Open Access
ARTICLE
Transcriptomic Responses of Garlic (Allium sativum L.) to Heat and Drought Stresses
Department of Industrial Plant Science and Technology, Chungbuk National University, Cheongju, 28644, Korea
* Corresponding Author: Tae Kyung Hyun. Email:
(This article belongs to the Special Issue: Abiotic and Biotic Stress Tolerance in Crop)
Phyton-International Journal of Experimental Botany 2023, 92(11), 3077-3090. https://doi.org/10.32604/phyton.2023.044032
Received 19 July 2023; Accepted 25 August 2023; Issue published 24 October 2023
Abstract
Heat and drought are prominent abiotic stressors that limit crop productivity and yield, particularly concerning climate change; therefore, understanding the molecular mechanisms underlying plant stress responses is crucial for stress-tolerant crop production. This study conducted a transcriptomic analysis to elucidate how garlic (Allium sativum L.) responds to drought and heat stress conditions. Transcriptome libraries were generated to identify differentially expressed genes (DEGs) induced by drought and heat stresses. Functional classification and clustering analysis of DEGs revealed stress-specific gene expression patterns. Notably, cell wall-related genes were implicated in the drought response, whereas heat stress was associated with heat stress transcription factors and heat shock proteins. Our results provide essential information for future studies on stress tolerances in garlic.Keywords
Heat stress, defined as soil and air temperatures elevated beyond a critical threshold, and drought, characterized by inadequate water availability due to minimal or no rainfall, are influential factors that reduce crop productivity and yield, consequently decreasing farmers’ income [1]. In addition, drought and heat stresses have surged in recent years due to global warming [2]. Under these stress conditions, plants exhibit diverse physiological and biochemical responses, including stomatal movement, cell growth, development, and photosynthesis inhibition, as well as modifications in biosynthetic pathways, and the respiration pathway [3–6]. This indicates that stress tolerance is achieved through a complex interplay of molecular, physiological, and biochemical processes that induce or repress gene regulations through intricate transcriptional networks [7]. Therefore, focusing on responsive genes and transcriptional networks induced by drought and heat stresses is crucial for effectively safeguarding crops.
Garlic (Allium sativum L.) is a valuable market crop, notably consumed as a nutritious green vegetable, spice, and medicinal herb [8]. However, since garlic is sensitive to water deficit conditions [9], achieving a maximum garlic bulb yield is predicated upon effective irrigation management [10]. In addition, heat stress decreases the rate and speed of garlic germination, effectuating depleted growth and yield [11]. Morphological and physiological alterations in garlic from various stress conditions have been extensively characterized. Yet, knowledge regarding genome-wide responses in garlic to drought or heat stress is limited, even though studying transcriptome changes aids in comprehending the molecular mechanisms behind a plant adaptation to environmental stresses.
This study aims to explore the molecular mechanisms underlying the response of garlic to environmental stress. We employed a transcriptomic approach to analyze the expression patterns of key genes under conditions of drought and heat stress. Through this analysis, we identified genes that are induced or repressed by drought and heat stress, classifying them as common or specifically regulated. Our findings shed light on the molecular mechanisms involved in the tolerance of garlic to environmental stress, offering valuable insights into the fundamental understanding of stress tolerance mechanisms.
2.1 Plant Materials and Stress Treatments
Seeds of garlic cloves (A. sativum L. cv. Daeseo) were grown in a growth chamber with a photoperiod of 16 h of light and 8 h of darkness at a temperature of 24°C. Based on our previous study, we observed physiological changes of garlic plants after exposure to heat stress at 45°C for 8 h [12]. To induce heat stress, 4-week-old plants were exposed to a temperature of 45°C for a duration of 8 h. A moderate drought stress does not induce significant morphophysiological changes, whereas severe water deficit notably triggered physiological and biochemical responses in various plant species [13,14]. Therefore, for drought stress, plants were subjected to a withholding of water for two weeks, resulting in the induction of severe drought stress. Each treatment was replicated three times, providing a total of three biological replicates.
2.2 H2O2 Accumulation, Malondialdehyde (MDA) Content, Relative Water Content (RWC), and Photosynthesis Determination
The accumulation of H2O2 in leaves subjected to drought or heat stress was detected using an endogenous peroxidase-dependent in situ histochemical staining method, following the protocol described by Ji et al. [12].
