacteria in alleviating the adverse effects of drought on plants. Biology, 10(6), 520. https://doi.org/10.3390/biology10060520 [Google Scholar] [PubMed] [CrossRef]
17. Elkelish, A., Qari, S. H., Mazrou, Y. M., Abdelaal, KH., Hafez, Y. et al. (2020). Exogenous ascorbic acid induced chilling tolerance in tomato plants through modulating metabolism, osmolytes, antioxidants, and transcriptional regulation of catalase and heat shock proteins. Plants, 9(4), 431. https://doi.org/10.3390/plants9040431 [Google Scholar] [PubMed] [CrossRef]
18. Alnusairi, G. S. H., Mazrou, Y. S. A., Qari, S. H., Elkelish, A. A., Soliman, M. H. et al. (2021). Exogenous Nitric oxide reinforces photosynthetic efficiency, osmolyte, mineral uptake, antioxidant, expression of stress-responsive genes and ameliorates the effects of salinity stress in wheat. Plants, 10(8), 1693. https://doi.org/10.3390/plants10081693 [Google Scholar] [PubMed] [CrossRef]
19. Helaly, M. N., Mohammed, Z., El-Shaeery, N. I., Abdelaal, K. H, Nofal, I. E. (2017). Cucumber grafting onto pumpkin can represent an interesting tool to minimize salinity stress. Physiological and anatomical studies. Middle East Journal of Agriculture Research, 6(4), 953–975. [Google Scholar]
20. Abdelaal, K. H, Attia, K., Alamery, S. F., El-Afry, M. M., Ghazy, A. I. et al. (2020). Exogenous application of proline and salicylic acid can mitigate the injurious impacts of drought stress on barley plants associated with physiological and histological characters. Sustainability, 12(5), 1736. https://doi.org/10.3390/su12051736 [Google Scholar] [CrossRef]
21. Arafa, S. A., Attia, K., Niedbała, G., Piekutowska, M., Alamery, S. et al. (2021). Seed priming boost adaptation in pea plants under drought stress. Plants, 10(10), 2201. https://doi.org/10.3390/plants10102201 [Google Scholar] [PubMed] [CrossRef]
22. Hafez, Y., Attia, K., Alamery, S., Ghazy, A., Al-Dosse, A. et al. (2020). Beneficial effects of biochar and chitosan on antioxidative capacity, osmolytes accumulation, and anatomical characters of water-stressed barley plants. Agronomy, 10(5), 630. https://doi.org/10.3390/agronomy10050630 [Google Scholar] [CrossRef]
23. AlKahtani, M. D. F., Hafez, Y. M., Attia, K., Rashwan, E., Husnain, L. A. et al. (2021). Evaluation of silicon and proline application on the oxidative machinery in drought-stressed sugar beet. Antioxidants, 10(3), 398. https://doi.org/10.3390/antiox10030398 [Google Scholar] [PubMed] [CrossRef]
24. Soliman, M. H., Alayafi, A. A. M., El Kelish, A. A., Abu-Elsaoud, A. M. (2018). Acetylsalicylic acid enhance tolerance of Phaseolus vulgaris L. to chilling stress, improving photosynthesis, antioxidants and expression of cold stress responsive genes. Botanical Studies, 59(1), 6. https://doi.org/10.1186/s40529-018-0222-1 [Google Scholar] [PubMed] [CrossRef]
25. Farooq, M., Hussain, M., Nawaz, A., Lee, D. J., Alghamdi, S. S. et al. (2017). Seed priming improves chilling tolerance in chickpea by modulating germination metabolism, trehalose accumulation and carbon assimilation. Plant Physiology and Biochemistry, 111, 274–283. https://doi.org/10.1016/j.plaphy.2016.12.012 [Google Scholar] [PubMed] [CrossRef]
26. El-Flaah, R. F., El-Said, R. A. R., Nassar, M. A., Hassan, M., Abdelaal, K. H (2021). Effect of Rhizobium, nano silica and ascorbic acid on morpho-physiological characters and gene expression of POX and PPO in faba bean (Vicia faba L.) under salinity stress conditions. Fresenius Environmental Bulletin, 30(6), 5751–5764. [Google Scholar]
27. ALKahtani, M. D. F., Attia, K. A., Hafez, Y. M., Khan, N., Eid, A. M. et al. (2020). Chlorophyll fluorescence parameters and antioxidant defense system can display salt tolerance of salt acclimated sweet pepper plants treated with chitosan and plant growth promoting rhizobacteria. Agronomy, 10(8), 1180. https://doi.org/10.3390/agronomy10081180 [Google Scholar] [CrossRef]
