Open Access
REVIEW
New insight into the role of exosomes in idiopathic membrane nephropathy
1 Department of Nephrology, Shaanxi Provincial Hospital of Traditional Chinese Medicine, Xian, 710000, China
2 School of Basic Medical Sciences and State Key Laboratory of Southwestern Chinese Medicine Resources, Chengdu University of Traditional Chinese Medicine, Chengdu, 611137, China
3 Department of Pathophysiology, West China College of Basic Medical Sciences & Forensic Medicine, Sichuan University, Chengdu, 610041, China
* Corresponding Author: XIAOHONG CHENG. Email:
# These authors contributed equally to this work
(This article belongs to the Special Issue: Advances in Biomarker Research: Unveiling the Pathways to Precision Medicine)
BIOCELL 2024, 48(1), 21-32. https://doi.org/10.32604/biocell.2023.045631
Received 02 September 2023; Accepted 07 November 2023; Issue published 30 January 2024
Abstract
Exosomes, nanoscale extracellular vesicles (EVs) derived from the invagination of the endosomal membrane, are secreted by a majority of cell types. As carriers of DNA, mRNA, proteins, and microRNAs, exosomes are implicated in regulating biological activities under physiological and pathological conditions. Kidney-derived exosomes, which vary in origin and function, may either contribute to the pathogenesis of disease or represent a potential therapeutic resource. Membranous nephropathy (MN), an autoimmune kidney disease characterized by glomerular damage, is a predominant cause of nephrotic syndrome. Notably, MN, especially idiopathic membranous nephropathy (IMN), often results in end-stage renal disease (ESRD), affecting approximately 30% of patients and posing a considerable economic challenge to healthcare systems. Despite substantial research, therapeutic options remain ineffective at halting IMN progression, underscoring the urgent need for innovative strategies. Emerging evidence has implicated exosomes in IMN’s pathophysiology; Providing a fresh perspective for the discovery of novel biomarkers and therapeutic strategies. This review aims to scrutinize recent developments in exosome-related mechanisms in IMN and evaluate their potential as promising therapeutic targets and diagnostic biomarkers, with the hope of catalyzing further investigations into the utility of exosomes in MN, particularly IMN, ultimately contributing to improved patient outcomes in these challenging disease settings.Graphic Abstract
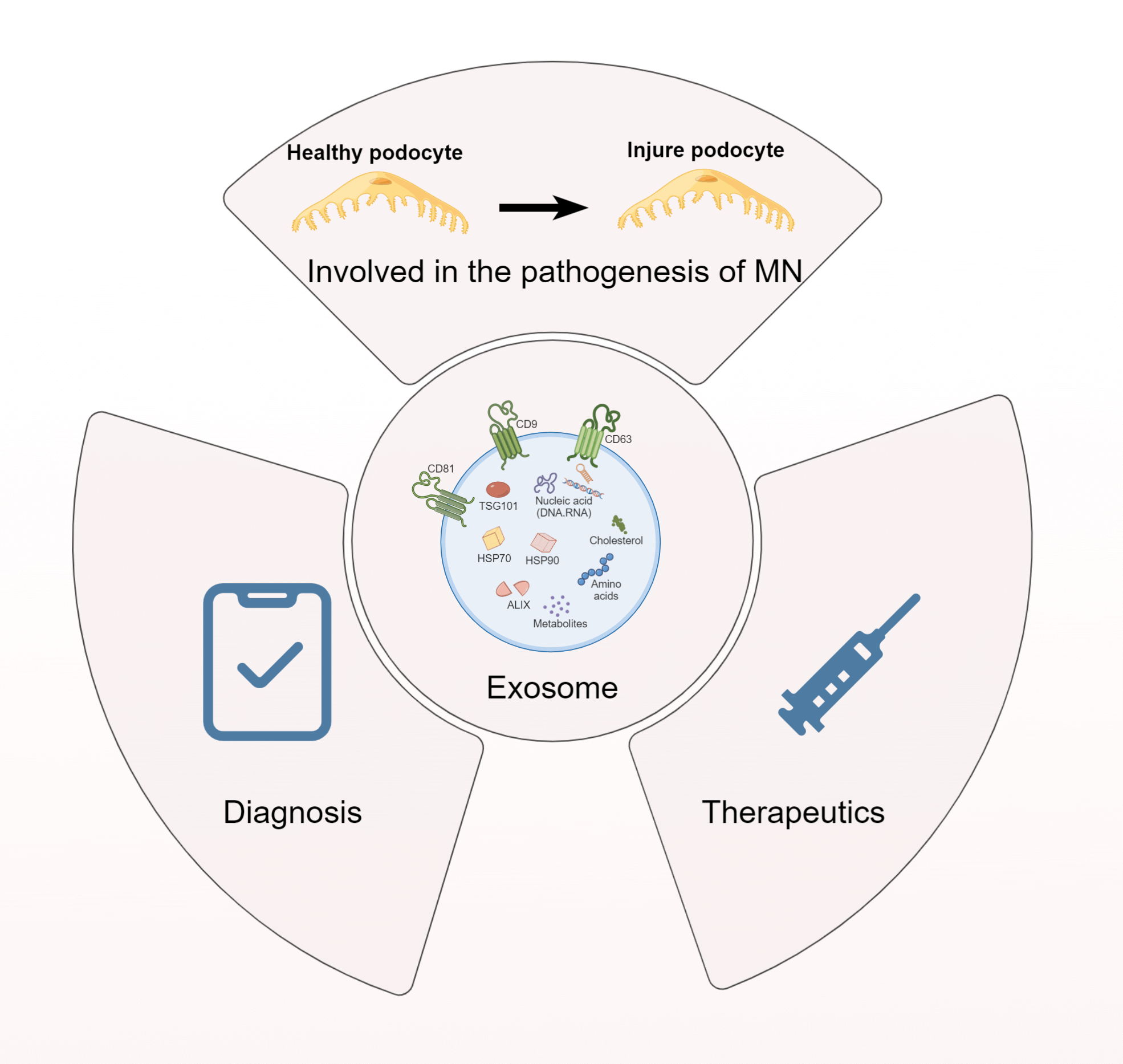
Keywords
Abbreviations
EVs | Extracellular vesicles |
MN | Membranous nephropathy |
ESRD | End-stage renal disease |
NS | Nephrotic syndrome |
IMN | Idiopathic membranous nephropathy |
PMN | Primary membranous nephropathy |
SMN | Secondary membranous nephropathy |
EM | Electron microscopy |
GBM | Glomerular basement membrane |
GFB | Glomerular filtration barrier |
NSAIDs | Nonsteroidal anti-inflammatory drugs |
MVBs | Multivesicular bodies |
ILVs | Intraluminal vesicles |
MSCs | Mesenchymal stem cells |
ESCRT | Endosomal sorting complexes required for transport |
PLA2R | M-type phospholipase A2 receptor |
THSD7A | Thrombospondin type-1 domain-containing 7A |
Treg | regulatory T |
DAF | Decay accelerating factor |
MCP | Membrane cofactor protein |
CR1 | Complement receptor 1 |
GFR | Glomerular filtration rate |
LIMP-2 | Lysosome membrane protein 2 |
MUC3A | Mucin 3A |
Membranous nephropathy (MN) is a leading etiology of adult nephrotic syndrome (NS) and a significant contributor to primary glomerulonephritis [1,2]. Approximately 80% of MN patients suffer from idiopathic membranous nephropathy (IMN) or primary membranous nephropathy (PMN), which is devoid of an evident secondary cause. This renal disorder is more prevalent among the middle-aged and elderly demographics, even though it can manifest across all age brackets [2,3]. MN is characterized by edema, severe proteinuria, hypoalbuminemia, and hyperlipidemia. However, significant hematuria is rarely observed [4,5]. Clinically, approximately one-third of patients progress to ESRD within 5–10 years, while another third remain in a state of microalbuminuria and the remaining third experience spontaneous remission [6–8]. The disease exhibits distinctive pathological features under optical microscopy, including diffuse glomerular lesions with thickened capillary walls, eosinophilic deposits on the epithelial lateral surface, and “spike” (stage II) or “chain ring” (stage III) changes. Immunofluorescence microscopy reveals fine granular deposits of IgG and complement C3 along the glomerular capillary wall. Electron microscopy (EM) examination reveals electron-dense deposits beneath the epithelium and extensive fusion of podocyte foot processes. Ehrenreich and Churg proposed a histomorphological classification for stages I through IV based on immunological deposits and glomerular basement membrane (GBM) thickness observed by EM [9]. Secondary membranous nephropathy (SMN) is a complex and multifactorial condition that affects the kidneys. It is characterized by inflammation and damage to the glomeruli, which are tiny structures in the kidney responsible for filtering waste products from the blood. There are several factors that can contribute to the development of SMN, including autoimmune diseases such as lupus or rheumatoid arthritis, infections like hepatitis B or C, malignancies such as lymphoma or leukemia, drug use including nonsteroidal anti-inflammatory drugs (NSAIDs), antibiotics and anticonvulsants, and exposure to heavy metals like mercury or lead. Yet, SMN forms nearly 20% of IMN cases, signifying its significant prevalence [10–13]. The inconsistent patient outcomes in IMN pose therapeutic challenges, emphasizing the pressing need for advanced biomarkers to accurately predict disease trajectory and outcomes [14].