Lipid peroxidation induced by drought or heat stress was assessed by measuring the content of malondialdehyde (MDA), according to the procedure outlined by Eom et al. [6].
The relative water content (RWC) was determined before and after subjecting the plants to drought or heat stress, following the method described by Eom et al. [13].
The impact of drought or heat stress on photosynthesis was evaluated using chlorophyll fluorescence analysis, specifically employing the FluorPen FP110 (Photon Systems Instruments, Drásov, Czech Republic).
2.3 Transcriptome and Differentially Expressed Genes (DEGs) Analyses
To generate cDNA libraries, an equal amount of total RNA from three independent replicates was pooled. The cDNA libraries were synthesized, and paired-end sequencing was performed using the Illumina HiSeq™ 2500 platform. The obtained sequencing reads were processed following a previously established protocol [13]. The reads were then aligned to the assembled garlic sequence available at (https://doi.org/10.6084/m9.figshare.12570947.v1) [15] using the HISAT2 aligner [16]. The sequencing results have been deposited at the National Agricultural Biotechnology Information Center (NABIC, http://nabic.rda.go.kr).
Transcript levels were computed employing SAMtools (https://sourceforge.net/projects/samtools/files/samtools/), and the comparative transcript abundances were assessed through DEseq. The transcript level of each gene was quantified as fragments per kilobase of transcript sequence per million base pairs (FPKM). Differentially expressed genes (DEGs) were identified by applying a p-value threshold of 0.05 and adjusting for |log2(fold change)| of at least 1 [17]. For functional classification of the identified DEGs, Blast2GO (https://www.blast2go.com/) and KEGG (https://www.genome.jp/kegg/) were employed.
To validate the transcription patterns observed in the RNA-Seq data, qRT-PCR analysis was performed on heat stress transcription factors (Hsfs). The primer pairs used for qRT-PCR are provided in Table S1.
The results of all experiments conducted in this study were presented as mean values accompanied by the standard error (SE), derived from three independent experimental replicates. To determine significant differences among the data, Duncan’s multiple range test (p < 0.05) was applied.
3.1 Physiological Changes in Response to Drought or Heat Stress
Physiological responses of plants to environmental stresses encompass various symptoms such as leaf wilting, senescence, leaf necrosis, and decreased water loss through transpiration [18]. Drought stress reduces turgor pressure, while heat stress enhances evapotranspiration in plants. Therefore, leaf wilting is a prevalent phenotype in plants subjected to drought and heat stress. As shown in Fig. 1A, leaf wilting was apparent when garlic plants were exposed to drought or heat stresses. In addition, RWC levels were attenuated by 25.8% from drought and 20.6% from heat stress compared to control plants (normal growth) (Fig. 1C). When plants respond to drought and heat stresses, chloroplasts overproduce reactive oxygen species, resulting in the induction of oxidative damage to DNA, proteins, and lipids in different cellular compartments and inhibiting photosynthesis [3,19,20]. Similarly, H2O2 (Fig. 1B) and MDA (lipid peroxidation, Fig. 1D) accumulation were observed in drought- and heat-treated garlic plants. Furthermore, these treatments caused a decrease in the potential activity of PSII (Fv/Fo) and the maximum quantum efficiency of PSII photochemistry (Fv/Fm) (Fig. 1E). These physiological changes substantiate the efficacy of our stress treatments, and we proceeded to harvest the leaves of plants exposed to drought and heat stresses for further analyses.
Figure 1: Physiological responses of garlic plants to drought and heat stress. (A) Visual phenotypes of garlic plants following exposure to drought stress (water withholding for 2 weeks) and heat stress (45°C for 8 h). The changes in levels of H2O2 (B), relative water content (C), MDA (D), and photosynthesis (E) were measured after the drought or heat treatments. The means (±SE) with distinct letters (p < 0.05, Duncan’s multiple range test) indicate significant differences between groups
3.2 Determination of the Transcriptomic Alterations in Response to Drought and Heat Stresses
Transcriptome sequencing has proven to be a highly effective approach for investigating the global transcription network in response to various stresses in different crops, such as Kimchi Cabbage [6,13], rapeseed [21], wheat [22], chickpea [23], and cucumber [24]. In this study, we employed transcriptome sequencing to uncover the molecular mechanisms underlying the stress response in garlic. RNA libraries were generated from the leaves of control (CT), drought-stressed (DT), and heat-stressed (HT) garlic plants. These libraries have been deposited in the NABIC (Table 1). After filtering low-quality reads, we obtained 42–46 million high-quality reads (6.21 to 6.85 Gb) from the three libraries, with over 85% of the clean reads successfully mapped to the reference genome (Table 1). Two pair-wise comparisons (CT vs. DT and CT vs. HT) were conducted to identify differentially expressed genes (DEGs) induced by the stress treatments.