28. Al-Shammari, W. B., Altamimi, H. R., Abdelaal, K. (2023). Improvement in physiobiochemical and yield characteristics of pea plants with nano silica and melatonin under salinity stress conditions. Horticulturae, 9(6), 711. https://doi.org/10.3390/horticulturae9060711 [Google Scholar] [CrossRef]
29. Yadav, A. N., Verma, P., Kour, D., Rana, K. L., Kumar, V. et al. (2017). Plant microbiomes and its beneficial multifunctional plant growth promoting attributes. International Journal of Environmental Sciences & Natural Resources, 3(1), 1–8. https://doi.org/10.19080/IJESNR.2017.03.555601 [Google Scholar] [CrossRef]
30. Kour, D., Rana, K. L., Yadav, A. N., Yadav, N., Kumar, M. et al. (2020). Microbial biofertilizers: Bioresources and eco-friendly technologies for agricultural and environmental sustainability. Biocatalysis and Agricultural Biotechnology, 23, 101487. https://doi.org/10.1016/j.bcab.2019.101487 [Google Scholar] [CrossRef]
31. Çakmakçi, R., Dönmez, F., Aydın, A., Şahin, F. (2006). Growth promotion of plants by plant growth promoting rhizobacteria under greenhouse and two different field soil conditions. Soil Biology and Biochemistry, 38(6), 1482–1487. https://doi.org/10.1016/j.soilbio.2005.09.019 [Google Scholar] [CrossRef]
32. Abdelaal, K. H (2015a). Pivotal role of bio and mineral fertilizer combinations on morphological anatomical and yield characters of sugar beet plant (Beta vulgaris L.). Middle East Journal of Agriculture Research, 4(4), 717–734. [Google Scholar]
33. Abdelaal, K. H, Badawy, S. H. A., Abdel Aziz, R. M., Neana, S. H (2015). Integrated effect of mineral nitrogen levels and biofertilizer on morphophysiological characters of three sweet sorghum varieties (Sorghum bicolor L. Moench). Journal Plant Production Mansoura University, 6(2), 189–203. [Google Scholar]
34. Hungria, M., Rondina, A. B. L., Nunes, A. L. P., Araujo, R. S., Nogueira, M. A. (2021). Seed and leaf-spray inoculation of PGPB in brachiarias (Urochloa spp.) as an economic and environmental opportunity to improve plant growth forage yield and nutrient status. Plant and Soil, 46(3), 171–186. https://doi.org/10.1007/s11104-021-04908-x [Google Scholar] [CrossRef]
35. Heinrichs, R., Meirelles, G. C., de Melo Santos, L. F., da Silva Lira, M. V., de Marcos Lapaz, A. et al. (2020). Azospirillum inoculation of’ Marandu’palisade grass seeds: Effects on forage production and nutritional status. Semina: Cincias Agrárias, 41(2), 465–478. https://doi.org/10.5433/1679-0359.2020v41n2p465 [Google Scholar] [CrossRef]
36. Pappalardo, H., Genovese, C., Puglia, G. D., Leonardi, C., Toscano, V. et al. (2020). Principal mechanism of tolerance to abiotic stresses in Cynara cardunculus L. Acta Horticulturae, 1284, 109–116. https://doi.org/10.17660/ActaHortic.2020.1284.14 [Google Scholar] [CrossRef]
37. Michel, H. (2013). Carotenoid oxidation products as stress signals in plant. The Plant Journal, 79(4), 597–606. https://doi.org/10.1111/tpj.12386 [Google Scholar] [PubMed] [CrossRef]
38. Michaeli, S., Fromm, H. (2015). Closing the loop on the GABA shunt in plants: Are GABA metabolism and signaling entwined? Frontiers in Plant Sciences, 6, 419. https://doi.org/10.3389/fpls.2015.00419 [Google Scholar] [PubMed] [CrossRef]
39. Koyro, H. W., Ahmad, P., Geissler, N. (2012). Abiotic stress responses in plants: An overview. In: Ahmad, P., Prasad, M. N. V. (Eds.Environmental adaptations and stress tolerance of plants in the era of climate change, pp. 1–28. New York, NY, USA: Springer. [Google Scholar]
40. Nemhauser, J. L., Hong, F., Chory, J. (2006). Different plant hormones regulate similar processes through largely nonoverlapping transcriptional responses. Cell, 126(3), 467–475. https://doi.org/10.1016/j.cell.2006.05.050 [Google Scholar] [PubMed] [CrossRef]
41. Beneduzi, A., Ambrosini, A., Passaglia, L. M. (2012). Plant growth-promoting rhizobacteria (PGPBTheir potential as antagonists and biocontrol agents. Genetics and Molecular Biology, 35(4), 1044–1051. https://doi.org/10.1590/S1415-47572012000600020 [Google Scholar] [PubMed] [CrossRef]