Exosomes are extracellular vesicles that play a significant role in intercellular communication by encapsulating proteins and nucleic acids, such as single-stranded DNAs, double-stranded DNAs, mitochondrial DNAs, mRNAs, and microRNAs. Ranging from 40 to 160 nm in size, they serve as important mediators of cell-to-cell communication [15,16], which originate from small intraluminal vesicles within multivesicular bodies (MVBs), undergo fusion with the cell’s plasma membrane to release intraluminal vesicles (ILVs) into the intercellular space [17]. Exosome biogenesis facilitates the transportation of various receptors, proteins, genetic materials, and lipids to target cells. Recent studies have investigated the components of urinary exosomes originating from various nephron segments and their correlation with renal physiology and pathophysiology, including serum/plasma/blood, renal tubular cells, renal tissues, glomerular endothelial cells, mesenchymal stem cells (MSCs), urinary stem cells, and macrophages in kidney diseases are being explored [18–20]. Given these findings, the central research question guiding this review is: “How do exosomes contribute to the pathogenesis, diagnosis, and potential therapeutic interventions of IMN?” By focusing on this central theme, we aim to synthesize and critically assess the current knowledge on the multifaceted roles of exosomes in IMN.
In the late 1960s, Bonucci and Anderson reported that chondrocytes secrete small vesicles measuring 100 nm, marking the initial documentation of small, secreted vesicles [21,22]. Later on in 1981, Trams et al. utilized transmission electron microscopy to scrutinize exosomes [23]. Six years later, Pan et al. were able to provide a more precise characterization of exosomes through their analysis of reticulocyte maturation processes in sheep [24]. However, initially these cells were dismissed as mere “waste” and their potential role was not immediately recognized by researchers.
Exosomes are currently recognized as lipid bilayers with a width ranging from 40–160 nm. Exosome formation begins within the endosomal system of the cell. When a portion of the plasma membrane undergoes invagination, early endosomes are formed. Within the early endosomes, there are further invaginations of the limiting membrane, leading to the formation of ILVs inside. As more ILVs accumulate within an early endosome, it matures into a MVBs or late endosome [25,26]. MVBs ultimately merge with the plasma membrane, liberating ILVs into the extracellular milieu. When ILVs are released this way, they are termed exosomes. In addition, the production of exosomes is primarily regulated by the endosomal sorting complexes required for transport (ESCRT), machinery, which consists of four distinct components: ESCRT-0, ESCRT-I, ESCRT-II and ESCRT-III [27,28]. Moreover, MVBs undergo relocalization to the plasma membrane under the guidance of small RabGTPase proteins [29]. This protein family also participates in the recycling of endosomes, trafficking of multivesicular bodies, and transit of intracellular vesicles [30] (Fig. 1).
FIGURE 1: The journey of exosome formation, maturation, and release within a cell.
Beginning with the nucleus as the epicenter of cellular information, the synthesis of proteins and lipids is initiated in the endoplasmic reticulum. As these molecules mature and undergo post-translational modifications, they traverse through the trans-Golgi network, emphasizing the seamless continuum of intracellular trafficking. Concurrently, the cell’s energy metabolism is anchored by the depicted mitochondrion. The structural scaffold, maintained by actin filaments, not only provides architectural stability but also coordinates with embedded cell surface proteins to mediate endocytosis. This uptake of extracellular materials swiftly transitions into a systematic and orchestrated sorting within the endosomal compartments. With the critical guidance of the ESCRT machinery, select cargoes are compartmentalized into intraluminal vesicles within the multivesicular body (MVB). These MVBs, guided by the navigational cues from RabGTPase proteins and poised amidst the cellular waste management pathways of lysosomes, eventually align with the plasma membrane. In a harmonized finale, the MVBs fuse, releasing exosomes—cellular messengers replete with proteins, RNA, DNA, amino acids, and metabolites—into the extracellular space, symbolizing the cell’s mastery in intercellular communication and adaptative signaling.
Exosomes carry a multifaceted molecular cargo, encompassing DNA, RNA, proteins, lipids, microRNAs, small RNAs and non-coding RNAs that are subject to variation based on the originating cell [31]. These cargoes are strictly regulated by the parent cell and serve as a conduit for transmitting specific cellular information to acceptor cells [15]. Despite their heterogeneity in terms of size, composition, and biological origin, exosomes have attracted significant attention in research due to their potential roles in diagnosing and treating various diseases such as neurodegenerative disorders, metabolic diseases, and cancer [32–36]. Surface engineering of exosomes for targeted drug delivery has also been explored, showcasing promising strategies for the diagnosis and treatment of diseases [37–39].
The isolation and extraction of exosomes constitute pivotal initial steps in exploring their functions. Various sophisticated techniques have been developed for exosome isolation and purification, including immunoaffinity capture, density gradient ultracentrifugation, size exclusion chromatography, polymer precipitation, and ultracentrifugation (Fig. 2) [40]. For instance, immunoaffinity capture leverages the specific binding of antibodies to exosomal surface proteins for isolation, density gradient ultracentrifugation separates exosomes based on their buoyant densities, size exclusion chromatography employs a gel filtration technique to isolate exosomes based on their size, polymer precipitation induces the aggregation of exosomes, facilitating their subsequent centrifugation, ultracentrifugation is a common technique that separates exosomes by spinning a liquid sample at high speed. Each technique offers distinct advantages and potential drawbacks, necessitating meticulous consideration in accordance with the research objectives. While certain methods may yield greater exosome purity, others may prove more efficient or less labor-intensive. Therefore, selecting an appropriate isolation and extraction method is crucial to successfully studying exosome functions.
FIGURE 2: Icons show the extraction method of exosomes.
Exosomes are Involved in the Pathogenesis of IMN
In patients with IMN, the development of autoimmunity occurs due to circulating autoantibodies binding to glomerular podocyte antigens. This binding is the most crucial mechanism for the development of IMN. Currently, it is known that autoantibodies bind to antigens such as M-type phospholipase A2 receptor (PLA2R), thrombospondin type-1 domain-containing 7A (THSD7A), and other podocyte antigens, forming basement membrane subepithelial deposits and activating complement [41–43]. This process ultimately damages podocytes, leading to proteinuria [44]. Autoantibodies are generated against both endogenous and exogenous antigens in the early stages of IMN pathogenesis. These antibodies specifically recognize antigens that are inherently expressed on podocytes in IMN, leading to the formation of subepithelial immune complexes upon binding with endogenous antigens [45]. Although the subepithelial deposition of circulating immune complexes may potentially serve as a disease-initiating mechanism in IMN, its confirmation in patients remains elusive [46]. From previous research, the development of autoantibodies can span years or even decades prior to the clinical manifestation of disease, rendering it a formidable challenge to investigate the initial immunological responses and reduction in immunological tolerance in IMN (proteinuria or edema) [47]. Levels of anti-PLA2R antibodies typically rise a few months before a diagnosis of idiopathic membranous nephropathy in patients. The development of clinical symptoms may hinge on a multitude of factors, including the quantity of immune complexes deposited in the subepithelial space, the antibody’s ability to traverse the GBM, specific epitope-binding capacity, and IgG subclass composition, all in addition to circulating anti-PLA2R antibody levels. However, it remains uncertain whether these characteristics vary depending on the implicated antigens and associated autoantibodies [48].