According to the comparison between CT and DT, a total of 1,099 genes (376 drought-induced and 723 drought-reduced) were identified as DEGs. In addition, we determined 791 heat-induced and 285 heat-reduced genes, as indicated on a Volcano plot (Fig. 2A). Using hierarchical clustering expression patterns of all 2,031 DEGs, we distinguished six clusters (C1 to C6) (Fig. 2B) and analyzed the gene ontology (GO) terms. C3 included 337 up-regulated genes from drought stress with “membrane,” “cellular metabolic process,” and “hydrolase activity” as top GO terms, whereas C4 encompassed 776 up-regulated genes from heat stress with “heterocyclic compound binding,” “primary metabolic process,” and “intracellular organelle” GO terms (Fig. 2C). Furthermore, genes classified as “response to stress” were up-regulated by both stresses. In contrast, genes related to the “carboxylic acid biosynthetic process” and “carbohydrate metabolic process” were down-regulated by drought and heat stresses, respectively.
Figure 2: Drought or heat stress-induced differentially expressed genes (DEGs) in garlic leaves. Volcano (A) plot and hierarchical analysis (B) were conducted according to expression patterns of DEGs. The analyses of Gene ontology (GO) enrichment (C) and Kyoto Encyclopedia of Genes and Genomes (KEGG) pathway (D) were performed using DEGs. The color scale ranging from red to blue represents the raw Z-score
The Kyoto Encyclopedia of Genes and Genomes (KEGG) pathway database serves as a knowledge hub, facilitating the methodical analysis of gene functions within the context of complex gene networks [25]. Therefore, to identify the biological pathways activated by heat stress or drought stress, DEGs underwent annotation through BLASTx alignments against the KEGG database with a significance threshold set at 10−5. As shown in Fig. 2D, the mapped DEGs corresponded to metabolic pathways involving significant biomolecules, such as starch, hormones, phenylpropanoids, etc. The pathways with most representation by DT-induced DEGs were “starch and sucrose metabolism (up-regulated DEGs),” and “MAPK signaling pathway-plant (down-regulated DEGs)”. SNF1-related protein kinase 2 (SnRK2), a member of the “MAPK signaling pathway–plant,” holds crucial roles in the plant response to abiotic stresses and nutrient limitations, functioning via pathways that are both ABA-dependent and independent [26]. In garlic plants, two SnRK2 genes (Asa5G00500 and Asa7G05049) were up-regulated by drought stresses, indicating that they might be interesting candidate genes for improving drought tolerance. In addition, “protein processing in endoplasmic reticulum” represented the largest group in HT (Fig. 2D). Proteins produced in the rough endoplasmic reticulum (ER) fold accurately aided by the ER lumen chaperone, a member of the heat shock protein (Hsp) [27]. This suggests that garlic plants have the capability to modify their metabolism in order to prevent harm by the production of major Hsps, as described below. A majority of proteins synthesized in the ER undergo glycosylation, which is essential for proper folding, protecting against digestive enzymes, and enabling signal conduction [28]. This indicates that HT-induced DEGs in “glycolysis/gluconegenesis” (Fig. 2D) might play a crucial role in maintaining the structural stability and functionality of protein molecules.
3.3 Unique Transcriptomic Changes under Each Stress Condition
Analyzing stress-specific genes and biological processes that shift in response to drought or heat stress will contribute to our understanding of the unique adaptation strategies relative to each stress. For this objective, the metabolic pathways of stress-induced DEGs were analyzed using MapMan. As shown in Fig. 3, cell wall-related genes involved in cell wall modification, pectin esterases, and cell wall degradation were enriched among drought-induced DEGs. Drought stress affects the extensibility of the cell wall, which is crucial in regulating cell growth and cell wall expansion [29]. This impact is observed through the suppression of cell wall-related genes in garlic plants (Fig. 3). Pectin esterases (pectin methylesterases; EC 3.1.1.11) are essential for cell wall elongation, pollen development, seed germination, seed dehiscence, root tip extension, fruit softening, and stress resistance [30]. Notably, overexpression of poplar pectin methylesterase in Arabidopsis inhibited stoma opening, resulting in lower water loss rate in detached leaves [31]. In addition, the transient silencing of pectin methylesterase inhibitor significantly improved tomato drought resistance [32]. To validate the gene expression results from RNA-seq, the expression levels of four pectin esterases were analyzed using qRT-PCR. As shown in Fig. S1, these genes were down-regulated by drought stress. These findings indicate that down-regulating pectin esterases in garlic may adversely impact its drought resistance. Consequently, analyzing the physiological functions of these proteins will facilitate to obtain a deeper understanding about drought tolerance in garlic.