42. Venkateshwaran, M., Volkening, J. D., Sussman, M. R., Ané, J. M. (2013). Symbiosis and the social network of higher plants. Current Opinion in Plant Biology, 16(1), 118–127. https://doi.org/10.1016/j.pbi.2012.11.007 [Google Scholar] [PubMed] [CrossRef]
43. Damam, M., Kaloori, K., Gaddam, B., Kausar, R. (2016). Plant growth promoting substances (phytohormones) produced by rhizobacterial strains isolated from the rhizosphere of medicinal plants. International Journal of Pharmaceutical Sciences Review and Research, 37, 130–136. [Google Scholar]
44. Egener, T., Hurek, T., Reinhold-Hurek, B. (1999). Endophytic expression of nif genes of Azoarcus sp. strain BH72 in rice roots. Molecular Plant Microbe Interaction, 12(9), 813–819. https://doi.org/10.1094/MPMI.1999.12.9.813 [Google Scholar] [CrossRef]
45. Gyaneshwar, P., James, E. K., Mathan, N., Reddy, P. M., Reinhold-Hurek, B. et al. (2001). Endophytic colonization of rice by a diazotrophic strain of Serratia marcescens. Journal of Bacteriology, 183(8), 2634–2645. https://doi.org/10.1128/JB.183.8.2634-2645.2001 [Google Scholar] [PubMed] [CrossRef]
46. Raheem, A., Shaposhnikov, A., Belimov, A. A., Dodd, I. C., Ali, B. (2018). Auxin production by rhizobacteria was associated with improved yield of wheat (Triticum aestivum L.) under drought stress. Archives of Agronomy and Soil Science, 64(4), 574–587. https://doi.org/10.1080/03650340.2017.1362105 [Google Scholar] [CrossRef]
47. García, J. E., Maroniche, G., Creus, C., Suárez-Rodríguez, R., RamirezTrujillo, J. A. et al. (2017). In vitro PGPB properties and osmotic tolerance of different Azospirillum native strains and their effects on growth of maize under drought stress. Microbiological Research, 202(1), 21–29. https://doi.org/10.1016/j.micres.2017.04.007 [Google Scholar] [PubMed] [CrossRef]
48. Shirmohammadi, E., Alikhani, H. A., Pourbabaei, A. A., Etesami, H. (2020). Improved phosphorus (P) uptake and yield of rainfed wheat fed with P fertilizer by drought tolerant phosphate-solubilizing fluorescent pseudomonads strains: A field study in drylands. Journal of Soil Science and Plant Nutrition, 20(4), 2195–2211. https://doi.org/10.1007/s42729-020-00287-x [Google Scholar] [CrossRef]
49. Etesami, H., Beattie, G. A. (2017). Plant-microbe interactions in adaptation of agricultural crops to abiotic stress conditions. In: Kumar, V., Kumar, M., Sharma, S., Prasad, R. (Eds.Probiotics and plant health, pp. 163–200. Singapore: Springer. https://doi.org/10.1007/978-981-10-3473-2_7 [Google Scholar] [CrossRef]
50. Rajawat, M. V. S., Singh, R., Singh, D., Yadav, A. N., Singh, S. et al. (2020). Spatial distribution and identification of bacteria in stressed environments capable to weather potassium aluminosilicate mineral. Brazilian Journal of Microbiology, 51(2), 751–764. https://doi.org/10.1007/s42770-019-00210-2 [Google Scholar] [PubMed] [CrossRef]
51. Tiwari, P., Bajpai, M., Singh, L. K., Mishra, S., Yadav, A. N. (2020). Phytohormones producing fungal communities: Metabolic engineering for abiotic stress tolerance in crops. In: Yadav, A. N., Mishra, S., Kour, D., Yadav, N., Kumar, A. (Eds.Agriculturally important fungi for sustainable agriculture perspective for diversity and crop productivity, vol. 1, pp. 56–86. Springer, Cham. [Google Scholar]
52. Rolli, E., Marasco, R., Vigani, G. (2015). Improved plant resistance to drought is promoted by the root-associated microbiome as a water stress-dependent trait. Environmental Microbiology, 17(2), 316–331. https://doi.org/10.1111/1462-2920.12439 [Google Scholar] [PubMed] [CrossRef]
53. Sharma, A., Shahzad, B., Kumar, V. (2019). Phytohormones regulate accumulation of osmolytes under abiotic stress. Biomolecules, 9(7), 285. https://doi.org/10.3390/biom9070285 [Google Scholar] [PubMed] [CrossRef]
54. Furlan, F., Saatkamp, K., Volpiano, C. G., Franco, F., Santos, M. F. et al. (2017). Plant growth-promoting bacteria effect in withstanding drought in wheat cultivars. Scientia Agrarian, 18(2), 104–113. https://doi.org/10.5380/rsa.v18i2.51385 [Google Scholar] [CrossRef]