Although the exact mechanism remains elusive, exosomes are believed to play a pivotal role in the pathogenesis of IMN. These roles include: exosome-mediated transfer of antibodies or antigens, facilitation of intercellular communication, immunomodulation, inflammatory response, and podocyte injury. Notably, these effects are believed to reinforce each other.
While IMN is caused by autoantibodies binding to podocyte antigens, the mystery lies in how these autoantibodies penetrate the subepithelial area, given that the glomerular filtration barrier (GFB) is highly selective and virtually impenetrable to antibodies. Mario Schiffer’s team has tackled this issue and discovered that patients with IMN have elevated levels of GEC-derived miR-192-5p and podocyte-derived miR-378a-3p in their urine and glomeruli, while glomerular NPNT is reduced. In a paracrine manner, GECs use exosomes containing miR-192-5p to inhibit podocyte NPNT transmission. Diminished NPNT induces structural modifications in the GBM, characterized by intensified luminosity, division and stratification, particularly in the atypical inner layer resembling the ultrastructure of advanced MN. The reduction of NPNT leading to increased GBM permeability may exert a pivotal role in IMN pathophysiology by facilitating podocyte antigen presentation and enabling autoantibodies to infiltrate into the subepithelial region [49] (Fig. 2). At this juncture, exosomes appear to assume a ‘treacherous’ role in the progression of the ailment.
Exosome isolation techniques vary depending on the source and intended use of these extracellular particles. Some common methods include (A) Schematic representation of the exosome isolation procedure using ultracentrifugation. The process begins with the collection of cell supernatant, followed by a centrifugal decellularization step to remove whole cells. Subsequently, centrifugal debris removal is performed to eliminate cellular fragments and other impurities. This leads to the accumulation of precipitated exosomes at the bottom. After a final purification step, purified exosomes are obtained, ready for downstream analyses or applications. (B) Schematic representation of the exosome isolation procedure using polymer precipitation. The workflow starts with the extraction of the cell supernatant. This is followed by centrifugal decellularization to exclude whole cells. The next phase involves centrifuge debris removal, eliminating cellular fragments and potential contaminants. Afterward, the sample undergoes coincubation with polymers, facilitating the aggregation and subsequent precipitation of exosomes. (C) Schematic representation of the exosome isolation procedure using immunoaffinity capture. This method employs specific antibodies targeted against exosomal markers such as CD9, CD63, and CD81. Initially, the sample is exposed to immobilized antibodies that recognize these markers. Upon binding, the exosomes are selectively captured while non-targeted components are washed away. This results in a highly specific collection of exosomes that can be eluted and utilized for downstream analyses. (D) Schematic representation of the exosome isolation procedure using size exclusion chromatography. A mixture containing exosomes is loaded onto a chromatography column packed with porous beads. As the sample travels through the column, larger particles are excluded from entering the pores and elute earlier, while smaller particles permeate into the beads and are delayed. Exosomes, having a specific size range, are then selectively eluted at a distinct fraction, allowing for their effective separation from other components. Each method has its own unique set of advantages and limitations that must be carefully considered when selecting an appropriate protocol.
In another aspect, podocytes may secrete exosomes to interact with other cells, such as immune cells, resulting in immune modulation, complement activation and other processes. For instance, it has been reported that dendritic cells can release exosomes containing antigen-presenting characteristics and peptide-MHC complexes which facilitate the activation of both dendritic cells and T cells [50]. However, there is insufficient literary evidence to substantiate whether this holds true for foot cells. Furthermore, T cells play a pivotal role in the development of autoimmunity. Several studies conducted on individuals with IMN have demonstrated alterations in T cell subpopulations [51]. Patients with IMN exhibit an elevated frequency of T helper 17 cells and a decreased frequency of regulatory T (Treg) cells in their serum, as compared to healthy individuals. However, this imbalance can be rectified through appropriate proteinuria treatment [52,53]. Another clinical report has revealed that the severity of IMN is closely associated with the number of follicular helper T cells, as well as plasma cells and regulatory B cells [54–56]. Intriguingly, a vast body of literature attests to the direct or indirect activation of T cells by exosomes. For instance, one study revealed that tumor-derived exosomes can downregulate CD3 in primary activated T cells and induce apoptosis in CD8+ T cells [57]. A separate investigation revealed that during homologous interactions between T cells and dendritic cells, exosomes secreted by the latter into the extracellular milieu specifically target activated T cells within that microenvironment [58]. Furthermore, it has been reported that exosomes possess the ability to present antigens to dendritic cells and bind with TLR2/3, thereby initiating dendritic cell activation through the NF-κB signaling pathway. This ultimately leads to an imbalance in CD4+ T lymphocyte differentiation [59].
In addition to that, complement activation plays a crucial role in the pathophysiology of MN. Complement components, including C1q, C3, C4d and C5b-9, are frequently detected within the immunological deposits of MN patients alongside proteins from both classical and alternative complement pathways [60]. This has been demonstrated in a rat model of Heymann nephritis, where immunological deposits triggered the activation of complement system components, including C3 and C5b-9 deposition [61,62]. Currently, mounting evidence suggests the pivotal role of exosomes in this process. For example, a study on Diabetic Retinopathy (DR) revealed that plasma-borne exosomes carrying IgG trigger the classical complement pathway. Interestingly, an increase in these IgG-laden exosomes was observed in diabetes, exacerbating the pathological damage associated with DR [63]. In a separate investigation, it has been revealed that exosomes derived from tumors can activate the complement system via a calcium-sensitive pathway, demonstrating a concentration-dependent effect. This activation subsequently enhances the metastatic dissemination of the tumor [64]. Exosomes secreted by platelets, leukocytes, and red blood cells collectively act as mediators of the pathogenic aspects of complement activation. They can induce complement activation on their parental cells or explicitly accumulate complement on their own surface [65–67]. This behavior is expected to be more prevalent in individuals with complement-mediated diseases rather than those who are healthy. Under physiological conditions, the excessive activation of complement on exosomes derived from blood is regulated by various complement regulators, such as decay accelerating factor (DAF), membrane cofactor protein (MCP), CD59, and complement receptor 1 (CR1) [68].
Lastly, the impact of exosomes on MN development and progression is multifaceted and extends beyond mere facilitation of autoantibody formation. One crucial role they play is in antigen delivery. By carrying and presenting specific antigens, exosomes can trigger localized inflammation within the glomeruli. This inflammation can trigger a cascade of immunological reactions, leading to modifications in the glomerular basement membrane and culminating in the formation of subepithelial deposits, which are characteristic of MN. This process not only contributes to the initial stages of MN but also has the potential to drive its progression. In the presence of persistent inflammation and formation of immune complexes, renal damage can intensify, resulting in a decline in renal function and the progressive nature of MN. Additionally, exosomes may also play a role in regulating glomerular filtration by means of signaling pathways that are mediated by these tiny vesicles, which could potentially increase the glomerular filtration rate (GFR). The glomerular filtration rate (GFR) is of paramount importance to the proper functioning of the kidneys, and any deviations from this critical parameter have been linked to the onset of nephrotic syndrome, a common affliction among those with MN. Exosome-mediated mechanisms facilitating an increase in GFR may potentially exacerbate proteinuria, a significant clinical manifestation of MN. Additionally, these exosomes may transport molecules capable of modulating the permeability of glomerular capillaries, indirectly influencing the filtration process and worsening the severity of nephrotic syndrome.
Consequently, a thorough grasp of the diverse roles exosomes play in MN pathogenesis, encompassing aspects such as antigen presentation, immunomodulation, inflammatory response, and podocyte injury, among others, could offer fresh perspectives on disease mechanisms and uncover potential therapeutic avenues. Nevertheless, more extensive research is crucial to conclusively determine the depth of exosome participation in MN and evaluate their viability as therapeutic targets.