Figure 3: MapMan visualization of differentially expressed genes (DEGs) associated with cell wall-related processes in each experimental comparison. The transcription levels in response to drought or heat stress are depicted using various color representations
Heat stress transcription factors (Hsfs) are vital for cellular responses to heat stress by controlling the transcriptional activity of multiple genes involved in diverse signaling and metabolic pathways [33]. Hsfs primarily promote the rapid synthesis and accumulation of heat shock proteins (Hsps), molecular chaperones that prevent protein aggregation and ensure protein homeostasis [33,34]. We identified 9 Hsfs and 89 Hsps in heat-specific up-regulated DEGs (Fig. 4A and Table S2). Under heat stress condition, Hsp70s interact with extended protein peptide segments as well as partially folded proteins, preventing aggregation, reshaping folding pathways, and regulating activity [35]. When exposed to high temperature treatment at 35°C–42°C, the HSP70s were up-regulated in various plants including cucumber, pepper, and tomato [36–38]. In addition, overexpression of herbaceous peony HSP70 in Arabidopsis improved the tolerance to heat stress [39]. In HT-induced DEGs, we found 15 HSP70 genes (Asa8G05234, Asa8G05236, Asa0G05775, Asa7G01686, Asa7G05922, Asa0G01584, Asa0G01583, Asa0G01585, Asa8G05256, Asa6G05836, Asa7G01677, Asa8G05235, Asa0G05725, Asa6G00770, and Asa0G05726; Table S2), suggesting that they could serve as promising candidate genes for enhancing heat tolerance in garlic plants. 9 Hsfs were subjected to qRT-PCR analysis to validate the RNA-seq data, confirming the reliability of our results (Fig. 4B). These findings indicate that these Hsf-Hsp networks likely impact garlic’s response to heat stress and contribute to its heat tolerance.
Figure 4: Heat-specific up-regulated heat stress transcription factors (Hsfs) and heat shock proteins (Hsps) (A). The expression patterns of the selected garlic Hsfs were determined using qRT-PCR (B). The color scale ranging from red to blue represents the raw Z-score. Significant differences between means (±SE) were denoted by different letters (p < 0.05, determined by Duncan’s multiple range test)
Understanding the molecular mechanisms in plant responses to environmental stress is crucial for developing climate-resilient crops. We successfully identified overlapping and stress-specific molecular responses in garlic plants through a comparative transcriptomic analysis from drought and heat stresses. We explored unique transcriptomic changes under each condition, focusing on cell wall-related genes regarding drought stress and Hsfs-Hsps for heat stress. Our findings provide a basis for future research aimed at further understanding the molecular mechanisms involved in the response to environmental stresses.
Acknowledgement: Not applicable.
Funding Statement: This work was carried out with the support of “Cooperative Research Program for Agriculture Science and Technology Development (Project No. PJ01501905)” Rural Development Administration, Korea.
Author Contributions: The authors confirm their contribution to the paper as follows: study conception and design: Tae Kyung Hyun; analysis and interpretation of results: Seung Hee Eom; draft manuscript preparation: Seung Hee Eom, Tae Kyung Hyun. All authors reviewed the results and approved the final version of the manuscript.
Availability of Data and Materials: All data generated or analyzed during this study are included in this published article (and its Supplementary Materials).
Ethics Approval: Not applicable.
Conflicts of Interest: The authors declare that they have no conflicts of interest to report regarding the present study.