55. Barnawal, D., Bharti, N., Pand, S. S., Pandey, A., Chanotiya, S. C. et al. (2017). Plant growth promoting rhizobacteria enhance wheat salt and drought stress tolerance by altering endogenous phytohormone levels and TaCTR1/TaDREB2 expression. Physiologia Plantarum, 161(4), 502–514. https://doi.org/10.1111/ppl.12614 [Google Scholar] [PubMed] [CrossRef]
56. Meenakshi, K., Govindasamy, V., Ajit, V., Choudhary, D. K. (2019). Mitigation of drought stress in wheat crop by drought tolerant endophytic bacterial isolates. Vegetos, 32(4), 486–493. https://doi.org/10.1007/s42535-019-00060-1 [Google Scholar] [CrossRef]
57. Nemeskéri, E., Horváth, K. Z., Andryei, B., Ilahy, R., Takács, S. et al. (2022). Impact of plant growth-promoting rhizobacteria inoculation on the physiological response and productivity traits of field-grown tomatoes in hungary. Horticulturae, 8(7), 641. https://doi.org/10.3390/horticulturae8070641 [Google Scholar] [CrossRef]
58. Sandhya, V., Ali, S. Z., Grover, M., Reddy, G., Venkateswarlu, B. (2010). Effect of plant growth promoting Pseudomonas spp on compatible solutes antioxidant status and plant growth of maize under drought stress. Plant Growth Regulation, 62(1), 21–30. https://doi.org/10.1007/s10725-010-9479-4 [Google Scholar] [CrossRef]
59. Karimzadeh, J., Alikhani, H. A., Etesami, H., Pourbabaei, A. A. (2021). Improved phosphorus uptake by wheat plant (Triticum aestivum L.) with rhizosphere fluorescent Pseudomonads strains under water-deficit stress. Journal Plant Growth Regulation, 40(1), 162–178. https://doi.org/10.1007/s00344-020-10087-3 [Google Scholar] [CrossRef]
60. Mahmoudi, T. R., Yu, J. M., Liu, S., Pierson, L. S., Pierson, E. A. (2019). Drought-stress tolerance in wheat seedlings conferred by phenazine-producing rhizobacteria. Frontiers in Microbiology, 10, 1590. https://doi.org/10.3389/fmicb.2019.01590 [Google Scholar] [PubMed] [CrossRef]
61. Mahreen, N., Yasmin, S., Asif, M., Yahya, M., Ejaz, K. et al. (2023). Mitigation of water scarcity with sustained growth of rice by plant growth promoting bacteria. Frontiers in Plant Science, 14, 1081537. https://doi.org/10.3389/fpls.2023.1081537 [Google Scholar] [PubMed] [CrossRef]
62. Liu, F., Ma, H., Liu, B., Du, Z., Ma, B. et al. (2023). Effects of plant growth-promoting rhizobacteria on the physioecological characteristics and growth of walnut seedlings under drought stress. Agronomy, 13(2), 290. https://doi.org/10.3390/agronomy13020290 [Google Scholar] [CrossRef]
63. Ghosh, D., Gupta, A., Mohapatra, S. A. (2019). Comparative analysis of exopolysaccharide and phytohormone secretions by four drought-tolerant rhizobacterial strains and their impact on osmotic-stress mitigation in Arabidopsis thaliana. World Journal of Microbiology and Biotechnology, 35(6), 90. https://doi.org/10.1007/s11274-019-2659-0 [Google Scholar] [PubMed] [CrossRef]
64. Danish, S., Zafar-Ul-Hye, M., Hussain, S., Riaz, M., Qayyum, M. F. (2020). Mitigation of drought stress in maize through inoculation with drought tolerant ACC deaminase containing PGPB under axenic conditions. Pakistan Journal of Botany, 52(1). https://doi.org/10.30848/PJB2020-1(7) [Google Scholar] [CrossRef]
65. Igiehon, O. N., Babalola, O. (2021). Rhizobium and mycorrhizal fungal species improved soybean yield under drought stress conditions. Currant Microbiology, 78(4), 1615–1627. https://doi.org/10.1007/s00284-021-02432-w [Google Scholar] [PubMed] [CrossRef]
66. Admassie, M., Woldehawariat, Y., Alemu, T., Gonzalez, E., Jimenez, J. F. (2022). The role of plant growth-promoting bacteria in alleviating drought stress on pepper plants. Agricultural Water Management, 272, 107831. https://doi.org/10.1016/j.agwat.2022.107831 [Google Scholar] [CrossRef]
67. Petrillo, C., Vitale, E., Ambrosino, P., Arena, C., Isticato, R. (2022). Plant growth-promoting bacterial consortia as a strategy to alleviate drought stress in Spinacia oleracea. Microorganisms, 10(9), 1798. https://doi.org/10.3390/microorganisms10091798 [Google Scholar] [PubMed] [CrossRef]