Roles of Exosomes in the Diagnosis of IMN
To this day, renal biopsy remains the diagnostic gold standard for IMN. Its unparalleled accuracy provides a definitive diagnosis, but the procedure comes with its own set of challenges. The invasive nature of a renal biopsy, combined with its specific puncture requirements and potential complications, makes it a less than ideal choice for routine diagnosis, especially for monitoring disease progression or therapeutic responses.
With the identification of PLA2R and THSD7A as target antigens for IMN, diagnosis has been refined to align with the disease’s underlying pathology [69]. PLA2R and THSD7A exhibit a remarkable degree of specificity towards IMN [43,70,71]. As an illustration, a study revealed that IMN was present in 129 out of 132 patients with anti-PLA2R antibody-positive status following renal biopsies [72]. In a meta-analysis of 15 trials, the sensitivity and specificity of anti-PLA2R antibody detection were found to be 78% and 99%, respectively [73]. Similarly, antibodies against THSD7A have not been detected in healthy individuals or patients with other renal and systemic diseases, demonstrating 100% specificity for MN damage. However, a previous study found that among a group of 42 patients with PLA2R antigen-positive immune complexes in renal biopsies, 10 individuals had no detectable blood antibodies. In a separate investigation, the presence of anti-PLA2R antibody was only detected in 73 out of 217 IMN patients (33.6%) in Japan [74]. According to these studies, while anti-PLA2R antibodies may persist in some individuals for years without manifesting any significant clinical symptoms, in other patients their levels escalate and become detectable only a few months prior to an MN diagnosis. One possible explanation for this phenomenon is that despite the strong affinity of anti-PLA2R antibody towards its antigen, it remains unrecognized until all binding sites are occupied [75]. Therefore, it serves as a poignant reminder that despite the efficacy of anti-PLA2R and anti-THSD7A antibodies in IMN diagnosis, limitations persist.
Exosomes, especially those derived from urine, present a promising non-invasive method for IMN diagnosis. Their stability, combined with the ease of collection, offers significant advantages over traditional methods. The cost-effectiveness of exosome-based tests, given their non-invasive nature, makes them an attractive option for routine diagnosis and monitoring. Exosomes remain stable at 4°C for 24 h prior to storage at −80°C, while those derived from urine maintain stability for up to 12 months when stored under similar conditions, as reported by Lv et al. Remarkably, even after undergoing five freeze-thaw cycles, exosomal miRNAs remain detectable [76,77]. Urinary exosomes, accumulating evidence suggests, hold great potential as noninvasive indicators for the diagnosis of IMN [78,79]. For example, proteomic studies have offered valuable insights into the role of proteins in urinary exosomes. One notable protein is lysosome membrane protein 2 (LIMP-2), which is found in abundance in the urinary microvesicles of IMN patients. This presence correlates with an increased expression of LIMP-2 in the glomerulus. Additionally, the co-localization of LIMP-2 and IgG on the glomerular basement membrane has been observed, pointing towards the significance of urinary exosomes in biomarker discovery. Such findings have established the de novo expression of LIMP-2 in the glomeruli affected by IMN [80].
Interestingly, MicroRNAs (miRNAs) carried by urinary exosomes have been the focus of several studies. The researchers have discovered that IMN patients exhibit increased levels of miR-192-5p and miR-378a-3p in their urine and glomeruli, accompanied by a corresponding reduction in glomerular NPNT. By delivering exosomes carrying miR-192-5p, glomerular endothelial cells have inhibited podocyte NPNT through paracrine signaling. This interaction has resulted in structural alterations within the GBM, particularly affecting its inner layer (Fig. 3) [49]. Furthermore, a high-throughput sequencing analysis has revealed that miRNAs present in urine exosomes are the most efficacious biomarkers for distinguishing IMN in adults. The authors discovered significant disparities in the urinary exosome miRNA expression profile between individuals with IMN and those who are healthy. MiR-9-5p exhibited a close correlation with various clinical indicators of IMN, including serum albumin, triglycerides, and β2-microglobulin, among others. Additionally, MiR-30b-5p demonstrated a positive correlation with anti-phospholipase A2 receptor antibodies. Therefore, miR-30b-5p and miR-9-5p may serve as novel non-invasive biomarkers for IMN [81]. The results of another high-throughput sequencing analysis have revealed significant differences in the expression patterns of urinary exosomal miRNAs and repetitive region-derived sRNAs between patients with IMN and healthy individuals [82].
FIGURE 3: Exosomes are involved in the pathogenesis of primary membranous nephropathy.
On glomerular podocytes, the endogenous antigens phospholipase A2 receptor 1 (PLA2R1) and thrombospondin type 1 domaincontaining protein 7A (THSD7A) are expressed, whereas, GEC blocks podocyte NPNT via paracrine transmission of exosomes containing miR-192-5p. Reduced NPNT as a result of GBM permeability may permit autoantibodies to enter the subepithelial region. As revealed for PLA 2R1 and THSD7A, primary membranous nephropathy (MN) is initiated by the binding of circulating antibodies to endogenous antigens expressed on glomerular podocytes. Antibody binding induces the production of immunological deposits in the subepithelial space and in the glomerular basement membrane (GBM), which might affect the integrity of the podocyte cytoskeleton and result in proteinuria. The presence of immune deposits activates the complement system. The activation of secondary mediators like oxygen radicals and eicosanoids is caused by the development of the terminal complement component C5b-9, commonly known as the membrane assault complex. These mechanisms result in the breakdown of the glomerular filtration barrier and initate proteinuria. These structural alterations are mediated by TGF-induced production of laminin and collagen type IV, as well as the metalloproteinase 9 (MMP9) protein turnover in the GBM.
Recent research has directed attention to the potential of circular RNAs (circRNAs) as diagnostic markers for IMN. Profiling these circRNAs in exosomes from IMN patients’ serum and urine has revealed distinct and specific expression patterns. For instance, MUC3A originating from chr7:100550808|1005501062 could potentially be a diagnostic biomarker for IMN [83]. Another study pinpointed the increased expression of hsa circ 0001250 in IMN, especially in instances of high proteinuria. This circRNA might play a role in IMN’s pathophysiology by targeting specific miRNAs [84] (Table 1).
Urinary exosomes, enriched with specific microRNAs and proteins, offer early detection capabilities for IMN and insights into its progression. As reflections of their parent cell’s biological state, exosomes reveal insights into disease development. Changes in exosomal content can signal disease severity. While we are still learning about exosomal cargo origins and mechanisms, it is essential to discern if these changes are mere disease byproducts or active contributors to IMN. The clinical utility of exosomal biomarkers needs validation in larger patient groups, but they hold promise for non-invasive IMN diagnosis and personalized treatment. Future research should focus on understanding exosome biology and its therapeutic potential for IMN (Table 2).
Roles of Exosomes in Therapeutics Target of IMN
Despite the ability of current therapies to temper the course of IMN, a pervasive linkage remains with a high prevalence of ESRD. This saliently underscores the urgency for more efficacious interventions that could arrest or reverse the progression of IMN. Consequently, the pursuit of innovative, robust renoprotective strategies is of paramount importance. Emerging evidence advocates the potential exploitation of exosomes, given their rich reservoir of mRNAs, miRNAs, and proteins, coupled with their instrumental role in mediating cell-cell communication, as propitious therapeutic delivery vectors [85]. Exosomes, laden with RNA, may induce a transient restoration of perturbed cellular processes by transferring their cargo to recipient cells. For example, studies have demonstrated that specific miRNAs, when transported via exosomes, can modulate molecular pathways integral to the pathogenesis of IMN. However, the acquisition of a comprehensive mechanistic understanding of this process remains indispensable [86]. Moreover, exosomes derived from MSCs have unveiled considerable promise in the IMN treatment landscape, particularly owing to their inherent immunomodulatory properties. By fine-tuning immune cell functionality and attenuating inflammation, MSC-derived exosomes could facilitate the re-establishment of immune equilibrium within the kidneys, thereby potentially ameliorating symptoms and decelerating disease progression [87].
Exosomes show transformative potential for both the diagnosis and treatment of IMN. Their role in mediating cell-cell communication and their rich content of mRNAs, miRNAs, and proteins could offer novel renoprotective strategies for IMN. Moreover, understanding the mechanisms underlying exosome-mediated miRNA and circRNA regulation, through both in vitro and in vivo models, can shed light on their utility in a disease-specific context. MSCs derived exosomes, in particular, hold promise due to their inherent immunomodulatory properties.