References
1. Lamaoui, M., Jemo, M., Datla, R., Bekkaoui, F. (2018). Heat and drought stresses in crops and approaches for their mitigation. Frontiers in Chemistry, 6, 26. https://doi.org/10.3389/fchem.2018.00026 [Google Scholar] [PubMed] [CrossRef]
2. Zandalinas, S. I., Fritschi, F. B., Mittler, R. (2021). Global warming, climate change, and environmental pollution: Recipe for a multifactorial stress combination disaster. Trends in Plant Science, 26(6), 588–599. https://doi.org/10.1016/j.tplants.2021.02.011 [Google Scholar] [PubMed] [CrossRef]
3. Li, M., Kim, C. (2021). Chloroplast ROS and stress signaling. Plant Communications, 3(1), 100264. https://doi.org/10.1016/j.xplc.2021.100264 [Google Scholar] [PubMed] [CrossRef]
4. Yang, X., Lu, M., Wang, Y., Wang, Y., Liu, Z. et al. (2021). Response mechanism of plants to drought stress. Horticulturae, 7(3), 50. https://doi.org/10.3390/horticulturae7030050 [Google Scholar] [CrossRef]
5. Zhou, Y., Xu, F., Shao, Y., He, J. (2022). Regulatory mechanisms of heat stress response and thermomorphogenesis in plants. Plants, 11(24), 3410. https://doi.org/10.3390/plants11243410 [Google Scholar] [PubMed] [CrossRef]
6. Eom, S. H., Lim, H. B., Hyun, T. K. (2023). Overexpression of the Brassica rapa bZIP transcription factor, BrbZIP-S, increases the stress tolerance in Nicotiana benthamiana. Biology, 12(4), 517. https://doi.org/10.3390/biology12040517 [Google Scholar] [PubMed] [CrossRef]
7. Praveen, A., Dubey, S., Singh, S., Sharma, V. K. (2023). Abiotic stress tolerance in plants: A fascinating action of defense mechanisms. 3 Biotech, 13(3), 102. https://doi.org/10.1007/s13205-023-03519-w [Google Scholar] [PubMed] [CrossRef]
8. Dorrigiv, M., Zareiyan, A., Hosseinzadeh, H. (2020). Garlic (Allium sativum) as an antidote or a protective agent against natural or chemical toxicities: A comprehensive update review. Phytotherapy Research, 34(8), 1770–1797. https://doi.org/10.1002/ptr.6645 [Google Scholar] [PubMed] [CrossRef]
9. Habuš Jerčić, I., Bošnjak Mihovilović, A., Matković Stanković, A., Lazarević, B., Goreta Ban, S. et al. (2023). Garlic ecotypes utilise different morphological, physiological and biochemical mechanisms to cope with drought stress. Plants, 12(9), 1824. https://doi.org/10.3390/plants12091824 [Google Scholar] [PubMed] [CrossRef]
10. Abd-Elhady, M., Eldardiry, E. I. (2016). Maximize crop water productivity of garlic by modified fertilizer management under drip irrigation. International Journal of ChemTech Research, 9, 144–150. [Google Scholar]
11. El-Zohiri, S. S. M., Farag, A. A. (2021). Reduce the adverse impact of high temperature on the green garlic production for export. Fayoum Journal of Agricultural Research and Development, 31, 17–35. [Google Scholar]
12. Ji, H. S., Bang, S. G., Ahn, M. A., Kim, G., Kim, E. et al. (2021). Molecular cloning and functional characterization of heat stress-responsive superoxide dismutases in garlic (Allium sativum L.). Antioxidants, 10(5), 815. https://doi.org/10.3390/antiox10050815 [Google Scholar] [PubMed] [CrossRef]
13. Eom, S. H., Baek, S. A., Kim, J. K., Hyun, T. K. (2018). Transcriptome analysis in Chinese cabbage (Brassica rapa ssp. pekinensis) provides the role of glucosinolate metabolism in response to drought stress. Molecules, 23(5), 1186. https://doi.org/10.3390/molecules23051186 [Google Scholar] [PubMed] [CrossRef]
14. Medeiros, D. B., da Silva, E. C., Santos, H. R. B., Pacheco, C. M., Musser, R. S. et al. (2012). Physiological and biochemical responses to drought stress in Barbados cherry. Brazilian Journal of Plant Physiology, 24(3), 181–192. https://doi.org/10.1590/S1677-04202012000300005 [Google Scholar] [CrossRef]