68. Kumar, A., Behera, I., Langthasa, M., Prakash Naroju, S. (2023). Effect of plant growth-promoting rhizobacteria on alleviating salinity stress in plants: A review. Journal of Plant Nutrition, 46(10), 2525–2550. https://doi.org/10.1080/01904167.2022.2155548 [Google Scholar] [CrossRef]
69. Hasan, M. K., El Sabagh, A., Sikdar, M., Alam, M. D, Ratnasekera, D. et al. (2017). Comparative adaptable agronomic traits of blackgram and mung bean for saline lands. Plant Archives, 17(1), 589–593. [Google Scholar]
70. Sukweenadhi, J., Balusamy, S. R., Kim, Y. J., Lee, C. H., Kim, Y. J. et al. (2018). A growth-promoting bacteria Paenibacillus yonginensis DCY84T enhanced salt stress tolerance by activating defense-related systems in panax ginseng. Frontiers in Plant Science, 9, 813. https://doi.org/10.3389/fpls.2018.00813 [Google Scholar] [PubMed] [CrossRef]
71. El Nahhas, N., AlKahtani, M., Abdelaal, K. H, Al Husnain, L., AlGwaiz, H. et al. (2021). Biochar and jasmonic acid application attenuates antioxidative systems and improves growth, physiology, nutrient uptake and productivity of faba bean (Vicia faba L.) irrigated with saline water. Plant Physiology and Biochemistry, 166(98), 807–817. https://doi.org/10.1016/j.plaphy.2021.06.033 [Google Scholar] [PubMed] [CrossRef]
72. Ghanem, A. E. M. M., Mohamed, E., Kasem, A. M. M. A., El-Ghamery, A. A. (2021). Differential salt tolerance strategies in three halophytes from the same ecological habitat: Augmentation of antioxidant enzymes and compounds. Plants, 10(6), 1100. https://doi.org/10.3390/plants10061100 [Google Scholar] [PubMed] [CrossRef]
73. Kumar, K., Anil, A., Geetha, P., Kumar, T., Pandey, B. (2023). Effect of plant growth regulators and plant growth promoting rhizobacteria on physio-chemical properties of mung bean under drought stress. Biological Forum—An International Journal, 15(4), 753–761. [Google Scholar]
74. Naz, I., Bano, A., Ul-Hassan, T. (2009). Isolation of phytohormones producing plant growth promoting rhizobacteria from weeds growing in Khewra salt range, Pakistan and their implication in providing salt tolerance to Glycine max L. African Journal of Biotechnology, 8(21), 5762–5768. https://doi.org/10.5897/AJB09.1176 [Google Scholar] [CrossRef]
75. Shilev, S. (2020). Plant-growth-promoting bacteria mitigating soil salinity stress in plants. Applied Sciences, 10(20), 7326. https://doi.org/10.3390/app10207326 [Google Scholar] [CrossRef]
76. Pawar, S. T., Bhosale, A. A., Gawade, T. B., Nale, T. R. (2013). Isolation, screening and optimization of exopolysaccharide producing bacterium from saline soil. Journal of Microbiology and Biotechnology Research, 3, 24–31. [Google Scholar]
77. Singh, R. P., Pandey, D. M., Jha, P. N., Ma, Y. (2022). ACC deaminase producing rhizobacterium enterobacter cloacae ZNP-4 enhance abiotic stress tolerance in wheat plant. PLoS One, 17(5), e0267127. https://doi.org/10.1371/journal.pone.0267127 [Google Scholar] [PubMed] [CrossRef]
78. Agha, M. S., Haroun, S. A., Abbas, M. A., Sofy, M., Mowafy, A. M. (2023). Growth and metabolic response of glycine max to the plant growth-promoting enterobacter delta PSK and bradyrhizobium japonicum under salinity stress. Journal Plant Growth Regulation, 42, 5816–5830. https://doi.org/10.1007/s00344-023-10967-4 [Google Scholar] [CrossRef]
79. Pérez-Rodriguez, M. M., Pontin, M., Piccoli, P., Lobato Ureche, M. A., Gordillo, M. G. et al. (2022). Halotolerant native bacteria Enterobacter 64S1 and Pseudomonas 42P4 alleviate saline stress in tomato plants. Physiologia Plantarum, 174(4), e13742. https://doi.org/10.1111/ppl.13742 [Google Scholar] [PubMed] [CrossRef]
80. Ali, B., Wang, X., Saleem, M. H., Hafeez, A., Afridi, M. S. et al. (2022). PGPB-mediated salt tolerance in maize by modulating plant physiology, antioxidant defense, compatible solutes accumulation and bio-surfactant producing genes. Plants, 11(3), 345. https://doi.org/10.3390/plants11030345 [Google Scholar] [PubMed] [CrossRef]