However, harnessing this potential is not without challenges. The production of these nano-sized vesicles requires upscaling, and there is a paramount need for pure extraction without contamination from other vesicles. This necessitates robust protocols for exosome production, characterization, and administration, especially given the current lack of standardization in the field. The variable content of exosomes between individuals, influenced by factors such as genetics and environment, could affect diagnostic precision. Additionally, the accurate delivery of exosomes to target tissues, long-term safety concerns, and the cost-prohibitive nature of advanced exosomal techniques pose significant hurdles.
As we continue to explore exosomes’ role in IMN, it is crucial to approach their potential with a comprehensive understanding, considering both their promising aspects and the challenges that need addressing.
Conclusions and Future Perspectives
Idiopathic Membranous Nephropathy presents a significant and intricate public health challenge that affects individuals worldwide. Despite numerous efforts, targeted therapies for IMN have yet to be discovered. Collaboratively, nephrologists and endocrinologists are exploring innovative techniques to promptly and accurately detect renal damage caused by IMN while investigating the disease’s molecular basis to develop groundbreaking treatments.
Recent research on exosomes in IMN has unveiled novel aspects of the disease’s pathogenesis. Exosomes play a pivotal role in facilitating intercellular communication, exerting significant influence on both the onset and progression of IMN. Exosomes released by immune cells and podocytes can incite inflammation and inflict damage upon glomeruli, ultimately leading to the formation of immune complexes and the subsequent development of MN. Exosomes present a significant potential as both diagnostic and therapeutic targets in the management of IMN. Scientists have identified exosomal biomarkers, including specific miRNAs and proteins, which may serve as non-invasive diagnostic tools for MN. Moreover, targeting exosomes offers a promising avenue for the treatment of MN. Preliminary studies suggest that inhibiting either exosome release or uptake could reduce the severity of MN, emphasizing that therapies based on exosomes might prove effective.
However, it is of paramount importance to delve deeper into the molecular mechanisms underlying the role of exosomes in IMN. Understanding these intricacies can provide a clearer picture of the potential therapeutic applications of exosomes in treating IMN. Furthermore, comprehending the challenges in harnessing exosomes for clinical use is indispensable. While the identification of specific exosomal biomarkers linked with the development and progression of MN is crucial for creating efficacious diagnostic tools and therapies, the mechanics governing kidney exosome release and uptake remain vital areas of research for developing potent exosome-centered treatments.
In conclusion, the examination of exosomes in IMN has yielded invaluable insights into the pathogenesis of this disease, emphasizing their potential as both diagnostic and therapeutic targets. Further exploration in this realm may pave the way for revolutionary diagnostic tools and therapies, ultimately enhancing clinical outcomes for patients afflicted with IMN.
Acknowledgement: We would like to thank KetengEdit (www.ketengedit.com) for linguistic assistance and figdraw (www.figdraw.com) for helping make the illustrations during the preparation of this manuscript.
Funding Statement: This work was supported by grants from the National Key Research and Development Program (Grant No. 2019YFC1709404) and the Science and Technology Department of Shaanxi Province (Grant No. 2021LCZX-13).
Author Contributions: Study conception and design: JHL, KH, XHC; data collection: KH, HW; analysis and interpretation of results: JHL, KH, HW; draft manuscript preparation: KH, HW. All authors reviewed the results and approved the final version of the manuscript.
Availability of Data and Materials: All data generated or analyzed during this study are included in this published article. The datasets supporting the conclusions of this article are available in the (PubMed) repository, https://pubmed.ncbi.nlm.nih.gov/.
Ethics Approval: This review article did not involve human participants or animal experiments, therefore ethics approval and consent to participate were not required.
Conflicts of Interest: The authors declare that they have no known competing financial interests or personal relationships that could have appeared to influence the work reported in this paper.
References
1. Ponticelli C, Glassock RJ. Glomerular diseases: membranous nephropathy—a modern view. Clin J Am Soc Nephrol. 2014;9(3):609–16. doi:https://doi.org/10.2215/CJN.04160413. [Google Scholar] [PubMed] [CrossRef]
2. Sinico R, Mezzina N, Trezzi B, Ghiggeri G, Radice A. Immunology of membranous nephropathy: from animal models to humans. Clin Exp Immunol. 2016;183(2):157–65. doi:https://doi.org/10.1111/cei.12729. [Google Scholar] [PubMed] [CrossRef]
3. Maisonneuve P, Agodoa L, Gellert R, Stewart JH, Buccianti G, Lowenfels AB, et al. Distribution of primary renal diseases leading to end-stage renal failure in the United States, Europe, and Australia/New Zealand: results from an international comparative study. Am J Kidney Dis. 2000;35(1):157–65. doi:https://doi.org/10.1016/S0272-6386(00)70316-7. [Google Scholar] [PubMed] [CrossRef]
4. Gupta S, Pepper RJ, Ashman N, Walsh SB. Nephrotic syndrome: oedema formation and its treatment with diuretics. Front Physiol. 2019;9:1868. doi:https://doi.org/10.3389/fphys.2018.01868. [Google Scholar] [PubMed] [CrossRef]
5. Vaziri ND. Disorders of lipid metabolism in nephrotic syndrome: mechanisms and consequences. Kidney Int. 2016;90(1):41–52. doi:https://doi.org/10.1016/j.kint.2016.02.026. [Google Scholar] [PubMed] [CrossRef]
6. Couser WG. Primary membranous nephropathy. Clin J Am Soc Nephrol. 2017;12(6):983–97. doi:https://doi.org/10.2215/CJN.11761116. [Google Scholar] [PubMed] [CrossRef]
7. Tesar V, Hruskova Z. Autoantibodies in the diagnosis, monitoring, and treatment of membranous nephropathy. Front Immunol. 2021;12:593288. doi:https://doi.org/10.3389/fimmu.2021.593288. [Google Scholar] [PubMed] [CrossRef]
8. Daina E, Cortinovis M, Remuzzi G. Kidney diseases. Immunol Rev. 2023;313(1):239–61. doi:https://doi.org/10.1111/imr.13167. [Google Scholar] [PubMed] [CrossRef]
9. Hua MR, Zhao YL, Yang JZ, Zou L, Zhao YY, Li X. Membranous nephropathy: mechanistic insights and therapeutic perspectives. Int Immunopharmacol. 2023;120:110317. doi:https://doi.org/10.1016/j.intimp.2023.110317. [Google Scholar] [PubMed] [CrossRef]