15. Sun, X., Zhu, S., Li, N., Cheng, Y., Zhao, J. et al. (2020). A chromosome-level genome assembly of garlic (Allium sativum) provides insights into genome evolution and allicin biosynthesis. Molecular Plant, 13(9), 1328–1339. https://doi.org/10.1016/j.molp.2020.07.019 [Google Scholar] [PubMed] [CrossRef]
16. Kim, D., Langmead, B., Salzberg, S. L. (2015). HISAT: A fast spliced aligner with low memory requirements. Nature Methods, 12, 357–360. [Google Scholar] [PubMed]
17. Eom, S. H., Ahn, M. A., Kim, E., Lee, H. J., Lee, J. H. et al. (2022). Plant response to cold stress: Cold stress changes antioxidant metabolism in heading type kimchi cabbage (Brassica rapa L. ssp. Pekinensis). Antioxidants, 11(4), 700. https://doi.org/10.3390/antiox11040700 [Google Scholar] [PubMed] [CrossRef]
18. Füzy, A., Kovács, R., Cseresnyés, I., Parádi, I., Szili-Kovács, T. et al. (2019). Selection of plant physiological parameters to detect stress effects in pot experiments using principal component analysis. Acta Physiologiae Plantarum, 41(5), 56. https://doi.org/10.1007/s11738-019-2842-9 [Google Scholar] [CrossRef]
19. Laxa, M., Liebthal, M., Telman, W., Chibani, K., Dietz, K. J. (2019). The role of the plant antioxidant system in drought tolerance. Antioxidants, 8(4), 94. https://doi.org/10.3390/antiox8040094 [Google Scholar] [PubMed] [CrossRef]
20. Song, Y., Feng, L., Alyafei, M. A. M., Jaleel, A., Ren, M. (2021). Function of chloroplasts in plant stress responses. International Journal of Molecular Sciences, 22(24), 13464. https://doi.org/10.3390/ijms222413464 [Google Scholar] [PubMed] [CrossRef]
21. Bianchetti, G., Clouet, V., Legeai, F., Baron, C., Gazengel, K. et al. (2021). RNA sequencing data for responses to drought stress and/or clubroot infection in developing seeds of Brassica napus. Data in Brief, 38, 107392. https://doi.org/10.1016/j.dib.2021.107392 [Google Scholar] [PubMed] [CrossRef]
22. Paul, S., Duhan, J. S., Jaiswal, S., Angadi, U. B., Sharma, R. et al. (2022). RNA-Seq analysis of developing grains of wheat to intrigue into the complex molecular mechanism of the heat stress response. Frontiers in Plant Science, 13, 904392. https://doi.org/10.3389/fpls.2022.904392 [Google Scholar] [PubMed] [CrossRef]
23. Singh, V., Gupta, K., Singh, S., Jain, M., Garg, R. (2023). Unravelling the molecular mechanism underlying drought stress response in chickpea via integrated multi-omics analysis. Frontiers in Plant Science, 14, 1156606. https://doi.org/10.3389/fpls.2023.1156606 [Google Scholar] [PubMed] [CrossRef]
24. Chen, L., Yun, M., Cao, Z., Liang, Z., Liu, W. et al. (2021). Phenotypic characteristics and transcriptome of cucumber male flower development under heat stress. Frontiers in Plant Science, 12, 758976. https://doi.org/10.3389/fpls.2021.758976 [Google Scholar] [PubMed] [CrossRef]
25. Du, J., Li, M., Yuan, Z., Guo, M., Song, J. et al. (2016). A decision analysis model for KEGG pathway analysis. BMC Bioinformatics, 17, 407. https://doi.org/10.1186/s12859-016-1285-1 [Google Scholar] [PubMed] [CrossRef]
26. Kulik, A., Wawer, I., Krzywińska, E., Bucholc, M., Dobrowolska, G. (2011). SnRK2 protein kinases-key regulators of plant response to abiotic stresses. OMICS, 15(12), 859–872. https://doi.org/10.1089/omi.2011.0091 [Google Scholar] [PubMed] [CrossRef]
27. Reyes-Impellizzeri, S., Moreno, A. A. (2021). The endoplasmic reticulum role in the plant response to abiotic stress. Frontiers in Plant Science, 12, 755447. https://doi.org/10.3389/fpls.2021.755447 [Google Scholar] [PubMed] [CrossRef]
28. Strasser, R. (2018). Protein quality control in the endoplasmic reticulum of plants. Annual Review of Plant Biology, 69(1), 147–172. https://doi.org/10.1146/annurev-arplant-042817-040331 [Google Scholar] [PubMed] [CrossRef]