81. Mahmood, S., Daur, I., Al-Solaimani, S. G., Ahmad, S., Madkour, M. H. et al. (2016). Plant growth promoting rhizobacteria and silicon synergistically enhance salinity tolerance of mung bean. Frontiers in Plant Science, 7(243), 876. https://doi.org/10.3389/fpls.2016.00876 [Google Scholar] [PubMed] [CrossRef]
82. Mukherjee, P., Mitra, A., Roy, M. (2019). Halomonas rhizobacteria of Avicennia marina of indian sundarbans promote rice growth under saline and heavy metal stresses through exopolysaccharide production. Frontiers in Microbiology, 10, 1207. https://doi.org/10.3389/fmicb.2019.01207 [Google Scholar] [PubMed] [CrossRef]
83. Sen, S., Chandrasekhar, C. N. (2014). Effect of PGPB on growth promotion of rice (Oryza sativa L.) under salt stress. Asian Journal Plant Sciences and Research, 4, 62–67. [Google Scholar]
84. Choudhary, M., Chandra, P., Dixit, B., Nehra, V., Choudhary, U. et al. (2022). Plant growth-promoting microbes: Role and prospective in amelioration of salt stress. Communications in Soil Science and Plant Analysis, 53(13), 1692–1711. https://doi.org/10.1080/00103624.2022.2063316 [Google Scholar] [CrossRef]
85. Glick, B. R. (2012). Plant growth-promoting bacteria: Mechanisms and applications. Scientifica, 2012, 963401. https://doi.org/10.6064/2012/963401 [Google Scholar] [PubMed] [CrossRef]
86. Zahir, Z. A., Ghani, U., Naveed, M., Nadeem, S. M., Asghar, H. N. (2009). Comparative effectiveness of Pseudomonas and Serratia sp. containing ACC-deaminase for improving growth and yield of wheat (Triticum aestivum L.) under salt-stressed conditions. Archives of Microbiology, 191(5), 415–424. https://doi.org/10.1007/s00203-009-0466-y [Google Scholar] [PubMed] [CrossRef]
87. Kapadia, C., Patel, N., Rana, A., Vaidya, H., Alfarraj, S. et al. (2022). Evaluation of plant growth-promoting and salinity ameliorating potential of halophilic bacteria isolated from saline soil. Frontiers in Plant Science, 13, 946217. https://doi.org/10.3389/fpls.2022.946217 [Google Scholar] [PubMed] [CrossRef]
88. Kusale, S. P., Attar, Y. C., Sayyed, R. Z., Malek, R. A., Ilyas, N. et al. (2021). Production of plant beneficial and antioxidants metabolites by Klebsiella variicola under salinity stress. Molecules, 26(7), 1894. https://doi.org/10.3390/molecules26071894 [Google Scholar] [PubMed] [CrossRef]
89. Bisht, S., Singh, S., Singh, M., Sharma, J. G. (2022). Augmentative role of Piriformospora indica fungus and plant growth promoting bacteria in mitigating salinity stress in Trigonella foenum-graecum. Journal of Applied Biology and Biotechnology, 10(1), 85–94. https://doi.org/10.7324/JABB.2021.100111 [Google Scholar] [CrossRef]
90. AlKahtani, M. D. F., Hafez, Y. M., Attia, K., Al-Ateeq, T., Ali, M. et al. (2021). Bacillus thuringiensis and silicon modulate antioxidant metabolism and improve the physiological traits to confer salt tolerance in lettuce. Plants, 10(5), 1025. https://doi.org/10.3390/plants10051025 [Google Scholar] [PubMed] [CrossRef]
91. Sapre, S., Gontia-Mishra, I., Tiwari, S. (2018). Klebsiella sp. confers enhanced tolerance to salinity and plant growth promotion in oat seedlings (Avena sativa). Microbiology Research, 206, 25–32. https://doi.org/10.1016/j.micres.2017.09.009 [Google Scholar] [PubMed] [CrossRef]
92. Nadeem, S. M., Zahir, Z. A., Naveed, M., Asghar, H. N., Arshad, M. (2010). Rhizobacteria capable of producing ACC-deaminase may mitigate salt stress in wheat. Soil Science Society of America Journal, 74(2), 533–542. https://doi.org/10.2136/sssaj2008.0240 [Google Scholar] [CrossRef]
93. Sapre, S., Gontia-Mishra, I., Tiwari, S. (2021). Plant growth promoting rhizobacteria ameliorates salinity stress in pea (Pisum sativum). Journal Plant Growth Regulation, 41(2), 647–656. https://doi.org/10.1007/s00344-021-10329-y [Google Scholar] [CrossRef]
94. Ansari, F., Jabeen, M., Ahmad, I. (2021). Pseudomonas azotoformans FAP5, a novel biofilm forming PGPB strain, alleviates drought stress in wheat plant. International Journal of Environmental Science and Technology, 18(12), 1–16. https://doi.org/10.1007/s13762-020-03045-9 [Google Scholar] [CrossRef]