10. Ponticelli C, Altieri P, Scolari F, Passerini P, Roccatello D, Cesana B, et al. A randomized study comparing methylprednisolone plus chlorambucil versus methylprednisolone plus cyclophosphamide in idiopathic membranous nephropathy. J Am Soc Nephrol. 1998;9(3):444–50. doi:https://doi.org/10.1681/ASN.V93444. [Google Scholar] [PubMed] [CrossRef]
11. Cattran DC, Appel GB, Hebert LA, Hunsicker LG, Pohl MA, Hoy WE, et al. A randomized trial of cyclosporine in patients with steroid-resistant focal segmental glomerulosclerosis. Kidney Int. 1999;56(6):2220–6. doi:https://doi.org/10.1046/j.1523-1755.1999.00778.x. [Google Scholar] [PubMed] [CrossRef]
12. Cattran DC, Appel GB, Hebert LA, Hunsicker LG, Pohl MA, Hoy WE, et al. Cyclosporine in patients with steroid-resistant membranous nephropathy: a randomized trial. Kidney Int. 2001;59(4):1484–90. doi:https://doi.org/10.1046/j.1523-1755.2001.0590041484.x. [Google Scholar] [PubMed] [CrossRef]
13. Fervenza FC, Appel GB, Barbour SJ, Rovin BH, Lafayette RA, Aslam N, et al. Rituximab or cyclosporine in the treatment of membranous nephropathy. N Engl J Med. 2019;381(1):36–46. doi:https://doi.org/10.1056/NEJMoa1814427. [Google Scholar] [PubMed] [CrossRef]
14. McQuarrie EP, Stirling CM, Geddes CC. Idiopathic membranous nephropathy and nephrotic syndrome: outcome in the era of evidence-based therapy. Nephrol Dial Transplant. 2012;27(1):235–42. doi:https://doi.org/10.1093/ndt/gfr220. [Google Scholar] [PubMed] [CrossRef]
15. Kalluri R, LeBleu VS. The biology, function, and biomedical applications of exosomes. Science. 2020;367(6478):eaau6977. doi:https://doi.org/10.1126/science.aau6977. [Google Scholar] [PubMed] [CrossRef]
16. Zhang Y, Liu Y, Liu H, Tang WH. Exosomes: biogenesis, biologic function and clinical potential. Cell Biosci. 2019;9(1):1–18. doi:https://doi.org/10.1186/s13578-019-0282-2. [Google Scholar] [PubMed] [CrossRef]
17. Hessvik NP, Llorente A. Current knowledge on exosome biogenesis and release. Cell Mol Life Sci. 2018;75:193–208. doi:https://doi.org/10.1007/s00018-017-2595-9. [Google Scholar] [PubMed] [CrossRef]
18. Grange C, Bussolati B. Extracellular vesicles in kidney disease. Nat Rev Nephrol. 2022;18(8):499–513. doi:https://doi.org/10.1038/s41581-022-00586-9. [Google Scholar] [PubMed] [CrossRef]
19. Erdbrügger U, Le TH. Extracellular vesicles in renal diseases: more than novel biomarkers? J Am Soc Nephrol. 2016;27(1):12–26. doi:https://doi.org/10.1681/ASN.2015010074. [Google Scholar] [PubMed] [CrossRef]
20. Ghasempour E, Hesami S, Movahed E, Keshel SH, Doroudian M. Mesenchymal stem cell-derived exosomes as a new therapeutic strategy in the brain tumors. Stem Cell Res Ther. 2022;13(1):527. doi:https://doi.org/10.1186/s13287-022-03212-4. [Google Scholar] [PubMed] [CrossRef]
21. Bonucci E. Fine structure of early cartilage calcification. J Ultrastruct Res. 1967;20(1–2):33–50. doi:https://doi.org/10.1016/S0022-5320(67)80034-0. [Google Scholar] [PubMed] [CrossRef]
22. Anderson HC. Vesicles associated with calcification in the matrix of epiphyseal cartilage. J Cell Biol. 1969;41(1):59–72. doi:https://doi.org/10.1083/jcb.41.1.59. [Google Scholar] [PubMed] [CrossRef]
23. Trams EG, Lauter CJ, Salem JN, Heine U. Exfoliation of membrane ecto-enzymes in the form of micro-vesicles. Biochim Biophys Acta. 1981;645(1):63–70. doi:https://doi.org/10.1016/0005-2736(81)90512-5. [Google Scholar] [PubMed] [CrossRef]
24. Pan BT, Johnstone RM. Fate of the transferrin receptor during maturation of sheep reticulocytes in vitro: selective externalization of the receptor. Cell. 1983;33(3):967–78. doi:https://doi.org/10.1016/0092-8674(83)90040-5. [Google Scholar] [PubMed] [CrossRef]
25. Gurung S, Perocheau D, Touramanidou L, Baruteau J. The exosome journey: from biogenesis to uptake and intracellular signalling. Cell Commun Signal. 2021;19(1):1–19. doi:https://doi.org/10.1186/s12964-021-00730-1. [Google Scholar] [PubMed] [CrossRef]
26. Jeppesen DK, Zhang Q, Franklin JL, Coffey RJ. Extracellular vesicles and nanoparticles: emerging complexities. Trends Cell Biol. 2023;33(8):667–81. doi:https://doi.org/10.1016/j.tcb.2023.01.002. [Google Scholar] [PubMed] [CrossRef]
27. Migliano SM, Teis D. ESCRT and membrane protein ubiquitination. Prog Mol Subcell Biol. 2018;57:107–35. doi:https://doi.org/10.1007/978-3-319-96704-2_4. [Google Scholar] [PubMed] [CrossRef]
28. Colombo M, Moita C, van Niel G, Kowal J, Vigneron J, Benaroch P, et al. Analysis of ESCRT functions in exosome biogenesis, composition and secretion highlights the heterogeneity of extracellular vesicles. J Cell Sci. 2013;126(24):5553–65. doi:https://doi.org/10.1242/jcs.128868. [Google Scholar] [PubMed] [CrossRef]
29. Ostrowski M, Carmo NB, Krumeich S, Fanget I, Raposo G, Savina A, et al. Rab27a and Rab27b control different steps of the exosome secretion pathway. Nat Cell Biol. 2010;12(1):19–30. doi:https://doi.org/10.1038/ncb2000. [Google Scholar] [PubMed] [CrossRef]
30. Mathieu M, Martin-Jaular L, Lavieu G, Théry C. Specificities of secretion and uptake of exosomes and other extracellular vesicles for cell-to-cell communication. Nat Cell Biol. 2019;21(1):9–17. doi:https://doi.org/10.1038/s41556-018-0250-9. [Google Scholar] [PubMed] [CrossRef]
31. Salomon C, Das S, Erdbrügger U, Kalluri R, Kiang Lim S, Olefsky JM, et al. Extracellular vesicles and their emerging roles as cellular messengers in endocrinology: an endocrine society scientific statement. Endocr Rev. 2022;43(3):441–68. doi:https://doi.org/10.1210/endrev/bnac009. [Google Scholar] [PubMed] [CrossRef]
32. Cocucci E, Meldolesi J. Ectosomes and exosomes: shedding the confusion between extracellular vesicles. Trends Cell Biol. 2015;25(6):364–72. doi:https://doi.org/10.1016/j.tcb.2015.01.004. [Google Scholar] [PubMed] [CrossRef]
33. Colombo M, Raposo G, Théry C. Biogenesis, secretion, and intercellular interactions of exosomes and other extracellular vesicles. Annu Rev Cell Dev Biol. 2014;30:255–89. doi:https://doi.org/10.1146/annurev-cellbio-101512-122326. [Google Scholar] [PubMed] [CrossRef]
34. Yuan L, Li JY. Exosomes in Parkinson’s disease: current perspectives and future challenges. ACS Chem Neurosci. 2019;10(2):964–72. doi:https://doi.org/10.1021/acschemneuro.8b00469. [Google Scholar] [PubMed] [CrossRef]
35. Castaño C, Kalko S, Novials A, Párrizas M. Obesity-associated exosomal miRNAs modulate glucose and lipid metabolism in mice. Proc Natl Acad Sci U S A. 2018;115(48):12158–63. doi:https://doi.org/10.1073/pnas.1808855115. [Google Scholar] [PubMed] [CrossRef]
36. Wu H, Fu M, Liu J, Chong W, Fang Z, Du F, et al. The role and application of small extracellular vesicles in gastric cancer. Mol Cancer. 2021;20(1):71. doi:https://doi.org/10.1186/s12943-021-01365-z. [Google Scholar] [PubMed] [CrossRef]
37. Liang Y, Duan L, Lu J, Xia J. Engineering exosomes for targeted drug delivery. Theranostics. 2021;11(7):3183–95. doi:https://doi.org/10.7150/thno.52570. [Google Scholar] [PubMed] [CrossRef]