29. Al-Yasi, H., Attia, H., Alamer, K., Hassan, F., Ali, E. et al. (2020). Impact of drought on growth, photosynthesis, osmotic adjustment, and cell wall elasticity in Damask rose. Plant Physiology and Biochemistry, 150(1), 133–139. https://doi.org/10.1016/j.plaphy.2020.02.038 [Google Scholar] [PubMed] [CrossRef]
30. Sun, J., Tian, Z., Li, X., Li, S., Li, Z. et al. (2022). Systematic analysis of the pectin methylesterase gene family in Nicotiana tabacum and reveal their multiple roles in plant development and abiotic stresses. Frontiers in Plant Science, 13, 998841. https://doi.org/10.3389/fpls.2022.998841 [Google Scholar] [PubMed] [CrossRef]
31. Yang, W., Ruan, M., Xiang, M., Deng, A., Du, J. et al. (2020). Overexpression of a pectin methylesterase gene PtoPME35 from Populus tomentosa influences stomatal function and drought tolerance in Arabidopsis thaliana. Biochemical and Biophysical Research Communications, 523(2), 416–422. https://doi.org/10.1016/j.bbrc.2019.12.073 [Google Scholar] [PubMed] [CrossRef]
32. Cheng, M., Meng, F., Qi, H., Mo, F., Wang, P. et al. (2022). Escaping drought: The pectin methylesterase inhibitor gene Slpmei27 can significantly change drought resistance in tomato. Plant Physiology and Biochemistry, 192(1), 207–217. https://doi.org/10.1016/j.plaphy.2022.10.008 [Google Scholar] [PubMed] [CrossRef]
33. von Koskull-Döring, P., Scharf, K. D., Nover, L. (2007). The diversity of plant heat stress transcription factors. Trends in Plant Science, 12(10), 452–457. https://doi.org/10.1016/j.tplants.2007.08.014 [Google Scholar] [PubMed] [CrossRef]
34. Fragkostefanakis, S., Röth, S., Schleiff, E., Scharf, K. D. (2015). Prospects of engineering thermotolerance in crops through modulation of heat stress transcription factor and heat shock protein networks. Plant, Cell & Environment, 38(9), 1881–1895. https://doi.org/10.1111/pce.12396 [Google Scholar] [PubMed] [CrossRef]
35. Wruck, F., Avellaneda, M. J., Koers, E. J., Minde, D. P., Mayer, M. P. et al. (2018). Protein folding mediated by trigger factor and Hsp70: New insights from single-molecule approaches. Journal of Molecular Biology, 430(4), 438–449. https://doi.org/10.1016/j.jmb.2017.09.004 [Google Scholar] [PubMed] [CrossRef]
36. Guo, M., Liu, J. H., Ma, X. (2016). Genome-wide analysis of the Hsp70 family genes in pepper (Capsicum annuum L.) and functional identification of CaHsp70-2 involvement in heat stress. Plant Science, 252, 246–256. https://doi.org/10.1016/j.plantsci.2016.07.001 [Google Scholar] [PubMed] [CrossRef]
37. Xu, W., Cai, S. Y., Zhang, Y. (2016). Melatonin enhances thermotolerance by promoting cellular protein protection in tomato plants. Journal of Pineal Research, 61(4), 457–469. https://doi.org/10.1111/jpi.12359 [Google Scholar] [PubMed] [CrossRef]
38. Chen, Q. H., Zhao, X. Q., Lei, D. K. (2017). Hydrogen-rich water pretreatment alters photosynthetic gas exchange, chlorophyll fluorescence, and antioxidant activities in heat-stressed cucumber leaves. Plant Growth Regulation, 83(1), 69–82. https://doi.org/10.1007/s10725-017-0284-1 [Google Scholar] [CrossRef]
39. Zhao, D., Xia, X., Su, J., Wei, M., Wu, Y. et al. (2019). Overexpression of herbaceous peony HSP70 confers high temperature tolerance. BMC Genomics, 20(1), 70. https://doi.org/10.1186/s12864-019-5448-0 [Google Scholar] [PubMed] [CrossRef]
Supplementary Materials
Figure S1: The expression patterns of the selected garlic pectin esterases were determined using qRT-PCR. Transcript levels of the selected genes were normalized to those of garlic actin, and were expressed relative to the values in the control. Level of expression is represented log2 ratio
Cite This Article
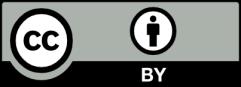