95. Tufail, M. A., Touceda-Gonzá, M., Pertot, I., Ehlers, R. U. (2021). Gluconacetobacter diazotrophicus Pal5 enhances plant robustness status under the combination of moderate drought and low nitrogen stress in Zea mays L. Microorganisms, 9(4), 870. https://doi.org/10.3390/microorganisms9040870 [Google Scholar] [PubMed] [CrossRef]
96. Gupta, A., Rai, S., Bano, A., Khanam, A., Sharma, S. et al. (2021). Comparative evaluation of different salt tolerant plant growth promoting bacterial isolates in mitigating the induced adverse effect of salinity in pisum sativum. Biointerface Research in Applied Chemistry, 11(5), 13141–13154. https://doi.org/10.33263/BRIAC115.1314113154 [Google Scholar] [CrossRef]
97. Patel, D., Jha, C. K., Tank, N., Saraf, M. (2012). Growth enhancement of chickpea in saline soils using plant growth promoting rhizobacteria. Journal Plant Growth Regulation, 31(1), 53–62. https://doi.org/10.1007/s00344-011-9219-7 [Google Scholar] [CrossRef]
98. Yasmin, H., Naeem, S., Bakhtawar, M., Jabeen, Z., Nosheen, A. et al. (2020). Halo-tolerant rhizobacteria Pseudomonas pseudoalcaligenes and Bacillus subtilis mediate systemic tolerance in hydroponically grown soybean (Glycine max L.) against salinity stress. PLoS One, 15(4), e0231348. https://doi.org/10.1371/journal.pone.0231348 [Google Scholar] [PubMed] [CrossRef]
99. Ahammed, G. J., Xu, W., Liu, A., Chen, S. (2018). COMT1 silencing aggravates heat stress-induced reduction in photosynthesis by decreasing chlorophyll content, photosystem II activity, and electron transport efficiency in tomato. Frontiers in Plant Science, 9, 998. https://doi.org/10.3389/fpls.2018.00998 [Google Scholar] [CrossRef]
100. Kim, M., Lee, U., Small, I., des Francs Small, C. C., Vierling, E. (2012). Mutations in an Arabidopsis mitochondrial transcription termination factor-related protein enhance thermo-tolerance in the absence of the major molecular chaperone HSP101. Plant Cell, 24(8), 3349–3365. https://doi.org/10.1105/tpc.112.101006 [Google Scholar] [PubMed] [CrossRef]
101. Ashraf, M., Harris, P. J. C. (2013). Photosynthesis under stressful environments: An overview. Photosynthetica, 51(2), 163–190. https://doi.org/10.1007/s11099-013-0021-6 [Google Scholar] [CrossRef]
102. Mukhtar, T., Ali, F., Rafique, M., Ali, J., Afridi, M. S. et al. (2023). Biochemical characterization and potential of Bacillus safensis Strain SCAL1 to mitigate heat stress in Solanum lycopersicum L. Journal of Plant Growth Regulation, 42(1), 523–538. https://doi.org/10.1007/s00344-021-10571-4 [Google Scholar] [CrossRef]
103. Shaffique, S., Khan, M. A., Wani, S. H., Pande, A., Imran, M. et al. (2022). A review on the role of endophytes and plant growth promoting rhizobacteria in mitigating heat stress in plants. Microorganisms, 10, 1286. https://doi.org/10.3390/microorganisms10071286 [Google Scholar] [PubMed] [CrossRef]
104. Issa, A., Esmaeel, Q., Sanchez, L., Courteaux, B., Guise, J. F. et al. (2018). Impacts of Paraburkholderia phytofirmans strain PsJN on tomato (Lycopersicon esculentum L.) under high temperature. Frontiers in Plant Science, 9, 1397. https://doi.org/10.3389/fpls.2018.01397 [Google Scholar] [PubMed] [CrossRef]
105. Meena, H., Ahmed, M. A., Pravin, P. (2015). Amelioration of heat stress in wheat, Triticum aestivum by PGPB (Pseudomonas aeruginosa strain 2CpS1). Bioscience Biotechnology Research Communications, 8(2), 171174. [Google Scholar]
106. Alexandre, A., Oliveira, S. (2011). Most heat-tolerant rhizobia show high induction of major chaperone genes upon stress. FEMS Microbiol Ecology, 75, 2836. https://doi.org/10.1111/j.1574-6941.2010.00993.x [Google Scholar] [PubMed] [CrossRef]
107. Li, S. S., Li, S., Zhang, M. L., Wang, D., Li, S. et al. (2023). Bacillus licheniformis (BE-L60) improved tolerance of spinach seedlings against heat stress. Russian Journal of Plant Physiology, 70(4), 65. [Google Scholar]