38. Zhang M, Hu S, Liu L, Dang P, Liu Y, Sun Z, et al. Engineered exosomes from different sources for cancer-targeted therapy. Signal Transduct Target Ther. 2023;8(1):124. doi:https://doi.org/10.1038/s41392-023-01382-y. [Google Scholar] [PubMed] [CrossRef]
39. Masaoutis C, Al Besher S, Koutroulis I, Theocharis S. Exosomes in nephropathies: a rich source of novel biomarkers. Dis Markers. 2020;2020:1–12. doi:https://doi.org/10.1155/2020/8897833. [Google Scholar] [PubMed] [CrossRef]
40. Yang D, Zhang W, Zhang H, Zhang F, Chen L, Ma L, et al. Progress, opportunity, and perspective on exosome isolation-efforts for efficient exosome-based theranostics. Theranostics. 2020;10(8):3684–707. doi:https://doi.org/10.7150/thno.41580. [Google Scholar] [PubMed] [CrossRef]
41. Glassock RJ. Human idiopathic membranous nephropathy—a mystery solved? N Engl J Med. 2009;361(1):81–3. doi:https://doi.org/10.1056/NEJMe0903343. [Google Scholar] [PubMed] [CrossRef]
42. BeckJr LH, Bonegio RG, Lambeau G, Beck DM, Powell DW, Cummins TD, et al. M-type phospholipase A2 receptor as target antigen in idiopathic membranous nephropathy. N Engl J Med. 2009;361(1):11–21. doi:https://doi.org/10.1056/NEJMoa0810457. [Google Scholar] [PubMed] [CrossRef]
43. Tomas NM, BeckJr LH, Meyer-Schwesinger C, Seitz-Polski B, Ma H, Zahner G, et al. Thrombospondin type-1 domain-containing 7A in idiopathic membranous nephropathy. N Engl J Med. 2014;371(24):2277–87. doi:https://doi.org/10.1056/NEJMoa1409354. [Google Scholar] [PubMed] [CrossRef]
44. Ronco P, Debiec H. Pathophysiological advances in membranous nephropathy: time for a shift in patient’s care. Lancet. 2015;385(9981):1983–92. doi:https://doi.org/10.1016/S0140-6736(15)60731-0. [Google Scholar] [PubMed] [CrossRef]
45. Debiec H, Valayannopoulos V, Boyer O, Nöel LH, Callard P, Sarda H, et al. Allo-immune membranous nephropathy and recombinant aryl sulfatase replacement therapy: a need for tolerance induction therapy. J Am Soc Nephrol. 2014;25(4):675–80. doi:https://doi.org/10.1681/ASN.2013030290. [Google Scholar] [PubMed] [CrossRef]
46. Debiec H, Lefeu F, Kemper MJ, Niaudet P, Deschênes G, Remuzzi G, et al. Early-childhood membranous nephropathy due to cationic bovine serum albumin. N Engl J Med. 2011;364(22):2101–10. doi:https://doi.org/10.1056/NEJMoa1013792. [Google Scholar] [PubMed] [CrossRef]
47. Burbelo PD, Joshi M, Chaturvedi A, Little DJ, Thurlow JS, Waldman M, et al. Detection of PLA2R autoantibodies before the diagnosis of membranous nephropathy. J Am Soc Nephrol. 2020;31(1):208–17. doi:https://doi.org/10.1681/ASN.2019050538. [Google Scholar] [PubMed] [CrossRef]
48. Hoxha E, Reinhard L, Stahl RA. Membranous nephropathy: new pathogenic mechanisms and their clinical implications. Nat Rev Nephrol. 2022;18(7):466–78. doi:https://doi.org/10.1038/s41581-022-00564-1. [Google Scholar] [PubMed] [CrossRef]
49. Müller-Deile J, Sopel N, Ohs A, Rose V, Gröner M, Wrede C, et al. Glomerular endothelial cell-derived microRNA-192 regulates nephronectin expression in idiopathic membranous glomerulonephritis. J Am Soc Nephrol. 2021;32(11):2777–94. doi:https://doi.org/10.1681/ASN.2020121699. [Google Scholar] [PubMed] [CrossRef]
50. Chaput N, Théry C. Exosomes: immune properties and potential clinical implementations. Semin Immunopathol. 2011;33(5):419–40. doi:https://doi.org/10.1007/s00281-010-0233-9. [Google Scholar] [PubMed] [CrossRef]
51. Zhao Q, Dai H, Liu X, Jiang H, Liu W, Feng Z, et al. Helper T cells in idiopathic membranous nephropathy. Front Immunol. 2021;12:665629. doi:https://doi.org/10.3389/fimmu.2021.665629. [Google Scholar] [PubMed] [CrossRef]
52. Roccatello D, Sciascia S, Di Simone D, Solfietti L, Naretto C, Fenoglio R, et al. New insights into immune mechanisms underlying response to Rituximab in patients with membranous nephropathy: a prospective study and a review of the literature. Autoimmun Rev. 2016;15(6):529–38. doi:https://doi.org/10.1016/j.autrev.2016.02.014. [Google Scholar] [PubMed] [CrossRef]
53. Motavalli R, Etemadi J, Soltani-Zangbar MS, Ardalan MR, Kahroba H, Roshangar L, et al. Altered Th17/Treg ratio as a possible mechanism in pathogenesis of idiopathic membranous nephropathy. Cytokine. 2021;141:155452. doi:https://doi.org/10.1016/j.cyto.2021.155452. [Google Scholar] [PubMed] [CrossRef]
54. Cantarelli C, Jarque M, Angeletti A, Manrique J, Hartzell S, O’Donnell T, et al. A comprehensive phenotypic and functional immune analysis unravels circulating anti-phospholipase A2 receptor antibody secreting cells in membranous nephropathy patients. Kidney Int Rep. 2020;5(10):1764–76. doi:https://doi.org/10.1016/j.ekir.2020.07.028. [Google Scholar] [PubMed] [CrossRef]
55. Shi X, Qu Z, Zhang L, Zhang N, Liu Y, Li M, et al. Increased ratio of ICOS+/PD-1+ follicular helper T cells positively correlates with the development of human idiopathic membranous nephropathy. Clin Exp Pharmacol Physiol. 2016;43(4):410–6. doi:https://doi.org/10.1111/1440-1681.12555. [Google Scholar] [PubMed] [CrossRef]
56. Zhang Z, Shi Y, Yang K, Crew R, Wang H, Jiang Y. Higher frequencies of circulating ICOS+, IL-21+ T follicular helper cells and plasma cells in patients with new-onset membranous nephropathy. Autoimmunity. 2017;50(8):458–67. doi:https://doi.org/10.1080/08916934.2017.1385775. [Google Scholar] [PubMed] [CrossRef]
57. Théry C, Duban L, Segura E, Véron P, Lantz O, Amigorena S. Indirect activation of naïve CD4+ T cells by dendritic cell-derived exosomes. Nat Immunol. 2002;3(12):1156–62. doi:https://doi.org/10.1038/ni854. [Google Scholar] [PubMed] [CrossRef]
58. Whiteside TL. Immune modulation of T-cell and NK (natural killer) cell activities by TEXs (tumour-derived exosomes). Biochem Soc Trans. 2013;41(1):245–51. doi:https://doi.org/10.1042/BST20120265. [Google Scholar] [PubMed] [CrossRef]
59. Cui X, Liu Y, Wang S, Zhao N, Qin J, Li Y, et al. Circulating exosomes activate dendritic cells and induce unbalanced CD4+ T cell differentiation in hashimoto thyroiditis. J Clin Endocrinol Metab. 2019;104(10):4607–18. doi:https://doi.org/10.1210/jc.2019-00273. [Google Scholar] [PubMed] [CrossRef]
60. Reinhard L, Stahl RA, Hoxha E. Is primary membranous nephropathy a complement mediated disease? Mol Immunol. 2020;128:195–204. doi:https://doi.org/10.1016/j.molimm.2020.10.017. [Google Scholar] [PubMed] [CrossRef]
61. Salant D, Belok S, Stilmant M, Darby C, Couser WG. Determinants of glomerular localization of subepithelial immune deposits: effects of altered antigen to antibody ratio, steroids, vasoactive amine antagonists, and aminonucleoside of puromycin on passive Heymann nephritis in rats. Lab Invest. 1979;41(1):89–99. [Google Scholar] [PubMed]
62. Salant DJ, Darby C, Couser WG. Experimental membranous glomerulonephritis in rats: quantitative studies of glomerular immune deposit formation in isolated glomeruli and whole animals. J Clin Invest. 1980;66(1):71–81. doi:https://doi.org/10.1172/JCI109837. [Google Scholar] [PubMed] [CrossRef]
63. Huang C, Fisher KP, Hammer SS, Navitskaya S, Blanchard GJ, Busik JV. Plasma exosomes contribute to microvascular damage in diabetic retinopathy by activating the classical complement pathway. Diabetes. 2018;67(8):1639–49. doi:https://doi.org/10.2337/db17-1587. [Google Scholar] [PubMed] [CrossRef]
64. Whitehead B, Wu L, Hvam ML, Aslan H, Dong M, Dyrskjøt L, et al. Tumour exosomes display differential mechanical and complement activation properties dependent on malignant state: implications in endothelial leakiness. J Extracell Vesicles. 2015;4(1):29685. doi:https://doi.org/10.3402/jev.v4.29685. [Google Scholar] [PubMed] [CrossRef]
65. Sadallah S, Eken C, Martin PJ, Schifferli JA. Microparticles (ectosomes) shed by stored human platelets downregulate macrophages and modify the development of dendritic cells. J Immunol. 2011;186(11):6543–52. doi:https://doi.org/10.4049/jimmunol.1002788. [Google Scholar] [PubMed] [CrossRef]
66. Ståhl Al, Vaziri-Sani F, Heinen S, Kristoffersson AC, Gydell KH, Raafat R, et al. Factor H dysfunction in patients with atypical hemolytic uremic syndrome contributes to complement deposition on platelets and their activation. Blood. 2008;111(11):5307–15. doi:https://doi.org/10.1182/blood-2007-08-106153. [Google Scholar] [PubMed] [CrossRef]
67. Yin W, Ghebrehiwet B, Peerschke EI. Expression of complement components and inhibitors on platelet microparticles. Platelets. 2008;19(3):225–33. doi:https://doi.org/10.1080/09537100701777311. [Google Scholar] [PubMed] [CrossRef]
68. Rabesandratana H, Toutant JP, Reggio H, Vidal M. Decay-accelerating factor (CD55) and membrane inhibitor of reactive lysis (CD59) are released within exosomes during in vitro maturation of reticulocytes. Blood. 1998;91(7):2573–80. doi:https://doi.org/10.1182/blood.V91.7.2573. [Google Scholar] [CrossRef]
69. De Vriese AS, Glassock RJ, Nath KA, Sethi S, Fervenza FC. A proposal for a serology-based approach to membranous nephropathy. J Am Soc Nephrol. 2017;28(2):421–30. doi:https://doi.org/10.1681/ASN.2016070776. [Google Scholar] [PubMed] [CrossRef]
70. van de Logt AE, Justino J, Vink CH, van den Brand J, Debiec H, Lambeau G, et al. Anti-PLA2R1 antibodies as prognostic biomarker in membranous nephropathy. Kidney Int Rep. 2021;6(6):1677–86. doi:https://doi.org/10.1016/j.ekir.2021.04.002. [Google Scholar] [PubMed] [CrossRef]
71. Gödel M, Grahammer F, Huber TB. Thrombospondin type-1 domain-containing 7A in idiopathic membranous nephropathy. N Engl J Med. 2015;372(11):1073–5. doi:https://doi.org/10.1056/NEJMc1500130. [Google Scholar] [CrossRef]
72. Bobart SA, de Vriese AS, Pawar AS, Zand L, Sethi S, Giesen C, et al. Noninvasive diagnosis of primary membranous nephropathy using phospholipase A2 receptor antibodies. Kidney Int. 2019;95(2):429–38. doi:https://doi.org/10.1016/j.kint.2018.10.021. [Google Scholar] [PubMed] [CrossRef]
73. Du Y, Li J, He F, Lv Y, Liu W, Wu P, et al. The diagnosis accuracy of PLA2R-AB in the diagnosis of idiopathic membranous nephropathy: a meta-analysis. PLoS One. 2014;9(8):e104936. doi:https://doi.org/10.1371/journal.pone.0104936. [Google Scholar] [PubMed] [CrossRef]
74. Debiec H, Ronco P. PLA2R autoantibodies and PLA2R glomerular deposits in membranous nephropathy. N Engl J Med. 2011;364(7):689–90. doi:https://doi.org/10.1056/NEJMc1011678. [Google Scholar] [PubMed] [CrossRef]
75. van de Logt AE, Fresquet M, Wetzels JF, Brenchley P. The anti-PLA2R antibody in membranous nephropathy: what we know and what remains a decade after its discovery. Kidney Int. 2019;96(6):1292–302. doi:https://doi.org/10.1016/j.kint.2019.07.014. [Google Scholar] [PubMed] [CrossRef]
76. Lv LL, Cao Y, Liu D, Xu M, Liu H, Tang RN, et al. Isolation and quantification of microRNAs from urinary exosomes/microvesicles for biomarker discovery. Int J Biol Sci. 2013;9(10):1021–31. doi:https://doi.org/10.7150/ijbs.6100. [Google Scholar] [PubMed] [CrossRef]
77. Kalluri R. The biology and function of exosomes in cancer. J Clin Invest. 2016;126(4):1208–15. doi:https://doi.org/10.1172/JCI81135. [Google Scholar] [PubMed] [CrossRef]
78. Lorenzen JM, Thum T. Circulating and urinary microRNAs in kidney disease. Clin J Am Soc Nephrol. 2012;7(9):1528–33. doi:https://doi.org/10.2215/CJN.01170212. [Google Scholar] [PubMed] [CrossRef]
79. Cheng L, Sun X, Scicluna BJ, Coleman BM, Hill AF. Characterization and deep sequencing analysis of exosomal and non-exosomal miRNA in human urine. Kidney Int. 2014;86(2):433–44. doi:https://doi.org/10.1038/ki.2013.502. [Google Scholar] [PubMed] [CrossRef]
80. Rood IM, Merchant ML, Wilkey DW, Zhang T, Zabrouskov V, van der Vlag J, et al. Increased expression of lysosome membrane protein 2 in glomeruli of patients with idiopathic membranous nephropathy. Proteomics. 2015;15(21):3722–30. doi:https://doi.org/10.1002/pmic.201500127. [Google Scholar] [PubMed] [CrossRef]
81. Guo S, Hao H, Li S, Zhang L, Li R. Differential expression of urinary exosomal miRNA in idiopathic membranous nephropathy and evaluation of its diagnostic value. Tohoku J Exp Med. 2022;256(4):327–36. doi:https://doi.org/10.1620/tjem.2022.J002. [Google Scholar] [PubMed] [CrossRef]
82. Zhang J, Zhu Y, Cai R, Jin J, He Q. Differential expression of urinary exosomal small RNAs in idiopathic membranous nephropathy. Biomed Res Int. 2020;2020:3170927. doi:https://doi.org/10.1155/2020/3170927. [Google Scholar] [PubMed] [CrossRef]
83. Ma H, Xu Y, Zhang R, Guo B, Zhang S, Zhang X. Differential expression study of circular RNAs in exosomes from serum and urine in patients with idiopathic membranous nephropathy. Arch Med Sci. 2019;15(3):738–53. doi:https://doi.org/10.5114/aoms.2019.84690. [Google Scholar] [PubMed] [CrossRef]
84. Li Q, Xu M, Zhang Z, Yin M, Zhang Y, Liu F. Urinary exosomal hsa_circ_0001250 as a novel diagnostic biomarker of idiopathic membranous nephropathy. J Transl Med. 2022;20(1):607. doi:https://doi.org/10.1186/s12967-022-03784-y. [Google Scholar] [PubMed] [CrossRef]
85. Hanjani NA, Esmaelizad N, Zanganeh S, Gharavi AT, Heidarizadeh P, Radfar M, et al. Emerging role of exosomes as biomarkers in cancer treatment and diagnosis. Crit Rev Oncol Hematol. 2022;169:103565. doi:https://doi.org/10.1016/j.critrevonc.2021.103565. [Google Scholar] [PubMed] [CrossRef]
86. Li XQ, Lerman LO, Meng Y. Potential role of extracellular vesicles in the pathophysiology of glomerular diseases. Clin Sci. 2020;134(20):2741–54. doi:https://doi.org/10.1042/CS20200766. [Google Scholar] [PubMed] [CrossRef]
87. Matsuzaka Y, Yashiro R. Advances in purification, modification, and application of extracellular vesicles for novel clinical treatments. Membranes. 2022;12(12):1244. doi:https://doi.org/10.3390/membranes12121244. [Google Scholar] [PubMed] [CrossRef]
Cite This Article
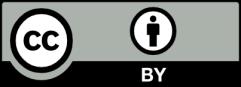