108. Duarte, B., Carreiras, J., Fonseca, B., de Carvalho, R. C., Matos, A. R. et al. (2023). Improving Salicornia ramosissima photochemical and biochemical resilience to extreme heatwaves through rhizosphere engineering with plant growth-promoting bacteria. Plant Physiology and Biochemistry, 199, 107725. https://doi.org/10.1016/j.plaphy.2023.107725 [Google Scholar] [PubMed] [CrossRef]
109. Kumawat, K. C., Nagpal, S., Sharma, P. (2022). Potential of plant growth promoting rhizobacteria plant interactions in mitigating salt stress for sustainable agriculture: A review. Pedosphere, 32(2), 223–245. https://doi.org/10.1016/S1002-0160(21) [Google Scholar] [CrossRef]
110. Kumawat, K. C., Sharma, B., Nagpal, S., Kumar, A., Tiwari, S. et al. (2023). Plant growth-promoting rhizobacteria: Salt stress alleviators to improve crop productivity for sustainable agriculture development. Frontiers in Plant Science, 13, 1101862. https://doi.org/10.3389/fpls.2022.1101862 [Google Scholar] [PubMed] [CrossRef]
111. Kumar, A., Singh, S., Gaurav, A. K., Srivastava, S., Verma, J. P. (2020). Plant growth promoting bacteria: Biological tools for the mitigation of salinity stress in plants. Frontiers in Microbiology, 11, 1216. https://doi.org/10.3389/fmicb.2020.01216 [Google Scholar] [PubMed] [CrossRef]
112. Akbar, A., Han, B., Khan, A. H., Feng, C., Ullah, A. et al. (2022). A transcriptomic study reveals salt stress alleviation in cotton plants upon salt tolerant PGPB inoculation. Environmental and Experimental Botany, 200, 104928. https://doi.org/10.1016/j.envexpbot.2022.104928 [Google Scholar] [CrossRef]
113. Jalmi, S. K., Sinha, A. K. (2022). Ambiguities of PGPB induced plant signaling and stress management. Frontiers in Microbiology, 13, 899563. https://doi.org/10.3389/fmicb.2022.899563 [Google Scholar] [PubMed] [CrossRef]
114. Ilangumaran, G., Smith, D. L. (2017). Plant growth promoting rhizobacteria in amelioration of salinity stress: A systems biology perspective. Frontiers in Plant Science, 8, 1768. https://doi.org/10.3389/fpls.2017.01768 [Google Scholar] [PubMed] [CrossRef]
115. Chen, L., Liu, Y., Wu, G., Veronican, N. K., Shen, Q. et al. (2016). Induced maize salt tolerance by rhizosphere inoculation of Bacillus amyloliquefaciens SQR9. Physiologia Plantarum, 158(1), 34–44. https://doi.org/10.1111/ppl.12441 [Google Scholar] [PubMed] [CrossRef]
116. Marulanda, A., Azcon, R., Chaumont, F., Ruiz-Lozano, J. M., Aroca, R. (2010). Regulation of plasma membrane aquaporins by inoculation with a Bacillus megaterium strain in maize (Zea mays L.) plants under unstressed and salt stressed conditions. Planta, 232(2), 533–543. https://doi.org/10.1007/s00425-010-1196-8 [Google Scholar] [PubMed] [CrossRef]
117. Kim, K., Jang, Y. J., Lee, S. M., Oh, B. T., Chae, J. C. et al. (2014). Alleviation of salt stress by Enterobacter sp. EJ01 in tomato and Arabidopsis is accompanied by up-regulation of conserved salinity responsive factors in plants. Molecules and Cells, 37(2), 109–117. https://doi.org/10.14348/molcells.2014.2239 [Google Scholar] [PubMed] [CrossRef]
118. Habib S. H., Kausar H., Saud H. M. (2016). Plant growth promoting rhizobacteria enhance salinity stress tolerance in okra through ROS scavenging enzymes. BioMed Research International. https://doi.org/10.1155/2016/6284547 [Google Scholar] [PubMed] [CrossRef]
119. Bharti, N., Pandey, S. S., Barnawal, D., Patel, V. K., Kalra, A. (2016). Plant growth promoting rhizobacteria Dietzia natronolimnaea modulates the expression of stress responsive genes providing protection of wheat from salinity stress. Scientific Reports, 6(1), 34768. https://doi.org/10.1038/srep34768 [Google Scholar] [PubMed] [CrossRef]
120. Nautiyal, C. S., Srivastava, S., Chauhan, P. S., Seem, K., Mishra, A. et al. (2013). Plant growth-promoting bacteria Bacillus amyloliquefaciens NBRISN13 modulates gene expression profile of leaf and rhizosphere community in rice during salt stress. Plant Physiology and Biochemistry, 66, 1–9. https://doi.org/10.1016/j.plaphy.2013.01.020 [Google Scholar] [PubMed] [CrossRef]
Cite This Article
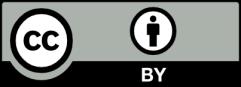